- 1State Key Laboratory Breeding Base of Green Pesticide and Agricultural Bioengineering, Key Laboratory of Green Pesticide and Agricultural Bioengineering, Ministry of Education, State-Local Joint Laboratory for Comprehensive Utilization of Biomass, Center for Research and Development of Fine Chemicals, Guizhou University, Guiyang, China
- 2Laboratory of Inorganic Materials and Catalysis, Schuit Institute of Catalysis, Department of Chemical Engineering and Chemistry, Eindhoven University of Technology, Eindhoven, Netherlands
Cellulose is the most abundant source of biomass, which can be converted into monosaccharide or other chemical platform molecules for the sustainable production of chemicals and fuels. Acid catalysts can promote hydrolytic degradation of cellulose into valuable platform molecules, which is of great significance in the development of chemicals and biofuels. However, there are still some shortcomings and limitations of the catalysts for the hydrolytic degradation of cellulosic biomass. Heteropoly acid (HPA), as a green catalyst, seems to be more conducive to the degradation of cellulosic biomass due to its extreme acidity. HPAs can be designed in homogeneous and heterogeneous systems. Moreover, they can be easily separated from the products in both systems by a simple extraction process. According to the unique properties of HPAs (e.g., good solubility, high thermal stability, and strong acidity), using heteropoly acid-based catalysts to depolymerize and convert cellulose into value-added chemicals and biofuels has become one of the most remarkable processes in chemistry for sustainability. In this review, the characteristics, advantages, and applications of HPAs in different categories for cellulose degradation, especially hydrolysis hydrolytic degradation, are summarized. Moreover, the mechanisms of HPAs catalysts in the effective degradation of cellulosic biomass are discussed. This review provides more avenues for the development of renewed and robust HPAs for cellulose degradation in the future.
Introduction
With the continuous exploitation of human beings, the amount of fossil energy that is the most main energy consumed in the world is decreasing gradually. Therefore, developing a sustainable renewable energy source that can provide valuable chemicals and biofuels is of great importance (Zhang et al., 2015). To furnish the desired bioproducts, some resources are needed which can provide us with basic elements such as C, H, and O in luxuriant amounts (Dhepe and Fukuoka, 2008). Compared with other energy sources (e.g., coal, oil, and natural gas), biomass energy has incomparable advantages as a renewable energy source with little pollution. Moreover, biomass has become the focus of development because of its universality, abundance, and low pollution. In some biorefinery typically using biomass instead of oil, biomass can be converted into energy and relevant bioproducts through multifarious platforms (Chang et al., 2013; Hu et al., 2015; Li et al., 2016; Liu et al., 2016). Especially, biorefinery combines the key technologies of transforming biological raw materials into industrial intermediates and final products (Scheme 1; Kamm and Kamm, 2004; Dhepe and Fukuoka, 2008; Li et al., 2015; Liu and Zhang, 2015; Zhang et al., 2018). Therefore, the utilization of biomass is a wonderful choice to develop sustainable and renewable energy.
As the most abundant source of biomass and one of the most widely distributed and abundant polysaccharides in nature, cellulose provides more possibilities for sustainable chemicals and fuels in the future (Huber et al., 2006; Climent et al., 2014; Sheldon, 2014; To et al., 2015). Hence, the conversion of cellulose could become a significant part of biomass utilization, which plays an important role in the development of chemicals and fuels in the future. However, cellulose is a homopolymer of D-glucose made up of β-1,4 glycosidic bonds (Scheme 2A). Its intramolecular and intermolecular hydrogen bonds are formed (Scheme 2B), resulting in its water insolubility (Geboers et al., 2011; Wang et al., 2012; Harada et al., 2014; Yabushita et al., 2014). Therefore, the processes of cellulose conversion are complicated because this biopolymer is insoluble in water, and most organic solvents (Klemm et al., 2005; Albert et al., 2014).
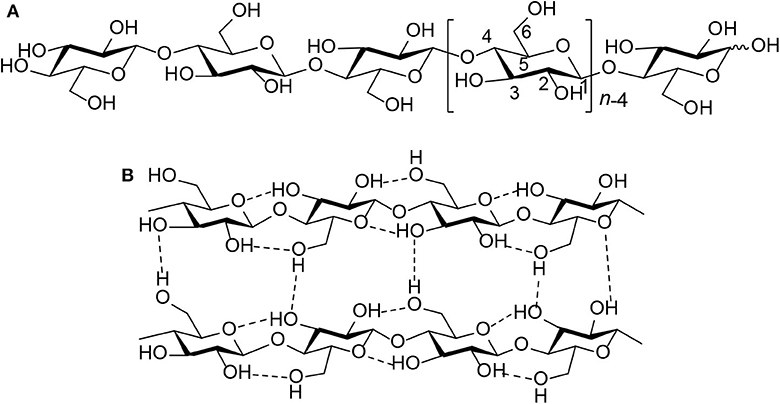
Scheme 2. (A) Molecular structure of cellulose. (B) Intermolecular and intramolecular hydrogen bond interactions in cellulose (n = DP, degree of polymerization).
Many homogeneous and heterogeneous acid catalysts were found to be capable of converting cellulose into glucose, a monosaccharide which is an important component for synthesizing different kinds of chemicals and fuels (Scheme 3), such as furans (Li et al., 2014), ethanol (Chheda et al., 2007), polyhydric alcohol (Geboers et al., 2010; de Op Beeck et al., 2013; Xie et al., 2014; He et al., 2018), furfural (Yu et al., 2009b; Feng et al., 2011; Li et al., 2013; Pan et al., 2013), organic acid (Yan et al., 2014; Zhang et al., 2014; Ren et al., 2015), and so on. Several excellent review literature on the conversion of cellulose have been reported, with focus on the discussion of different solid acid catalysts (e.g., Amberlyst-15, PCPs–SO3H, BC–SO3H, CMK-3-SO3H, Zn–Ca–Fe, CsH2PW12O40, Ru/CMK-3, Fe3O4-SBA–SO3H, CaFe2O4, H3PW12O40, H5BW12O40, H5AlW12O40, H5GaW12O40, and H6CoW12O40) for hydrolysis of cellulose (Table 1; Kobayashi et al., 2010; Pang et al., 2010; Rinaldi et al., 2010; Tian et al., 2010, 2011; Wu et al., 2010; Akiyama et al., 2011; Komanoya et al., 2011; Lai et al., 2011; Ogasawara et al., 2011; Zhang et al., 2011).
Compared with other solid acids, HPA catalysts are more favorable for hydrolysis of cellulose, considering that they have some unique properties such as stronger acidity compared to other mineral acids (e.g., H2SO4, HCl), high redox properties, good thermal stability, easy separation, good reusability, fewer side products, less waste generation, non-toxicity, and easy handling, as illustrated in Figure 1 (Kozhevnikov, 1987, 1998; Mizuno and Misono, 1994; Kaur and Kozhevnikov, 2002; Yu et al., 2009a; Yang et al., 2011; Deng et al., 2012; Reddy et al., 2012; de Op Beeck et al., 2013). Furthermore, HPA catalysts can be used in both homogeneous and heterogeneous systems. The most significant advantage of the heterogeneous system is that the HPA catalysts can be easily separated from the reaction products, thus improving the catalyst reusability. The most common HPAs are Keggin-type HPAs, which have the anions of [XM12O40]n−, where X is a heteroatom (e.g., P, Si) and M is a metal ion (e.g., W6+, Mo6+). Among them, H3PW12O40 is the most common Keggin-type HPA. Moreover, H3PW12O40 with strong acidity is highly soluble in water and can completely dissociate protons, which facilitates contact with the heterogeneous substrates, thus endowing enhanced reaction rates (Tian et al., 2010). Besides, the basic structural units of Keggin-type HPAs are oxygen-containing tetrahedron and octahedron anions in good symmetry and low charge density (Figure 2).
Given the unique features and rapid development of HPAs-based catalysts in the preliminary degradation of cellulosic biomass with high efficiency, this review mainly reports the design and structural characterization of different types of HPAs employed for hydrolytic depolymerization of cellulosic biomass. In addition, the properties and preparation methods of HPAs as well as their advantages in the hydrolysis of cellulose are discussed. Also, possible research trends of HPAs in the future are forecasted.
HPAs-Based Catalysts
HPA Properties and Classification
HPA catalysts have attracted people's attention, and are known as effective, environmentally friendly and economically viable acid catalysts (Kozhevnikov, 1998), which can be used in either homogeneous or heterogeneous state (Izumi et al., 1983; Nowinska et al., 1996; Song and Lee, 2004; Bennardi et al., 2010; Zhao et al., 2013). Compared with a homogeneous system, the heterogeneous system has the advantage that the catalyst is easy to separate from the reaction products. Due to these special features, HPA catalysts are more beneficial to the degradation of cellulosic biomass than other catalysts.
HPA catalysts can be classified into the following categories: (1) Keggin-type HPAs, the most common HAPs with the anions of [XM12O40]n−, where X is a heteroatom (e.g., P, Si) and M is a metal ion (e.g., W6+, Mo6+). (2) Substituted HPAs, the H+ ions on conventional HPAs are substituted by monovalent cations, such as Cs+ ions. (3) Supported HPAs, the heteropoly compounds are supported on suitable supporters, such as silica. (4) Assembled HPAs, the new catalysts with higher activity are synthesized by self-assembly of HPAs with other substances, such as ionic liquids. (5) Other types, new types of heterogeneous catalysts formed by an effective combination of HPAs with other substances.
Catalyst Preparation Methods
Up to the present, many patents and literature on the preparation of HPAs were published, including e.g., traditional acidification method incorporated with an ether extraction, ion exchange method, impregnation method, and sol-gel method (Bechtold and Square, 1950; Laferty and John, 1966; Chiola and Lawrence, 1969; Chiola et al., 1969; Izumi et al., 1995; Vázquez et al., 2000; Mrowiec-Białoń et al., 2002; Cardoso et al., 2004; Yang et al., 2005; Ahmed et al., 2011). The traditional synthesis method of conventional HPAs is an acidification process coupled with an ether extraction, which is commonly used for the synthesis of phosphotungstate HPA catalysts. In this method, the HPA is prepared by mixing heteroatom oxygen-containing acid and ligand oxide in a certain proportion, heating, refluxing, and then acidifying to obtain HPA. The HPA is dissolved in ether, and the solid HPA is obtained after ether volatilization (Chiola and Lawrence, 1969; Chiola et al., 1969). However, the acidification procedure coupled with an ether extraction has the disadvantages of low safety due to the use of toxic substances and low yield of some HPAs. In connection with this, the ion-exchange method has been developed, which first obtains the corresponding ammonium salt, and then obtains HPA through hydrogen ion-exchange resin. This method avoids the use of ether and improves process safety, but the production cycle is relatively long (Bechtold and Square, 1950; Laferty and John, 1966). For some supported HPAs, the impregnation method is commonly used. The method is to add the carrier into the solution of HPA with a certain concentration, stir for a long time at a certain temperature, stand still, and then remove the excess solvent to obtain the catalyst. The method is simple and safe, but there are some problems such as the loss of catalyst activity and limited recycling ability. Therefore, the support should be screened and optimized (Vázquez et al., 2000; Cardoso et al., 2004; Liu et al., 2004). The sol-gel method is to add raw materials to the HPA colloid and obtain the HPA catalyst through a series of specific processes, such as drying and heat treatment. This method can be used to prepare catalysts at a low temperature or mild condition, which is widely used in manufacturing nanomaterials catalysts. Likewise, this method has the disadvantages of low security and long cycle (Izumi et al., 1995; Mrowiec-Białoń et al., 2002; Yang et al., 2005). Some examples of catalysts for the preparation of HPAs are as follows:
1. Keggin-type Mo-V-P heteropoly acid catalysts (Scheme 4; Zhizhina and Odyakov, 2008; Odyakov and Zhizhina, 2009): Firstly, V2O5 was dissolved in cold diluted H2O2 solution to form peroxy complexes, which was gradually decomposed with oxygen to give H6O10V28. Then, by adding excess H3PO4, H6O10V28 acid produced was immediately stabilized to give H9PV14O42, following by addition into the boiling suspension of MoO3 and H3PO4 to form the catalyst.
2. Substituted heteropoly tungstic acid catalyst CsxH3−xPW12O40 (x = 1–3): It is usually prepared by titration method (Okuhara et al., 1992, 1996, 2000; Tian et al., 2011). At room temperature, dropping 0.10 mol dm−3 Cs2CO3 aqueous solution into 0.8 mol dm−3 of H3PW12O40 aqueous solution at a constant rate was stirred continuously. Then the resulting solution was aged overnight at room temperature and heated at 50°C to obtain the catalyst.
3. The synthesis of catalyst (HOCH2CH2N(CH3)3)x H3−xPW12O40 (abbreviated as ChxH3−xPW12O40) is shown in Scheme 5 (Duan et al., 2013; Zhang et al., 2016): choline chloride (ChCl, HOCH2CH2N(CH3)3Cl) (3.34 mmol) was added to H3PW12O40 (3.34 mmol) solution and the white precipitate was formed after stirring in 20 mL distilled water for 8 h at room temperature. Then the white precipitate was washed with distilled water, followed by recrystallization twice with CH3CN, drying at 60°C, and finally getting the white product ChH2PW12O40 (HOCH2CH2N(CH3)3H2PW12O40).
4. The self-assembled HPA ionic liquid catalyst [C4H6N2(CH2)3SO3H]3PW12O40 (Scheme 6; Leng et al., 2010): 0.11 mol methyl imidazole and 0.10 mol 1,3-propane sulfone were dissolved in 20 mL toluene under nitrogen and stirred for 24 h at 50°C. The resulting white precipitate is filtered and washed three times with ether and then dried in a vacuum. Then it was added to the aqueous solution of H3PW12O40 (0.02 mol) and stirred for 24 h at room temperature. Then water was removed in vacuum to obtain solid products, washed with water and ethanol, and dried at 100°C for 3 h.
Hydrolytic Depolymerization of Biomass With HPAS-Based Catalysts
Keggin-Type HPAs
Keggin-type HPAs are the most common HPA catalysts, which have great application prospects for biomass degradation. The potential of HPAs for catalyzing the hydrolysis of cellulose to glucose has been explored.
Cellulose Conversion to Glucose
Tian et al. developed the heteropoly acid H3PW12O40 for selective conversion of cellulose (Scheme 7). The heteropoly acid catalyst can promote cellulose hydrolysis, achieving the glucose selectivity and yield of 92.3 and 50.5%, respectively at 180°C for 2 h with 0.10 g cellulose amount. The authors also studied and optimized reaction parameters influencing the hydrolysis of cellulose and determined the most optimizing reaction conditions. Compared with HCl, H3PW12O40 had greater catalytic activity and glucose selectivity under identical reaction conditions with the same acid concentration (Table 2: 0.2 mmol HCl is equivalent to 0.06 mmol H3PW12O40, and 0.06 mmol H3PW12O40 had higher catalytic activity). After testing the catalytic activity of H3PW12O40 in six consecutive reaction cycles, the total loss of H3PW12O40 was 8.8% of its initial loss (Figure 3; Tian et al., 2010). It was found that the acid catalyst H3PW12O40 was generally stable and could be recycled by extraction using diethyl ether. However, there was still a little loss of catalyst activity, which led to a slight decrease in the yield of TRS and glucose.
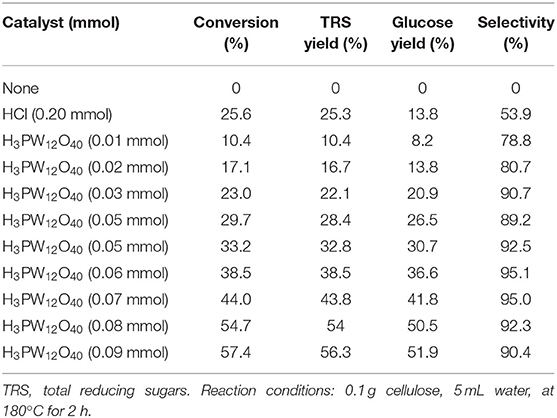
Table 2. Hydrolysis of cellulose with different acid catalysts (Tian et al., 2010).
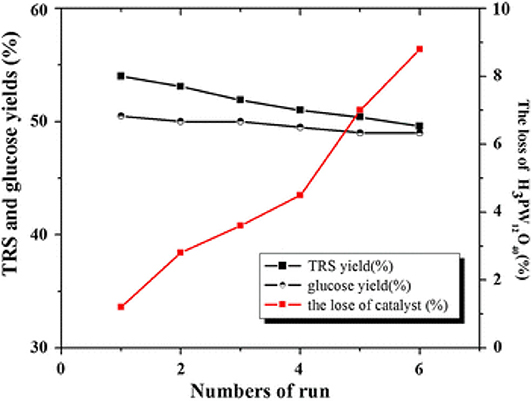
Figure 3. The recyclability of H3PW12O40 in six consecutive reaction cycles. Reaction conditions: 0.1 g cellulose, 0.08 mmol catalyst, 5 mL water at 180°C for 2 h. Reproduced with permission from Tian et al. (2010).
In 2009, Shimizu et al. studied the effects of Brønsted and Lewis acidities on the activity and selectivity of HPA catalysts for hydrolysis of cellulose (Furukawa et al., 2009). The authors also reported that HPAs (i.e., H3PW12O40 and H4SiW12O40) could catalyze the hydrolysis of cellulose with high selectivity to glucose. It was concluded that for Brønsted acid catalysts, the activity increases with the decrease in the deprotonation enthalpies (DPE, for the description of the acid strength of HPAs) (Macht et al., 2008; Shimizu and Satsuma, 2011), indicating that stronger Brønsted acidity is more beneficial to the hydrolysis of cellulose (The effect of DPE on catalysts activity shown in Figure 4). In other words, stronger Brønsted acid is more beneficial to the hydrolysis of β-1,4 glucosidic bonds of cellulose. More interestingly, the obtained catalyst rates are higher for moderate Lewis acidity, while the catalysts with moderate Lewis acidity have higher TOFs for glucose production than H3PW12O40 (Figure 5). Therefore, the Brønsted and Lewis acidities of HPA catalysts can influence the hydrolysis of cellulose. Moreover, with the increase of the catalyst dosage, the yield of glucose increased, which is due to the increase in the number of catalytic active sites. As shown in Figure 4, the lower DPE corresponds to stronger acidity of the catalysts, which is responsible for higher catalytic activity, with the TRS yield in the order of H4SiW12O40 > H3PW12O40 > HClO4 > H2SO4 > H3PO4.
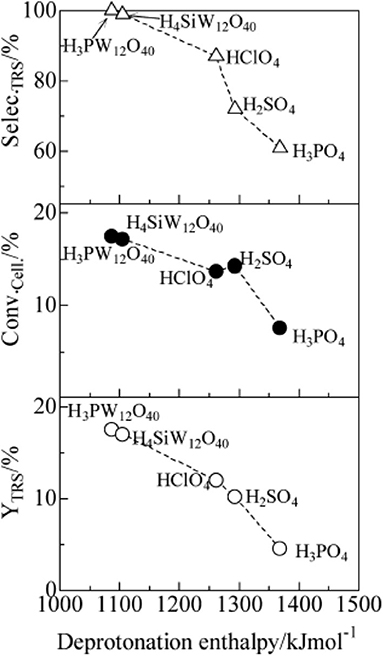
Figure 4. The effect of deprotonation enthalpy (DPE) of acid catalysts on catalysts activity (∘, TRS yield; •, cellulose conversion; Δ, TRS selectivity of cellulose hydrolysis). Reaction conditions: 0.58 mmol cellulose, 6 mL H2O, 6 mol catalyst (Mn/3PW12O40) at 150°C for 2 h. Reproduced with permission from Macht et al. (2008) and Shimizu and Satsuma (2011).
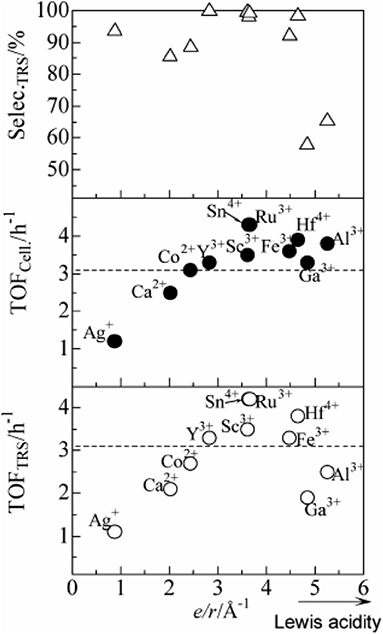
Figure 5. The effect of Lewis acid on the catalytic rate of catalysts (∘, TRS yield; •, cellulose conversion; Δ, TRS selectivity of cellulose hydrolysis). Reaction conditions: 0.58 mmol cellulose, 6 mL H2O, 6 mol catalyst (Mn/3PW12O40) at 150°C for 2 h. Reproduced with permission from Shimizu and Satsuma (2011).
Ogasawara et al. found that the highly negatively charged HPAs (e.g., H5BW12O40, H5AlW12O40, and H5GaW12O40) could efficiently promote the hydrolysis of crystalline cellulose into glucose in concentrated aqueous solutions (Ogasawara et al., 2011). In particular, H5BW12O40 showed a superior yield of glucose (77%) for 48 h in 0.7 mol/L cellulose solution at a lower reaction temperature (60°C), which avoided the formation of undesirable byproducts such as humic substances and dehydration products. And its performance is much better than the commonly utilized mineral acids and HPAs, such as H2SO4, HCl, H3PW12O40, and H4SiW12O40 (Table 3). Procedures for saccharification contains two important points: turning the acidity of catalysts and decreasing the crystallinity of cellulose by breaking intermolecular hydrogen bonding that can be achieved by concentrated H5BW12O40 aqueous solutions (0.70 mol/L). Besides crystalline cellulose, the present system was suitable for the selective transformation of cellobiose and starch into glucose with a good yield of 82 and 85%, respectively (Scheme 8). Also, H5BW12O40 and saccharide can be completely separated from aqueous reaction solutions by extraction using alcoholic solvents due to their different solubility. And the retrieved highly negatively charged HPA H5BW12O40 can be used repeatedly at least ten times without significant performance loss (Figure 6; Ogasawara et al., 2011).
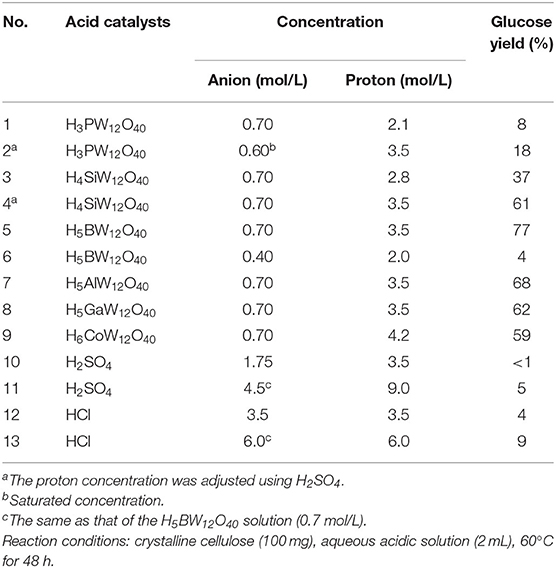
Table 3. Hydrolysis of cellulose using different concentrations of aqueous acidic solutions (Ogasawara et al., 2011).
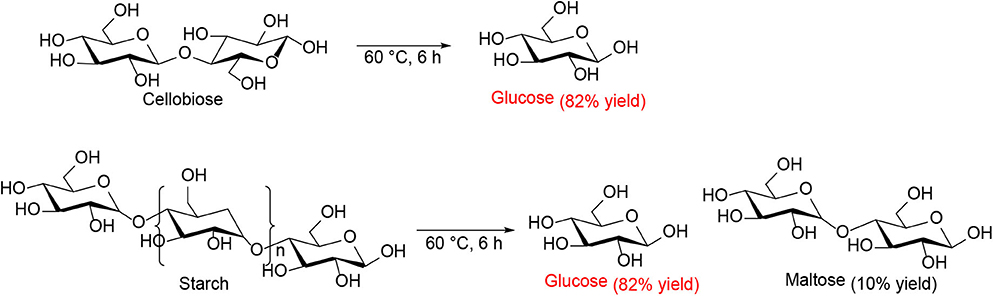
Scheme 8. Transformation of cellobiose and starch in concentrated H5BW12O40 aqueous solutions (0.70 mol/L, 2 mL).
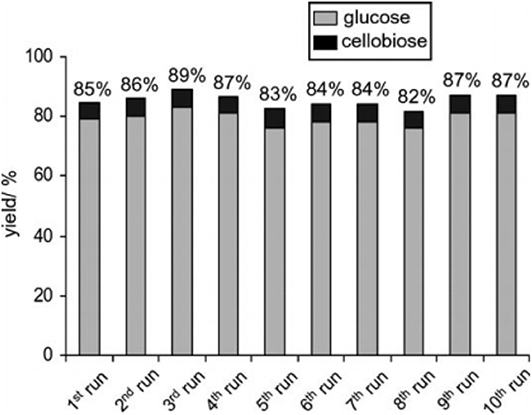
Figure 6. Recyclability of H5BW12O40 for the conversion of pretreated cellulose. Reaction conditions: 100 mg pretreated cellulose (the cellulose was mercerized and then ball-milled), 0.70 mol/L, 2 mL H5BW12O40 solution, 60°C for 24 h. Reproduced with permission from Ogasawara et al. (2011).
For the conversion pathways, the HPAs can initially hydrolyze cellulose into glucose that can be further converted into other substance in the assistance of their bifunctional properties (i.e., acidity and redox property), such as catalytic oxidation of cellulose to formic acid (Wölfel et al., 2011; Albert et al., 2012, 2014; Gromov et al., 2016; Lu et al., 2016), direct conversion of cellulose to glycolic acid (Zhang et al., 2012), and cellulose transformation into methyl glucosides (Scheme 9; Zheng et al., 2018).
Cellulose Conversion to Formic Acid
Conversion of cellulose to formic acid using HPAs as catalysts is an efficient and environmentally friendly catalytic system (Lu et al., 2016). This conversion system involves two stages (Scheme 10): (1) acid-catalyzed the hydrolysis of cellulose into glucose, and (2) oxidation of glucose to formic acid with an oxidizer (C-C bond in glucose is oxidized and cracked by HPA catalysts) (Albert et al., 2014; Gromov et al., 2016).
In 2014, different Keggin-type HPAs H3+n[PVnMo12−nO40] (n = 0–6) were synthesized, and the optimized HPA catalyst system H8[PV5Mo7O40] could selectively oxidize biomass to formic acid (Albert et al., 2014). The whole reaction mechanism was divided into three steps: acid-assisted hydrolysis of the insoluble substrate, oxidative cleavage of C–C bonds in the substrate, and re-oxidation of the in situ reduced HPA catalyst by molecular oxygen. The acid catalyst redox cycle is shown in Scheme 11. Albert et al. also confirmed that the HPA catalysts screened with glucose as substrate had general reactivity, and the higher V-substituted catalysts (n = 2–6) showed better activities than the lower V-substituted catalysts (n = 0–1) (Albert et al., 2014). The comparison of the yields of glucose to formic acid under different HPA catalysts is shown in Table 4. Moreover, when H5PV2Mo10O40 and p-toluenesulfonic acid (TSA) are used as a catalyst and an additive, respectively, the conversion of cellulose was explained as a two-stage process that acid-catalyzed both cellulose hydrolysis and glucose oxidation to formic acid (Wölfel et al., 2011; Albert et al., 2014). In the system, TAS was used for the hydrolysis of cellulose, while H5PV2Mo10O40 was used for the oxidant of glucose. It is worth noting that H8[PV5Mo7O40] catalyst has pronounced activity for the conversion of cellulose to formic acid, but with the disadvantage of low selectivity toward formic acid.
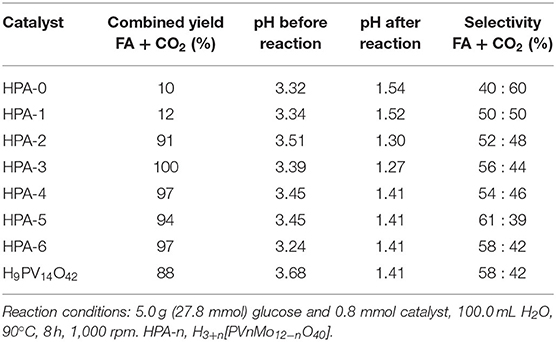
Table 4. The comparison of the yields of glucose to formic acid with different HPA catalysts (Albert et al., 2014).
In 2016, Lu et al. found that a Keggin-type heteropoly acid catalyst H5PV2Mo10O40 with H2SO4 was efficient for catalytic oxidation of cellulose to formic acid with oxygen as an oxidant (Lu et al., 2016). The authors discussed the effects of the pH on the catalyst and reaction pathway by adding H2SO4 with variable amounts (Table 5). It was shown in Figure 7 that when 1.6 wt% H2SO4 was added (equivalent to a significant reduction in pH), the conversion of cellulose increased from 60 to 100%, and the yield of formic acid increased from 28 to 61% in a reaction time of 5 min. It can be concluded that adding H2SO4 to the solution promotes the protonation of H5PV2Mo10O40. Decreasing the pH from 1.87 to 0.56 is beneficial for the catalyst protonation and can increase the oxidation potential. In summary, the H5PV2Mo10O40 + H2SO4 system shows a stronger catalytic impact by reducing pH than the direct use of H5PV2Mo10O40 (Lu et al., 2016).
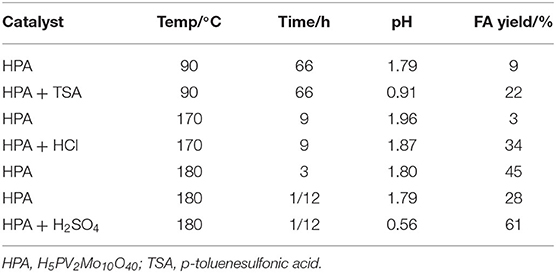
Table 5. Synthesis of formic acid (FA) from cellulose at different pH (Lu et al., 2016).
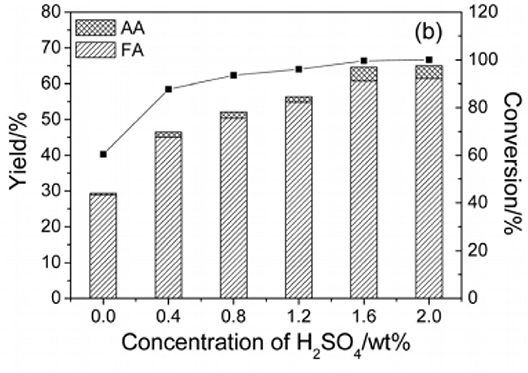
Figure 7. Conversion of cellulose to formic acid (FA) and acetic acid (AA) under different concentration of H2SO4. Reaction conditions: 0.16 g cellulose, 0.10 g catalyst, 6 mL H2O, 3 MPa O2, 180°C for 0.25 h. Reproduced with permission from Lu et al. (2016).
Likewise, Gromov et al. reported the one-pot catalytic process which was applied to hydrolytic oxidation of cellulose to formic acid (Gromov et al., 2016). In their work, Mo-V-P heteropoly acid catalysts were found to be beneficial for hydrolytic oxidation of cellulose to formic acid because of their bifunctional (i.e., oxidizing and acidic) catalytic properties. The oxidizing and acidic catalytic properties correspond to the conversion process of cellulose to formic acid which involves two stages: Step 1: hydrolysis of cellulose to glucose via acidic HPA sites; Step 2: The oxidation of glucose to formic acid taking place at the oxidation sites of Mo-V-P HPA (V5+) (Scheme 12). Therefore, cellulose was hydrolyzed and then oxidized to formic acid using the low-cost Mo-V-P HPA catalysts. Cascade hydrolysis and oxidation of cellulose to formic acid with high yields (65–66 mol%) were achieved by the single-step catalytic process in the presence of Mo-V-P HPA catalysts. In addition, the temperature of 150–160°C and air pressure of 10–20 Mpa (20% O2 and 80% N2) were found to be the optimal reaction conditions.
The Keggin-type (Mo-V-P)-HPAs were used to catalyze the hydrolysis of hemicellulose in a diluted aqueous solution that was reported by Shatalov (2019). HPAs of series H(n+3)[PMo(12−n)VnO40] used as the bifunctional catalysts were studied. For example (Mo-V-P)-HPAs-catalyzed hydrolysis of model xylan, which confirmed the established order of HPAs catalytic activity in hydrolysis reaction (H3PMo12O40 > H6[PMo8.9V3.1O40] > H7[PMo7.9V4.2O40] >> H2SO4). In addition (Mo-V-P)-HPAs, showed remarkable activity in both the acid-catalyzed reactions and the oxidative degradation of lignin. Therefore (Mo-V-P)-HPAs, as the green bifunctional catalysts have the potential to be an alternative to common mineral acids for selective hydrolysis and oxidative degradation of a variety of polysaccharides.
Cellulose Conversion to Other Substances
In the oxygen environment, HPAs can also catalyze cellulose being converted to glycolic acid. In 2019, Zhang et al. reported the conversion of cellulose to glycolic acid by phosphomolybdic acid catalyst (Zhang et al., 2012). In this reaction system, the heteromolybdic acid acted as multifunctional catalysts that first hydrolyzed cellulose to glucose, followed by oxidation to glycolic acid in a water medium, which combines the advantages of homogeneous and heterogeneous catalysts. The proposed reaction pathways for the conversion of cellulose to glycolic acid are shown in Scheme 13. Cellulose was hydrolyzed to glucose, which was converted to glycolaldehyde by the continuous retro-aldol reaction, and then to glyoxal by oxidation. Simultaneously, the isomerization of glucose produced fructose, which was converted to glycolaldehyde and formaldehyde via the retro-aldol reaction, which was then oxidized to glycolic acid and formic acid, respectively. In addition, the authors investigated the reusability of the phosphomolybdic acid catalyst in cellulose conversion (Figure 8). The results indicated that the catalyst exhibited constant catalytic performance. X-ray photoelectron spectroscopy (XPS) showed that the oxidation state of Mo in the heteromolybdic acid catalyst was unchanged after successive reactions (Figure 9). It can be concluded that Mo-containing HPAs can effectively promote the conversion of various kinds of cellulosic biomass materials to glycolic acid.
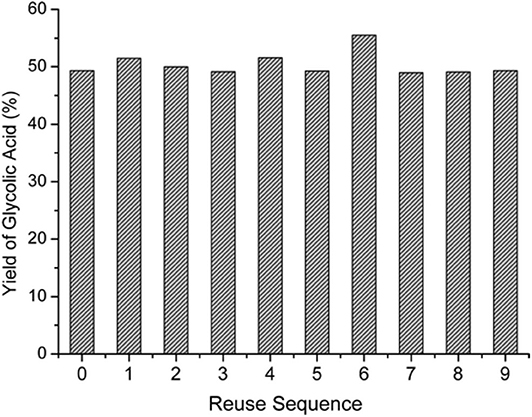
Figure 8. The yield of glycolic acid in the continuous reaction of cellulose conversion with HPM (H3PMo12O40) as the catalyst. Reproduced with permission from Zhang et al. (2012). Reaction conditions: 200 mg cellulose, 0.025 mmol catalyst, 20 mL H2O, 0.6 MPa O2, 180°C for 1 h. Reproduced with permission from Zhang et al. (2012).
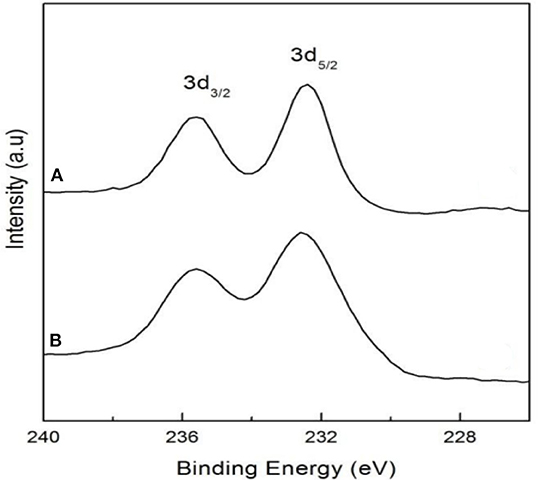
Figure 9. XPS spectra of Mo 3d in the fresh (A) and used (B) HPM (H3PMo12O40) catalyst. Reproduced with permission from Zhang et al. (2012).
The effective alcoholysis of cellulose to monosaccharide is of great significance for fuel production. The H3PW12O40 catalyst can promote cellulose transformation into methyl glucosides after pretreatment of microcrystalline cellulose by the ionic liquid 1-allyl-3-methylimidazolium chloride, as reported by Zheng et al. (2018). In their work, cellulose was firstly obtained by pretreatment with the ionic liquid 1-allyl-3-methylimidazolium chloride, followed by cellulose alcoholysis using H3PW12O40 as the bifunctional catalyst. It was found that cellulose was easier to be saccharified after pretreatment by the ionic liquid. Meanwhile, prolonging the pretreatment time with the increased temperature is beneficial to the succedent alcoholysis reaction. Whereafter, the authors investigated the effects of various parameters on the alcoholysis reaction, including the alcoholizing time, the alcoholizing temperature, and different concentrations of H3PW12O40 catalyst. It was observed that under the optimum conditions (110°C, 60 min), the yield of methyl glucosides could be up to 70.2% with this system. Therefore, using some processes such as pretreatment of cellulose with ionic liquid can make it easier to saccharify, thus better-promoting HPA catalytic conversion of cellulose into the desired products. However, the reusability of the catalyst is not satisfactory. As shown in Figure 10, the activity of the H3PW12O40 catalyst decreased gradually in the repeated experiment, indicating the low catalyst stability.
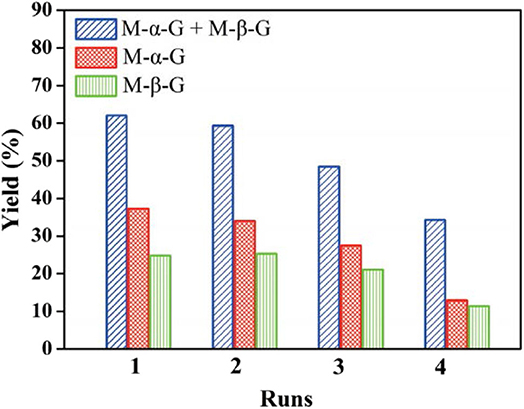
Figure 10. Recyclability of H3PW12O40 in alcoholysis of cellulose to methyl α-D-glucoside (M-α-G) and methyl β-D-glucoside (M-β-G). Reaction conditions: 0.3 g cellulose, 8.7 mmol/L catalyst H3PW12O40, 170°C for 2.75 h. Reproduced with permission from Zheng et al. (2018).
In addition, the HPA is also an important catalyst for the formation of glucose from other saccharides. In 2015, Klein et al. found that HPA can catalyze the hydrolysis of glycogen to glucose (Klein et al., 2015). Through a series of experiments, it can be concluded that glycogen could be completely converted to glucose by using H3PW12O40·nH2O and H4SiW12O40·nH2O as the catalyst, respectively, and the optimized hydrothermal conditions are a mass fraction of catalyst 2.4%, 100°C temperature, and 2 h reaction time. In addition to hydrolysis in an autoclave, the authors also investigated microwave irradiation and sonication heating mode for glucose synthesis (Table 6). The results showed that using H4SiW12O40 as a catalyst, glycogen could be completely converted into glucose by the autoclave method and sonication method without other by-products, so relatively high selectivity toward glucose (>99%) was obtained using these two methods. On the other hand, when glycogen was hydrolyzed by microwave method, glycogen was completely transformed in 0.25 h, but giving by-products like levulinic acid and formic acid, which showed lower selectivity of glucose than the other two methods. More importantly, under microwave irradiation, the formation of glucose was the result of the increase of reaction temperature in the process of microwave irradiation (Klein et al., 2012). In the process of sonochemical irradiation, the acoustic bubble collapsed at a faster speed, causing local high temperatures, thus promoting the hydrolysis of glycogen to glucose (Klein et al., 2012). Therefore, high temperature is the key factor of glycogen hydrolysis, and the HPAs are highly efficient, environmentally friendly, and reusable catalysts that can catalyze the hydrolysis of glycogen to glucose.
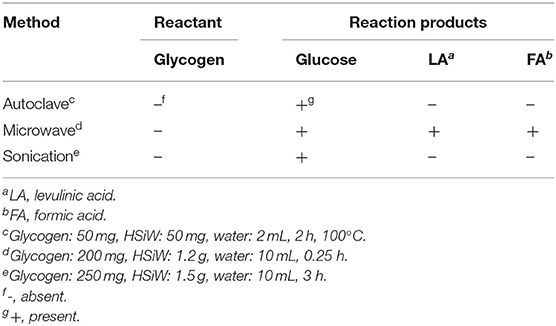
Table 6. Effect of H4SiW12O40 on glycogen hydrolysis using different methods (Klein et al., 2012).
Substituted HPAs
Substituted HPAs are formed when H+ ions on conventional HPSs are substituted by monovalent cations, such as Cs+ ions. One of the typical substitutional HPAs, CsxH3−xPW12O40 has the advantages of super acidity, microporous structure, shape selectivity, and hydrophobicity, which is conducive to the hydrolysis of cellulose (Okuhara, 2002).
Cellulose Conversion to Glucose
In 2010, Tian et al. reported hydrolysis of cellulose over the CsxH3−xPW12O40 (x = 1–3) catalysts, which were active in the hydrolysis of cellulose into glucose. Notably, cellulose can be hydrolyzed to glucose in the presence of CsxH3−xPW12O40 catalysts due to the breaking of β-1,4-glycoside bond (Scheme 14).
After a series of catalyst tests, it was found that Cs1H2PW12O40 showed the best catalytic performance in terms of cellulose conversion (54.0%) and glucose yield (27.2%), while Cs2.2H0.8PW12O40 showed the highest selectivity toward glucose (83.9%). The test results are shown in Figures 11A–C. In the meantime, they also studied the effect of CsxH3−xPW12O40's properties on cellulose hydrolysis. Cs1H2PW12O40 with the strongest protonic acid site was found to have the best catalytic performance for the hydrolysis and transformation of cellulose. However, the catalyst surface area and porous structure have little effect on the conversion of cellulose (Tian et al., 2010). Stronger Brønsted acid is more beneficial to the hydrolysis of β-1,4-glycoside bond in cellulose (Furukawa et al., 2009). It is further proved that Cs1H2PW12O40 with the strongest acidity is more conducive to the degradation of cellulose. When the catalyst and the unreacted cellulose were in the same reaction, the catalyst kept high activity during the hydrolysis of cellulose to TRS and glucose with the yields was 30.1 and 27.2%, respectively. Therefore, the tested acid catalyst is stable and can be reused.
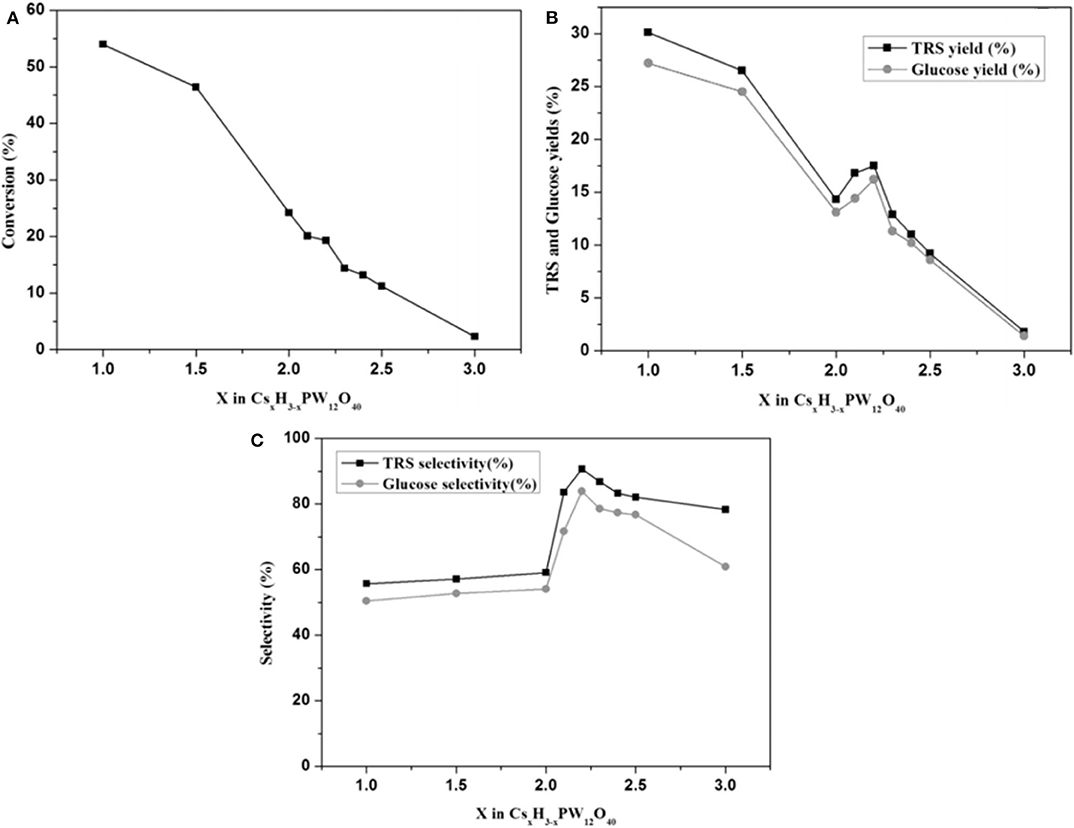
Figure 11. (A) The relationship between cellulose conversion and cesium content in CsxH3−xPW12O40, (B) the relationship between the yield of TRS and glucose and cesium content in CsxH3−xPW12O40, and (C) the relationship between the selectivity of TRS and glucose and cesium content in CsxH3−xPW12O40. Reaction conditions: 0.1 g microcrystalline cellulose, 0.06 mmol CsxH3−−xPW12O40, 5 mL distilled water, at 160°C for 6 h (stirring 30 rpm). Reproduced with permission from (Okuhara, 2002).
Cellulose Conversion to Other Products
In 2011, Geboers et al. studied the hydrotreated cesium salts of HPAs in combination with Ru/C which can be used to degrade cellulose for the synthesis of hexitol (Geboers et al., 2011). The authors discussed the effects of calcination temperature and cesium HPAs hydrotreating conditions on cellulose conversion. The catalytic properties of several cesium HPAs were discussed and compared with conventional HPAs (Table 7). The synthesized cesium salts of HPAs were more active than the natural HPA salts, and their hydrolysis activity was further improved by increasing the calcination and hydrotreating temperature in the synthesis process.
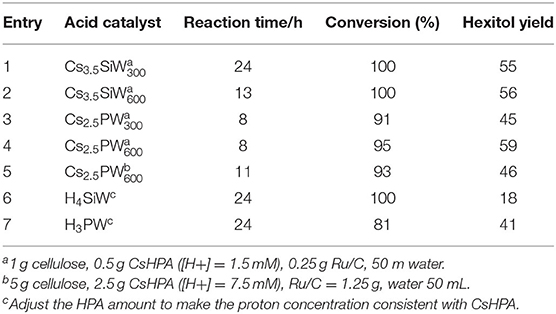
Table 7. Hydrolysis and hydrogenation of cellulose with Ru/C and different HPAs at 190°C and 5 MPa H2 (Geboers et al., 2011).
As compared with their fully protonated counterparts (Cs2.5PW and Ru/C or Cs3.5SiW and Ru/C), the cesium HPAs have higher proton activity due to their higher surface acidity and hydrophobicity. After a hydrotreatment of cesium HPAs catalysts in water at 190°C was performed, the activity and selectivity of the catalysts were improved obviously due to the increase of crystallinity and hydrophobicity of samples. Finally, they found that these cesium HPAs could be completely recovered under certain conditions by a simple recrystallization method (Geboers et al., 2011). In 2011, they had studied the catalysts formed by natural HPAs in combination with Ru/C for cellulose degradation to hexitols (90%). These catalysts also had extraordinary catalytic performance, but they were difficult to recovery and reuse because of the limitation of reaction temperature (Geboers et al., 2010). Therefore, the hydrotreated cesium salts of HPAs in combination with Ru/C are more ideal for cellulose degradation.
In 2014, Zhang et al. synthesized a series of HPA catalysts (HOCH2CH2N(CH3)3)xH3xPW12O40 (HOCH2CH2N(CH3)3 abbreviated as Ch), which could be used as heterogeneous catalysts for degradation of cellulose to 5-hydroxymethylfurfural (HMF) (Zhang et al., 2016). By comparing several different types of acid catalysts (Figure 12), they found that the conversion of cellulose with Brønsted acid in HCl and H3PW12O40 was 41.5 and 89.2%, respectively, while that of ChCl and Ch3PW12O40 without Brønsted acid was 2.0 and 12.2%, respectively. So the strong Brønsted acidity of the catalyst was the necessary condition for cellulose hydrolysis. And (HOCH2CH2N(CH3)2)H2PW12O40 had the highest catalytic activity among the previous catalysts. The yield of HMF can reach 75% when cellulose was catalyzed by (HOCH2CH2N(CH3)2)H2PW12O40 within 8 h at 140°C. Besides, the temperature-responsive property and high stability of the catalyst made the recovery and reuse benefit reach 10 times without obvious activity loss (Figure 13).
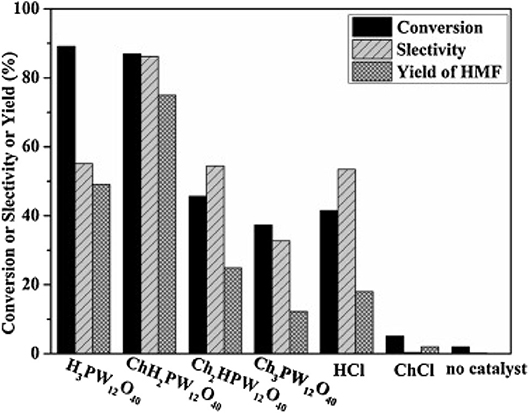
Figure 12. Hydrolysis of cellulose under different catalysts. Reaction conditions: 0.1 g cellulose, 0.11 mmol catalyst, 0.5 ml water, 5 mL methyl isobutyl ketone, 140°C for 8 h. Reproduced with permission from Zhang et al. (2016).
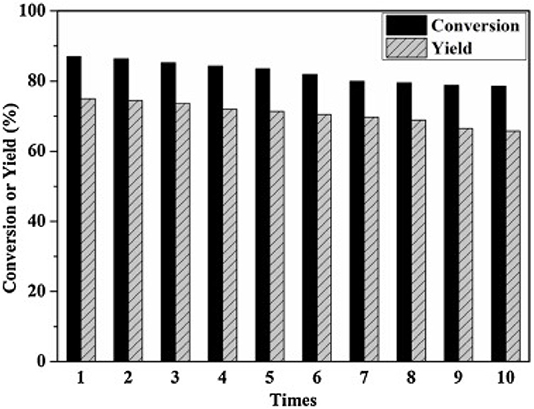
Figure 13. The recycle reaction test of catalyst. Reaction conditions: 0.1 g cellulose, 0.11 mmol ChH2PW12O40, 0.5 mL water, 5 ml methyl isobutyl ketone, 140°C for 8 h. Reproduced with permission from Zhang et al. (2016).
Overall, it can be seen that the substituted HPA catalysts showed high activity in the conversion of cellulose and they were stable and reusable. Therefore, the substituted HPA catalysts have good application prospects in cellulose degradation. However, the substituted HPA catalysts still suffer the disadvantage of low target product yield or selectivity, possibly due to the resistance of mass transfer.
Supported HPAs
So far, more and more attention has been paid to the supported acid catalysts, especially the supported HPAs. The dispersion of these catalysts on the support material increases the specific surface area, thus improving the catalytic activity. Some supported HPAs have been used in the degradation of cellulose. In 1981, Yusuke and Urabe found that activated carbon can encapsulate a certain amount of HPA, thus producing solid acid catalysts, which provided a convenient method for liquid phase etherification and gas-phase selective esterification of alcohols (Yusuke and Urabe, 1981). In 1989, the nuclear magnetic resonance (NMR) technology had been used in the structural research of HPAs and H3PW12O40 supported on SiO2 (Mastikhin et al., 1990).
HPAs are usually used as soluble catalysts in the liquid phase and as supported catalysts in the gas phase. From a practical point of view, it is useful to develop those supported HPA catalysts, which can be applied to a variety of reactions through fixed catalysts without leakage of HPA in the liquid, such as acylation of anisole with acetic anhydride (Bachillerbaeza and Anderson, 2004), the electrophilic substitution of phenols and aldehydes with HPA/MCM-41 (Udayakumar et al., 2007), and dehydration of aldehydes to nitriles catalyzed by silica-supported HPAs (Scheme 15; Parghi et al., 2011), and so on.
In 2007, Guo et al. studied a sol-gel co-condensation technology that can be used to prepare the green supported heteropoly acid catalysts (H3PW12O40-silica), which was an efficient and reusable solid acid catalyst for the catalytic esterification of levulinic acid (LA) with phenol (Guo et al., 2007). The SBA-15 silica-supported H3PW12O40 catalysts exhibited unique surface physicochemical properties, such as high porosity, larger pore size, and larger surface area (Table 8) that is attractive for the study of acid-catalyzed reactions. Figure 14 shows the nitrogen adsorption-desorption isotherms of the H3PW12O40/SBA-15 catalyst, indicating a clear mesoporous structure and relatively narrow pore size distribution. It is shown in Figure 14 that all isotherms are type IV and the results show that the capillary condensation occurs at high relative pressure (P/P0 = 0.45−0.85), indicating that the mesoporous structure is clear and the pore size distribution is relatively good.
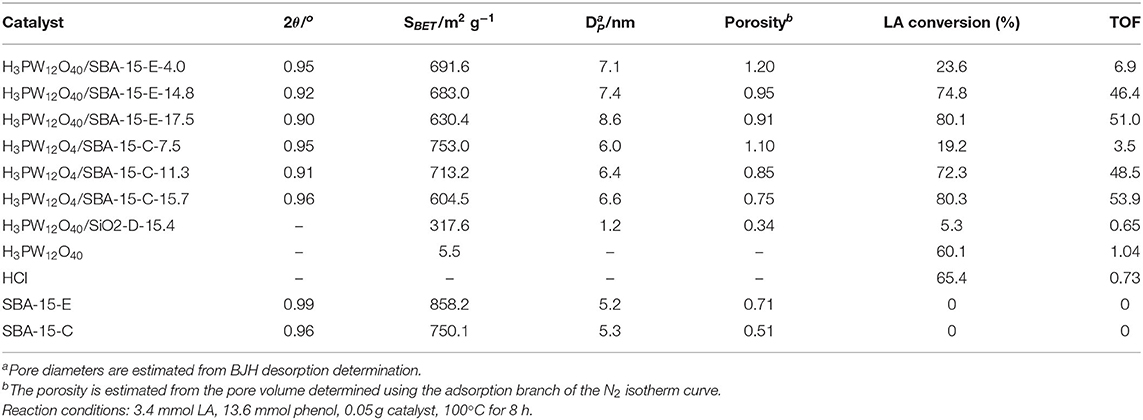
Table 8. Comparison of surface properties and catalytic activities of different catalysts in the esterification of levulinic acid (LA) with phenol (Guo et al., 2007).
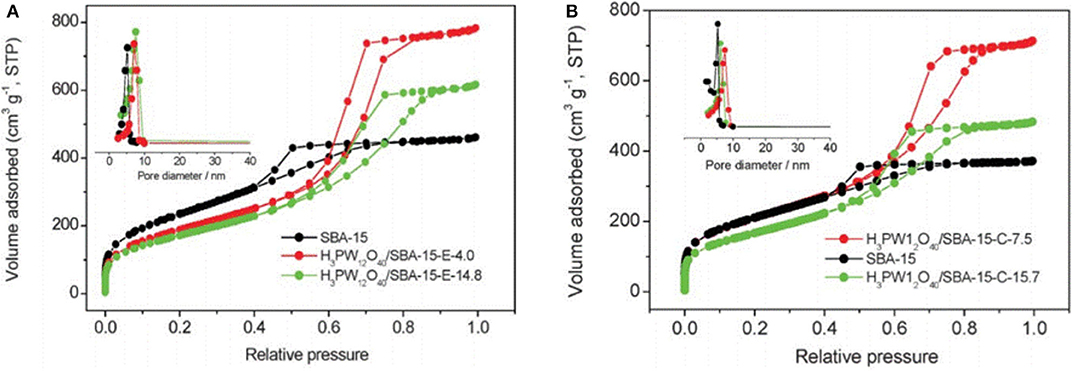
Figure 14. Nitrogen adsorption-desorption isotherms and pore size distribution profiles (inset) of H3PW12O40/SBA-15 materials. (A) Removal of H3PW12O40/SBA-15 by extraction with acidic ethanol, and (B) removal of H3PW12O40/SBA-15 by calcination at 400°C. Reproduced with permission from Guo et al. (2007).
In 2011, silica-supported HPA, a heterogeneous and environmentally benign catalyst was found by Parghi et al. (2011). And it was characterized by many analytical techniques. The effect of catalysts on aldosterone dehydration is shown in Table 9. The results showed that the silica-supported phosphotungstic acid catalyst was the most favorable catalyst for the aldosterone dehydration reaction they studied. The reusability of the catalyst was investigated, and it was found that the catalyst was stable in five consecutive recycles with benzonitrile yield slightly decreasing from 85 to 79%.
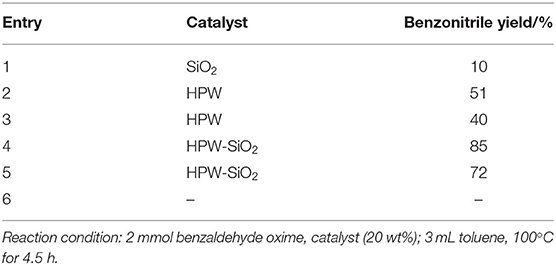
Table 9. Aldosterone dehydration with different catalysts (Parghi et al., 2011).
In 2012, An et al. reported on the conversion of cellulose and cellobiose by polyoxometalate-supported gold nanoparticles (An et al., 2012). The catalysts, CsxH3xPW12O40 supported Au nanoparticles can convert cellulose and cellobiose into gluconic acid that is widely used in the pharmaceutical and food industry. The reaction pathways of cellulose to gluconic acid are shown in Scheme 16. Cellulose was first hydrolyzed to glucose, which was then oxidized to gluconic acid by oxygen. The selectivity of Au/CsxH3xPW12O40 to gluconic acid was significantly higher than that of Au catalysts supported on typical metal oxides, carbon nanotubes, and zeolites (Table 10; An et al., 2012). The possible reasons were the acidity of polyoxometalates and the average particle size of Au nanoparticles. The strong acidity of polyoxometalates not only benefited the conversion of cellobiose but also promoted desorption to improve the selectivity of gluconic acid. And smaller Au nanoparticles expedited the oxidation of glucose to gluconic acid, thus increasing the conversion of cellobiose (Figure 15).
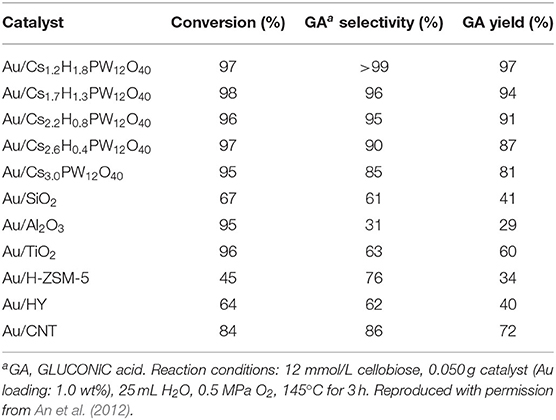
Table 10. Transformation of cellobiose with different supported catalysts (An et al., 2012).
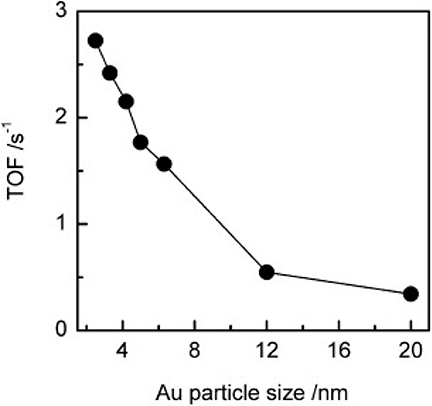
Figure 15. The effect of Au/Cs2.2H0.8PW12O40 catalysts with different average particle sizes of Au on TOFs of glucose oxidation to gluconic acid. Reaction conditions: 24 mmol/L glucose, 25 mL H2O, 0.5 MPa O2, 145°C for 3 h. Reproduced with permission from An et al. (2012).
In 2012, Lanzafame et al. reported that some solid acid catalysts can convert cellulose into glucose, HMF, and other soluble by-products in 190°C aqueous solution for 5 h or <5 h (Lanzafame et al., 2012). They mainly studied supported solid acid catalysts, including sulphated zirconia supported over HPAs, which were found to effectively transform cellulose at a reaction temperature of 190°C (Figure 16). It can be seen that all the catalysts were active in the hydrolysis of cellulose to glucose. However, a significant disadvantage is that the conversion of cellulose with a solid acid catalyst produces more by-products, resulting in low glucose selectivity.
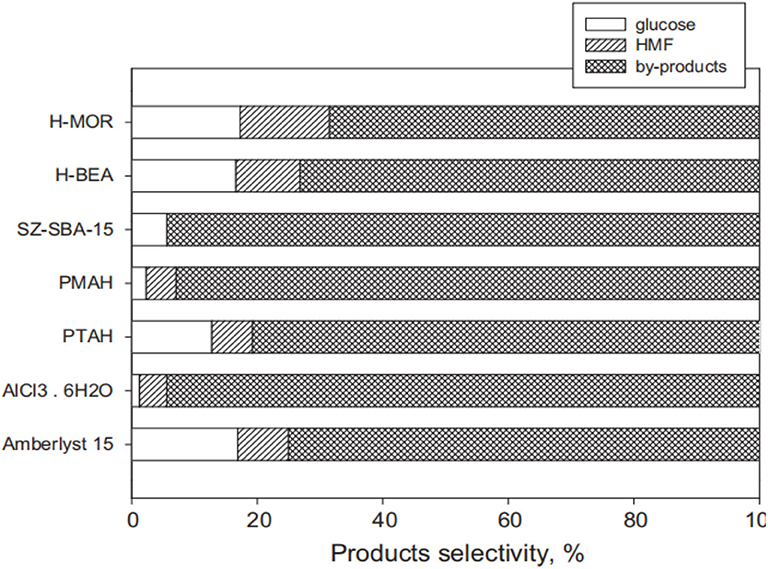
Figure 16. Activity comparison of different solid acid catalysts for cellulose conversion. Reaction conditions: 2 g cellulose, 0.2 g catalyst, 55 mL distilled water, 190°C for 5 h. Reproduced with permission from Lanzafame et al. (2012).
In 2014, Hafizi et al. found a unique heterogeneous silica-supported Preyssler heteropoly acid catalyst (H14NaP5W30O120), which can be used for alkylation of benzene (Hafizi et al., 2014). Preyssler HPA has the advantages of high thermal stability, high hydrolysis stability, regeneration, safety, easy separation, and so on (Bamoharram et al., 2004; Heravi et al., 2007, 2008). It combines with silica to form a supported heteropoly acid catalyst, which is active for the alkylation of benzene. In 2019, Zhang et al. developed a series of highly efficient heterogeneous catalysts with good stability and reusability for the catalytic conversion of cellulose to glucose. A typical Keggin type HPA (H3PW12O40) was immobilized on the surface and pore of carbon foam (CF) to prepare supported HPA catalyst (H3PW12O40/CF) for cellulose conversion to glucose (Zhang et al., 2019). After comparing with different CFs that were prepared from damaged starch and gluten protein (s is the percentage of gluten protein), it was found that CF30 was considered as the best porous support material for fixing the HPW catalyst because of its higher specific surface area (Table 11). In addition, H3PW12O40/CF30 catalyst had good reusability and was easy to separate and recover from the reaction system (Figure 17). It was shown that the supported catalyst could effectively catalyze the conversion of cellulose to glucose, and the supported technology could improve the catalytic performance and high-efficiency reusability of the catalyst.
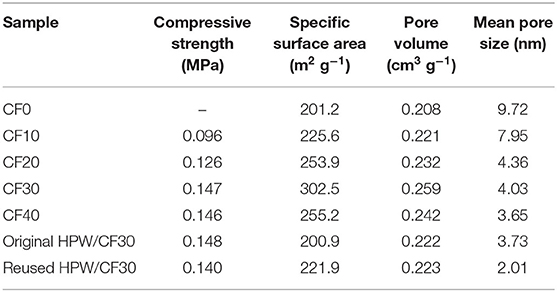
Table 11. Selected properties of different catalysts (Zhang et al., 2019).
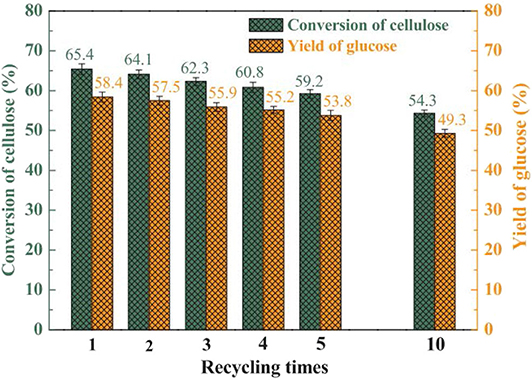
Figure 17. Reusability test of the HPW/CF30 catalyst for cellulose hydrolysis. Reaction conditions: 0.1 g MAMCC (mechanical activation microcrystalline cellulose), 1.0 g HPW/CF30, 10 mL H2O, 170°C for 9 h. Reproduced with permission from Zhang et al. (2019).
Based on the studies of these supported HPA catalysts, it can be seen that the supported HPAs have a higher specific surface area than conventional HPAs. Also, the supported HPAs can be used in the heterogeneous reaction, which is more convenient for product separation and can be reused. It is worth noting that there are still some shortcomings of the catalysts, such as low selectivity of the target product and gradual decline of stability of the catalysts in recycles.
Assembled HPAs
In recent years, people began to explore new efficient catalysts for cellulose conversion. The assembled HPA catalysts have also become one of the research directions. It is noteworthy that the assembled HPA catalysts were studied for the degradation of cellulose in biomass and other valuable catalytic reactions. For example, in 2010, Wee et al. proposed a simple and highly repetitive synthesis method for the assembly of nanomaterial catalyst H3PW12O40/Cu3(BTC)2 (BTC = benzene tricarboxylic acid). Cu3(BTC)2 was encapsulated into Keggin HPA H3PW12O40 for application in catalysis (Wee et al., 2010). And this assembled HPA nanomaterial catalyst can be used in acid-catalyzed esterification (Figure 18).
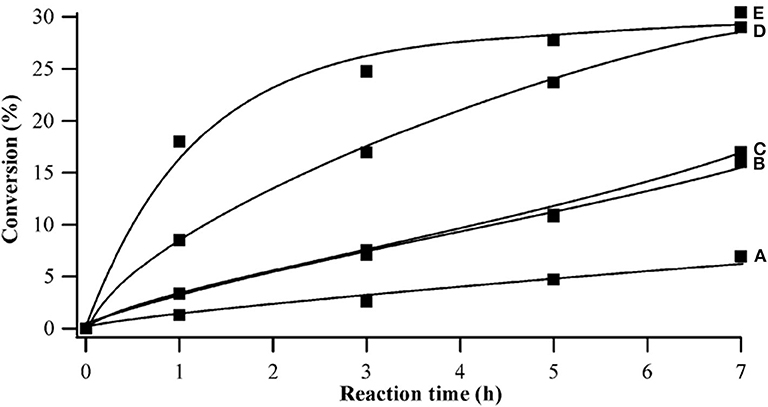
Figure 18. Effect of catalysts on conversion of acetic acid in esterification. Catalysts: (A) without catalyst, (B) micron-sized HPW/Cu3(BTC)2, (C) ultrastable Y zeolite (CBV 720), (D) 65 nm HPW/Cu3(BTC)2, and (E) 50 nm HPW/Cu3(BTC)2. Reaction conditions: 1 mol acetic acid, 40 mol 1-propanol, 2.23 wt% catalyst, 60°C. Reproduced with permission from Wee et al. (2010).
In 2011, Cheng et al. reported that a micellar HPA catalyst [C16H33N(CH3)3]H2PW12O40 (C16H2PW) was designed to hydrolyze cellulose and starch into glucose. The micellar HPA catalyst can allow cellulose molecules to enter into the catalytic sites, so as to improve the reaction rate and cellulose conversion rate (Dwars et al., 2005; Cheng et al., 2011). As a heterogeneous catalyst [C16H33N(CH3)3]H2PW12O40 exhibited remarkable catalytic performance for cellulose degradation, and the yield and selectivity of glucose were 39.3 and 89.1% respectively (Table 12). In addition, the catalyst was easily recovered by centrifuge and had good reusability (Figure 19). Therefore, the heterogeneous micelle HPA catalyst is a clean, economical and environmentally friendly catalyst for hydrolysis of cellulose.
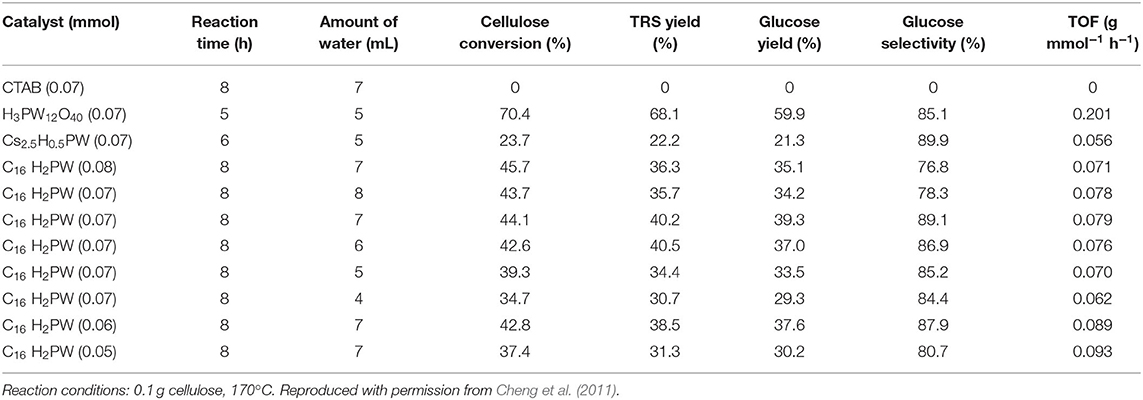
Table 12. Hydrolysis of cellulose over different catalysts (Cheng et al., 2011).
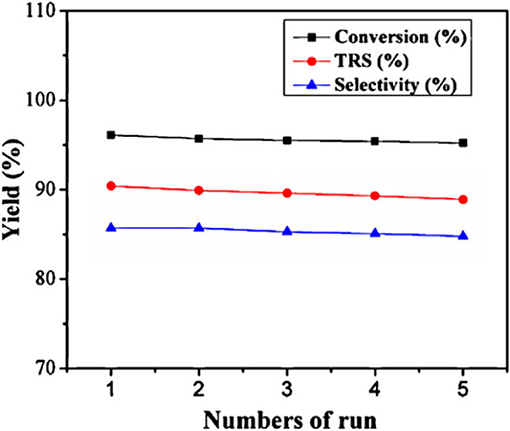
Figure 19. Recycling test of the [C16H33N(CH3)3]H2PW12O40 catalyst. Reaction conditions: 0.1 g cellulose, 0.07 mmol catalyst, 7 mL water, 170°C for 8 h. Reproduced with permission from Cheng et al. (2011).
In 2012, Sun et al. investigated a kind of HPA-ionic liquid catalysts [C4H6N2(CH2)3SO3H]3−nHnPW12O40 (n = 1, 2, 3) (abbreviated as [MIMPSH]nH32nPW), which can be used to degrade cellulose into water-soluble products such as glucose and levulinic acid (Sun et al., 2012). Glucose was the main product of hydrolysis of cellulose by the HPA-based on the ionic liquid catalyst, and the conversion of cellulose and the yield of glucose was 55.1 and 36.0%, respectively at 140°C for 5 h in a biphasic system (water–MIBK). Compared with the previously reported HPA catalysts, such as H3PW12O40 and Cs2.5H0.5PW112O40, the assembled catalyst showed much better catalytic performance because of its better solubility in water (Table 13). Moreover, the HPA-ionic liquids catalyst can also depolymerize other polysaccharides, from which high yields of glucose can be obtained (Scheme 17). More importantly, the HPA-ionic liquids catalyst can be completely recovered and reused six times without significant performance loss (Figure 20).
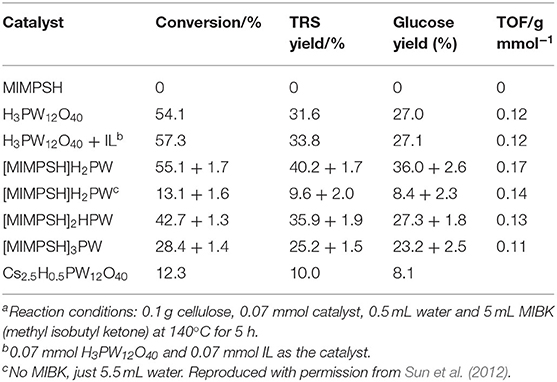
Table 13. Hydrolysis of cellulose with different catalystsa (Sun et al., 2012).
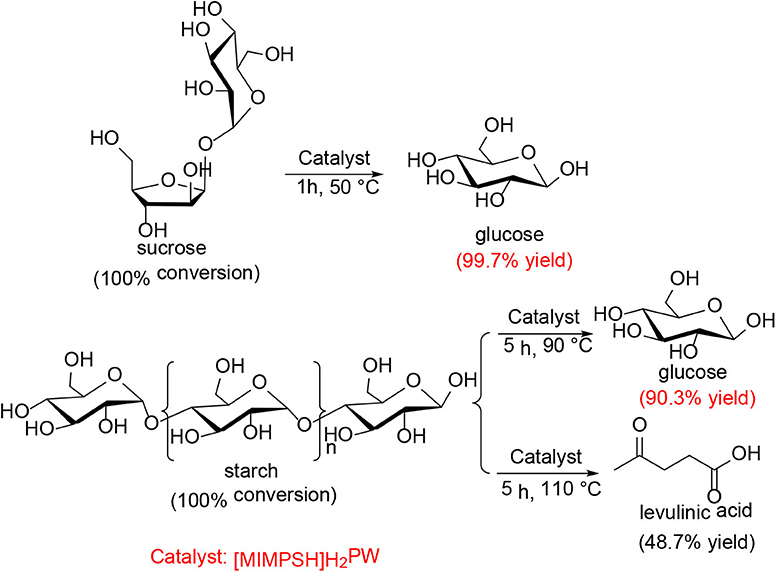
Scheme 17. Conversion of other polysaccharides over the [MIMPSH]H2PW catalyst. Reaction conditions: 0.077 mmol catalyst, 0.5 mL water, and 5.0 mL MIBK.
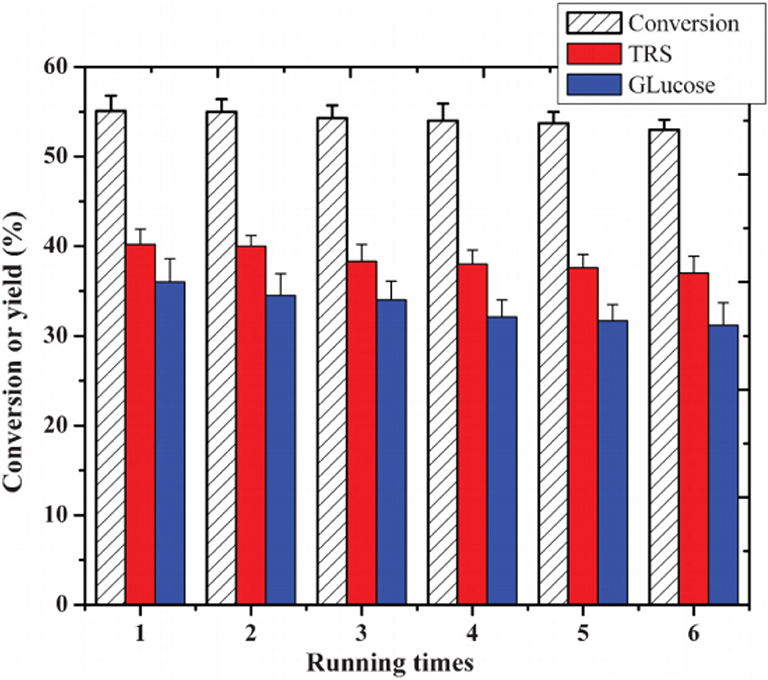
Figure 20. Reusability test of the [MIMPSH]H2PW catalyst. Reaction conditions: 0.1 g cellulose, 0.07 mmol catalyst, 0.5 mL H2O, 5 mL MIBK, 140°C for 5 h. Reproduced with permission from Sun et al. (2012).
In 2013, Chen et al. synthesized the polyvinylpyrrolidone-stabilized heteropolyacids (PVP–HPAs), a kind of reusable self-assembling catalysts for conversion of cellulose (Chen et al., 2013). A model for the synthesis of PVP-HPAs is shown in Scheme 18 and the alcoholysis diagram of cellulose is shown in Scheme 19. First, cellobiose alcoholysis, then dehydration between glucose and alcohol molecules, and final interconversion between β-alkylglucoside and α-alkylglucoside gives the product. The authors used a variety of characteristic techniques (e.g., FT-IR and Solid MAS 1H-NMR) to study the structure of the self-assembling catalysts (Figures 21A,B), and they found that HPAs can protonate PVP and the protonated PVP can form the structure similar to the ionic liquid and interact with heteropolyanion (Chen et al., 2013). Moreover, the structure of PVP assembled with HPA contributes to the easy separation and reuse of catalysts. After optimizing the content of PVP and HPA, the results showed that the self-assembling catalyst PVP–H4SiW12O40.5H2O (1/5:3/4) had excellent catalytic performance for the alcoholization of cellulose, and the conversion of cellulose was more than 60% (Chen et al., 2013). The application of the self-assembled catalyst is expected to expand to other reactions.
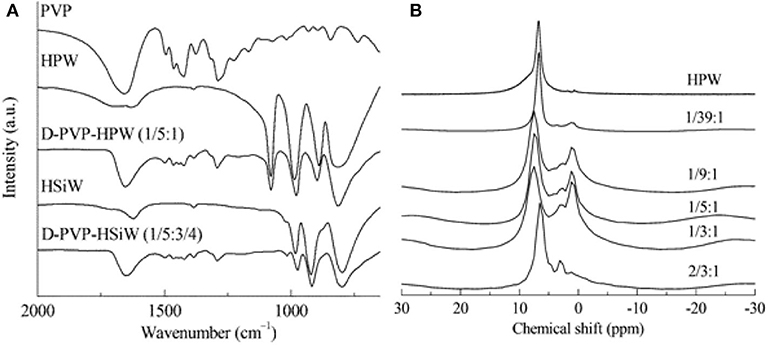
Figure 21. (A) The FT-IR spectra of PVP, HPAs, and PVP–HPAs, and (B) solid MAS 1H-NMR spectra of PVP–HPW catalysts. Reproduced with permission from Chen et al. (2013).
In 2015, Sun et al. synthesized an assembled HPA catalyst [C16H33N(CH3)3]xH6−xP2W18O62 (abbreviated as (C16TA)nH6−nP2W18O62). Wells–Dawson heteropolyacid H6P2W18O62 was used to synthesize the micellar assembly catalyst, which can catalyze the hydrolysis of cellulose to glucose (Sun et al., 2015). The mechanism of cellulose conversion by (C16TA)nH6−nP2W18O62 catalysts is shown in Scheme 20. The micelle catalyst first partially hydrolyzes cellulose to the oligomer, and then further degrades cellulose to glucose. Meanwhile, this catalyst can also promote the conversion of other polysaccharides, such as starch and cellobiose (Sun et al., 2015). The (C16TA)H5P2W18O62 (n = 1) catalyst exhibited higher catalytic activity than ordinary Keggin heteropoly acid catalyst H3PW12O40 (Table 14).
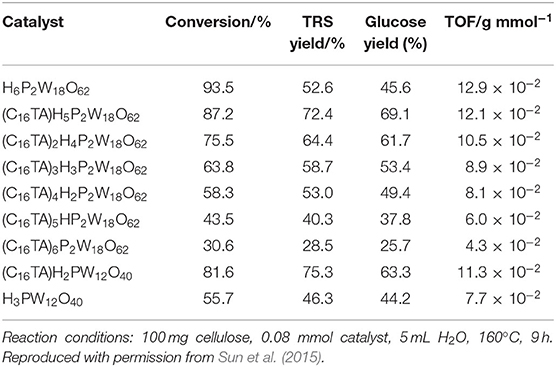
Table 14. Conversion of cellulose over different catalysts (Sun et al., 2015).
Sun et al. found that the high catalytic activity of the catalyst was due to the interaction of a high concentration of acid sites, which provided more opportunities for the substrate to enter the catalytic sites and the oxidation ability of (C16TA)nH6−nP2W18O62 (Sun et al., 2015). Moreover, this micellar assembled HPA catalyst was used as a heterogeneous catalyst and recycled by simple centrifuge (Figure 22).
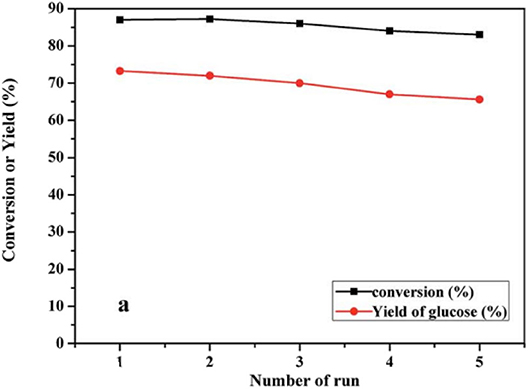
Figure 22. The circulation reaction test of the [C16H33N(CH3)3]H5P2W18O62 catalyst in conversion of cellulose to glucose. Reaction conditions: 100 mg cellulose, 0.08 mmol catalyst, 5 mL H2O, 160°C for 9 h. Reproduced with permission from Sun et al. (2015).
Lu et al. prepared heterogeneous catalysts (CnH2n+1N(CH3)3)H4PW11TiO40 (n = 4, 8, 12, 14, 16, and 18) composed of HPAs and amphiphilic quaternary ammonium salt by self-assembly method (Lu et al., 2015). The results showed that the heterogeneous catalyst (C16H33N(CH3)3)H4PW11TiO40 had the strongest acidity and exhibited excellent activity in the conversion of saccharides to other useful substances, with HMF yield of 53.7 and 50.8% from fructose and glucose, respectively (Figure 23). Meanwhile, the self-assembled structure of (C16H33N(CH3)3)H4PW11TiO40 in water could make cellulose and other polysaccharides gather to the catalytic sites, thus improving the catalytic efficiency. Also, the catalyst can be easily separated from the reactant, and the activity was almost no loss after recycling for many times (Figure 24).
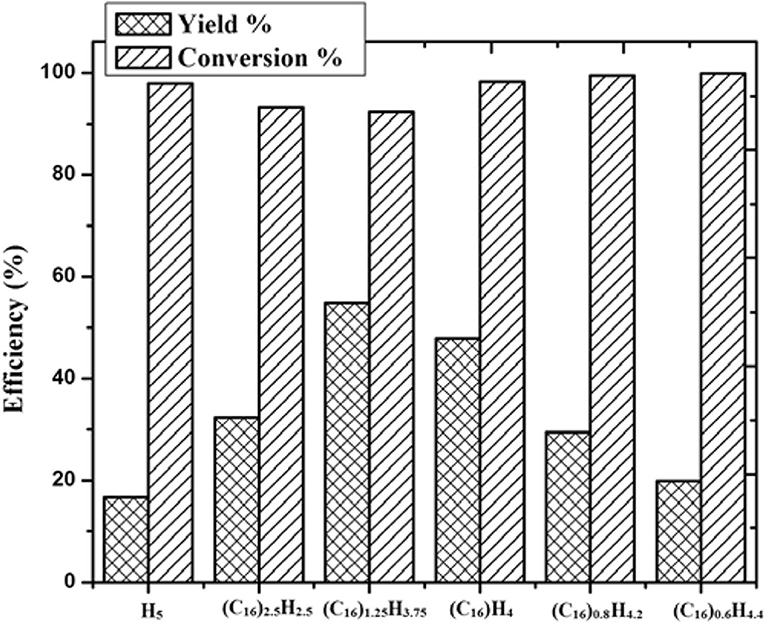
Figure 23. The efficiency of different catalysts for fructose transformation. Reaction conditions: 0.6 g fructose, 0.01 mmol catalyst, 2 mL H2O, 130°C for 1.5 h. Reproduced with permission from Lu et al. (2015).
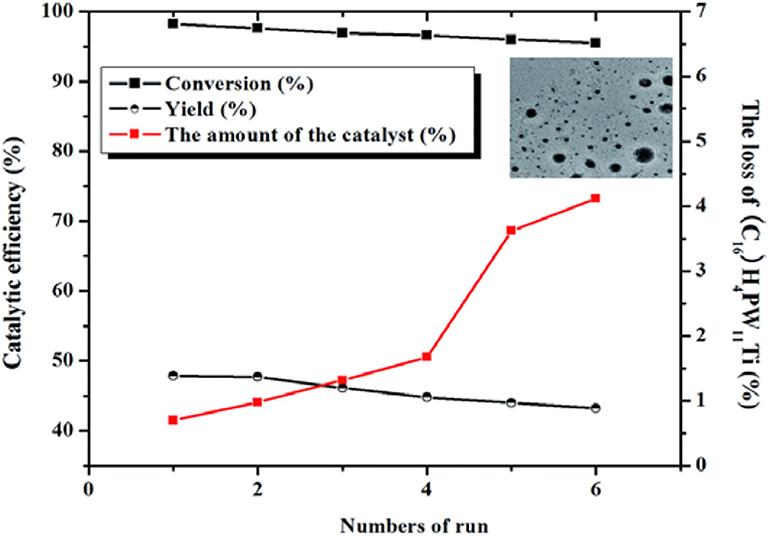
Figure 24. Recycling test of the (C16H33N(CH3)3)H4PW11TiO40 catalyst in the conversion of fructose to HMF. Reaction conditions: 0.6 g fructose, 0.01 mmol catalyst, 2 mL H2O, 130°C for 1.5 h. Reproduced with permission from Lu et al. (2015).
It can be seen that the assembled HPA catalysts can convert cellulose into glucose or other substances. Their unique structure can make cellulose aggregate to the catalytic sites, thus improving the catalytic efficiency. And almost all of them can be recycled by simple instruments. These advantages make their research more meaningful. However, it can not be ignored that some supported catalysts have low selectivity and yield for glucose, such as (C16TA)H5P2W18O62 and [MIMPSH]H2PW. Moreover, the stability of some HPA catalysts decreased after reuse, which should be improved by the exploration of novel preparation methods.
Other HPA Catalysts
In addition to the HPA catalysts mentioned above, many other HPAcatalysts have also been developed and utilized for cellulose degradation. For example, HPAs can be combined with other catalysts to facilitate cellulose conversion. Palkovits et al. proved that the combination of HPAs and supported Ru catalysts can directly convert cellulose into glycols with a yield of 81% (Palkovits et al., 2011). And the catalyst is very easy to recycle. In 2014, Xie et al. used HPA to synthesize a new catalyst Ru/[Bmim]3PW12O40, which can selectively convert microcrystalline cellulose to hexanol under mild conditions (Xie et al., 2014). The catalyst was synthesized by supporting Ru on ionic liquid (BmimPF6)-heteropoly acid (H3PW12O40·nH2O), which resulted in the formation of Brønsted acid sites. It improves the performance of the catalyst and is an important reason for the remarkable activity of the catalyst in the conversion of cellulose. Su et al. used HPA and ZrO2 to synthesize a kind of dual-functional heterogeneous catalyst (H3PW12O40/ZrO2-Et-HNS), which was composed of organosilicon hollow nanospheres and can be used in esterification (Su et al., 2014). The unique hollow nanospherical morphology of the catalyst increases its surface acidity and thus improves its catalytic activity. Basahel et al. (2016) synthesized a heterogeneous catalyst AlxH3xPW12O40 by ion-exchange method. The HPA catalysts formed by the exchange of hydrogen and aluminum ions showed higher activity because of the increase of Lewis acid sites on the surface of the catalysts. These catalysts proved to be able to synthesize bioactive pyrido[1,2-a]pyrimidines with yields above 90%. In 2017, Wu et al. synthesized a new luryamine-modified
phosphotungstic acid catalyst. They used several characterization instruments to characterize the catalyst and found that the modified heteropoly acid was still the Keggin structure (Wu et al., 2017). As compared with conventional phosphotungstic acid, the luryamine-modified HPA catalyst showed more stable and higher catalytic activity. It's worth mentioning that the yield of glucose reached 100% by hydrolysis of sucrose with the modified catalyst which can provide a way to study cellulose hydrolysis in the future. All the HPA catalysts reported above are heterogeneous. They have a common feature that they are easy to separate from reactants and can be reused with almost no loss of catalytic activity.
Comparison of Different HPA-Based Catalysts
As discussed above, it can be concluded that the Keggin HPA-based catalysts are one of the most common cellulose degradation catalysts. Due to their dual functional properties, the HPA-based catalysts can further promote the oxidation of hydrolysates into valuable compounds. However, their specific surface areas are relatively low, which is not conducive to the catalytic activity. To solve this deficiency, some substituted and supported heteropoly acid catalysts were developed, which are demonstrated to have higher specific surface areas, higher reactivity, and better thermal stability, and are more conducive to the separation because they are commonly used in the heterogeneous systems. It is worth noting that the preparation of these catalysts is complicated and the synthesis cycle is long. In order to further improve the catalytic activity of HPAs, the assembled HPAs and other modified HPA catalysts have been developed. With unique structures, these catalysts exhibit high catalytic performance in cellulose conversion. More importantly, they are all heterogeneous catalysts, providing convenience for the catalyst separation and recycling processes. Therefore, different HPA catalysts have their own characteristics, advantages and disadvantages, and the catalytic upgrading of cellulosic biomass can be effectively enabled with these HPA-based catalysts as long as their properties are properly switched.
Conclusions and Outlook
With the rapid development and utilization of chemical fuels, depolymerization of cellulosic biomass has become one of the ways to provide organic carbon sources. It is reported that cellulose can be converted into a variety of useful platform molecules that can be applied in various fields. In this review, the focus is placed on finding homogeneous and heterogeneous HPA catalysts for cellulose degradation.
HPAs, as a king of efficient, environmentally friendly, and safe catalysts, show high catalytic activity for cellulose degradation. HPA catalysts have higher catalytic activity than conventional solid acid catalysts, which are more conducive to the degradation of cellulose into valuable platform molecules, such as glucose. Meanwhile, due to its unique physical and chemical properties, HPAs can be used in homogeneous and heterogeneous systems. And they can all be reused after separation in the reactor with almost no loss of catalytic activity.
Several methods have been developed to synthesize HPA catalysts. (1) The acidification process coupled with an ether extraction is the most commonly used method, which is mainly used for the synthesis of common HPAs (e.g., H3PW12O40, H3SiW12O40), but its safety is low due to the use of some toxic substances. (2) The ion-exchange method has high safety, but it has the disadvantage of a long production cycle. (3) The impregnation method is simple and safe but suffers the disadvantage of the loss of catalyst activity and limited recycling ability. (4) The sol-gel method can prepare HPA catalysts at low temperatures or mild conditions, which is widely used in the synthesis of HPA-based nanomaterial catalysts. However, this method has the disadvantages of low security and long cycle. Therefore, it is very important and urgent to develop more efficient methods for the preparation of HPA-based catalysts.
As depicted in previous studies, typical Keggin-type HPA catalysts are the most common ones for cellulose degradation, and they can further catalyze the oxidation of the hydrolysates to valuable substances due to their dual functional properties. Then, the supported and substituted HPA catalysts always exist in heterogeneous form. After supporting and substituting disposal, the acid site number, the acid strength, and the specific surface area of the HPA catalysts increase, which improves the catalytic properties of the catalysts. In addition, the assembled HPA catalysts and other modified HPA catalysts show good catalytic performance in cellulose conversion because of their unique structure. Therefore, HPA-based catalysts provide convenience for cellulose degradation and more possibilities for the development of chemical fuels in the future. But it cannot be neglected that some of HPAs have the disadvantages of high catalyst dosage and relatively low catalytic activity with respect to the reaction conversion, product selectivity, and catalyst stability. For example, the selectivity of substituted and supported HPAs toward target products is lower than that of Keggin-type HPAs. Also, the stability of the supported HPAs is lower than that of common HPAs.
Although HPAs-based catalysts in either homogeneous or heterogeneous state have made great progress in the degradation of biomass cellulose, there are still many deficiencies to be improved:
(1) Study on the relationship between the properties of HPA catalysts and the degradation of cellulose to further improve the catalytic activity.
(2) Design of a cleaner, safer, and more effective HPA catalyst with simple preparation methods to promote cellulose degradation.
(3) Development of more new HPA catalysts with special structure, and ensure no or fewer side reactions in the reaction process, with the focus on the improvement of the HPA catalyst disadvantages especially unsatisfactory conversion, selectivity, and stability.
(4) Further understanding the structural characteristics of HPAs, study of the reaction mechanism catalyzed by HPAs, and promotional completion of the target reaction by linking the relationship between them.
(5) Extension of HPA catalysts to other biomass feedstocks, providing more help for the future development of fuels and HPAs in the field of catalysis.
In summary, this review mainly summarizes some examples of the degradation of cellulose by some kinds of HPA catalysts. The HPA catalysts are considered to have great application prospects in the degradation of cellulose and the conversion of other relevant research fields.
Author Contributions
XL and HL initiated the project. XL, HW, CL, ZL, HL, HZ, YL, YS, and SY searched the data and wrote, revised, and completed the manuscript. All authors contributed to the article and approved the submitted version.
Funding
The authors thank the financial support from the National Natural Science Foundation of China (21666008, 21908033, and 21576059), Fok Ying-Tong Education Foundation (161030), Guizhou Science & Technology Foundation ([2018]1037), and Program of Introducing Talents of Discipline to Universities of China (111 Program, D20023).
Conflict of Interest
The authors declare that the research was conducted in the absence of any commercial or financial relationships that could be construed as a potential conflict of interest.
References
Ahmed, A. I., El-Hakam, S. A., Elghany, M. A. A., and El-Yazeed, W. S. A. (2011). Synthesis and characterization of new solid acid catalysts, H3PW12O40 supported on nanoparticle tin oxide: An efficient catalyst for the preparation of 7-hydroxy-4-methylcoumarin. Appl. Catal. A Gen. 407, 40–48. doi: 10.1016/j.apcata.2011.08.020
Akiyama, G., Matsuda, R., Sato, H., Takata, M., and Kitagawaet, S. (2011). Cellulose hydrolysis by a new porous coordination polymer decorated with sulfonic acid functional groups. Adv. Mater. 23, 3294–3297. doi: 10.1002/adma.201101356
Albert, J., Luders, D., Bosmann, A., Guldi, D. M., and Wasserscheid, P. (2014). Spectroscopic and electrochemical characterization of heteropoly acids for their optimized application in selective biomass oxidation to formic acid. Green Chem. 16, 226–237. doi: 10.1039/C3GC41320A
Albert, J., Wolfel, R., Bosmann, A., and Wasserscheid, P. (2012). Selective oxidation of complex, water-insoluble biomass to formic acid using additives as reaction accelerators. Energy Environ. Sci. 5, 7956–7962. doi: 10.1039/c2ee21428h
An, D., Ye, A., Deng, W., Zhang, Q., and Wang, Y. (2012). Selective conversion of cellobiose and cellulose into gluconic acid in water in the presence of oxygen, catalyzed by polyoxometalate-supported gold nanoparticles. Chem. Eur. J. 18, 2938–2947. doi: 10.1002/chem.201103262
Bachillerbaeza, B., and Anderson, J. (2004). FTIR and reaction studies of the acylation of anisole with acetic anhydride over supported hpa catalysts. J. Catal. 228, 225–233. doi: 10.1016/j.jcat.2004.08.010
Bamoharram, F. F., Heravi, M. M., Heravi, H. M., and Dehghan, M. (2004). Photocatalytic oxidation of benzyl alcohols in the presence of H14[NaP5W30O110] as a green and reusable catalyst. Synth. React. Inorg. Metal Org. Nano Metal Chem. 39, 394–399. doi: 10.1080/15533170903129745
Basahel, S. N., Ahmed, N. S., Narasimharao, K., and Mokhtar, M. (2016). Simple and efficient protocol for synthesis of pyrido [1, 2-a] pyrimidin-4-one derivatives over solid heteropolyacid catalysts. RSC Adv. 6, 11921–11932. doi: 10.1039/C5RA22180C
Bechtold, M. F., and Square, K. (1950). Method of Preparing Heteropolyacids Containing a Heavy Metal. U.S. Patent No. 2503991.
Bennardi, D., Romanelli, G., Autino, J., Pizzio, L., Vázquez, P., Cáceres, C., et al. (2010). Comparative study of the catalytic preparation of flavones using keggin heteropolyacids under homogeneous, heterogeneous and solvent free conditions. React. Kinet. Mech. Catal. 100, 165–174. doi: 10.1007/s11144-010-0172-4
Cardoso, L. A. M., Alves, W., Gonzaga, A. R. E., Aguiar, L. G., and Andrade, H. M. C. (2004). Friedel-crafts acylation of anisole with acetic anhydride over silica-supported heteropolyphosphotungstic acid (HPW/SiO2). J. Mol. Catal. A Chem. 209, 189–197. doi: 10.1016/j.molcata.2003.08.022
Chang, F., Hanna, M. A., Zhang, D., Li, H., Zhou, Q., Song, B., et al. (2013). Production of biodiesel from non-edible herbaceous vegetable oil: Xanthium sibiricum Patr. Bioresour. Technol. 140, 435–438. doi: 10.1016/j.biortech.2013.04.111
Chen, J., Fang, X., Duan, X., Ye, L., Lin, H., and Yuan, Y. (2013). PVP-stabilized heteropolyacids as reusable self-assembling catalysts for alcoholysis of cellulosic saccharides. Green Chem. 16, 294–302. doi: 10.1039/C3GC40994E
Cheng, M., Tian, S., Guan, H., Wang, S., Wang, X., and Jiang, Z. J. (2011). Clean production of glucose from polysaccharides using a micellar heteropolyacid as a heterogeneous catalyst. Appl. Catal. B Environ. 107, 104–109. doi: 10.1016/j.apcatb.2011.07.002
Chheda, J. N., Huber, G. W., and Dumesic, J. A. (2007). Liquid-phase catalytic processing of biomass-derived oxygenated hydrocarbons to fuels and chemicals. Angew. Chem. Int. Ed. 46, 7164–7183. doi: 10.1002/anie.200604274
Chiola, V., and Lawrence, J. G. (1969). Preparation of Heteropoly Molybdic Acids. U.S. Patent No. 3425794. Washington, DC: U.S. Patent and Trademark Office.
Chiola, V., vanderpool, C. D., and Towanda, P. (1969). Method of Preparing Heteropoly Compounds. U.S. Patent No. 3446575. Washington, DC: U.S. Patent and Trademark Office.
Climent, M. J., Corma, A., and Iborra, S. (2014). Conversion of biomass platform molecules into fuel additives and liquid hydrocarbon fuels. Green Chem. 16, 516–547. doi: 10.1039/c3gc41492b
de Op Beeck, B., Geboers, J., and van de Vyver, S. (2013). Conversion of (ligno)cellulose feeds to isosorbide with heteropoly acids and Ru on carbon. ChemSusChem 6, 199–208. doi: 10.1002/cssc.201200610
Deng, W., Zhang, Q., and Wang, Y. (2012). Polyoxometalates as efficient catalysts for transformations of cellulose into platform chemicals. Dalton Trans. 41, 9817–9831. doi: 10.1039/c2dt30637a
Dhepe, P. L., and Fukuoka, A. (2008). Cellulose conversion under heterogeneous catalysis. ChemSusChem 1, 969–975. doi: 10.1002/cssc.200800129
Duan, X., Sun, G., Sun, Z., Li, J., Wang, S., Wang, X., et al. (2013). A heteropolyacid-based ionic liquid as a thermoregulated and environmentally friendly catalyst in esterification reaction under microwave assistance. Catal. Commun. 42, 125–128. doi: 10.1016/j.catcom.2013.08.014
Dwars, T., Paetzold, E., and Oehme, G. (2005). Reactions in micellar systems. Angew. Chem. Int. Ed. 44, 7174–7199. doi: 10.1002/anie.200501365
Feng, J., Zhu, Q., Ding, M., Liu, X., and Han, X. (2011). Direct conversion and nmr observation of cellulose to glucose and 5-hydroxymethylfurfural (HMF) catalyzed by the acidic ionic liquids. J Mol. Catal. A Chem. 334, 8–12. doi: 10.1016/j.molcata.2010.10.006
Furukawa, H., Kobayashi, N., Itaya, Y., Satsuma, A., and Shimizu, K. (2009). Effects of brøNsted and lewis acidities on activity and selectivity of heteropolyacid-based catalysts for hydrolysis of cellobiose and cellulose. Green Chem. 11, 1627–1632. doi: 10.1039/b913737h
Geboers, J., van de Vyver, S., Carpentier, K., de Blochouse, K., Jacobs, P., and Sels, B. (2010). Efficient catalytic conversion of concentrated cellulose feeds to hexitols with heteropoly acids and Ru on carbon. Chem. Commun. 46, 3577–3580. doi: 10.1039/c001096k
Geboers, J., van de Vyver, S., Carpentier, K., Jacobs, P., and Sels, B. (2011). Hydrolytic hydrogenation of cellulose with hydrotreated caesium salts of heteropoly acids and Ru/C. Green Chem. 13, 2167–2174. doi: 10.1039/c1gc15350a
Gromov, N. V., Taran, O. P., Delidovich, I. V., Pestunov, A. V., Rodikova, Y. A., Yatsenko, D. A., et al. (2016). Hydrolytic oxidation of cellulose to formic acid in the presence of Mo-V-P heteropoly acid catalysts. Catal. Today 278, 74–81. doi: 10.1016/j.cattod.2016.03.030
Guo, Y., Li, K., and Clark, J. H. (2007). The synthesis of diphenolic acid using the periodic mesoporous H3PW12O40-silica composite catalysed reaction of levulinic acid. Green Chem. 9, 839–841 doi: 10.1039/b702739g
Hafizi, A., Ahmadpour, A., Heravi, M. M., and Bamoharram, F. F. (2014). The application of silica-supported preyssler hpa as a heterogeneous and green catalyst for the alkylation of benzene. Petrol. Sci. Technol. 32, 1022–1027. doi: 10.1080/10916466.2011.637534
Harada, T., Tokai, Y., Kimura, A., Ikeda, S., and Matsumura, M. (2014). Hydrolysis of crystalline cellulose to glucose in an autoclave containing both gaseous and liquid water. RSC Adv. 4, 26838–26842. doi: 10.1039/c4ra02396j
He, J., Li, H., Riisager, A., and Yang, S. (2018). Catalytic transfer hydrogenation of furfural to furfuryl alcohol with recyclable Al–Zr@Fe mixed oxides. ChemCatChem 10, 430–438. doi: 10.1002/cctc.201701266
Heravi, M. M., Jani, B. A., Derikvand, F., Bamoharram, F. F., and Oskooie, H. A. (2008). Three component, one-pot synthesis of dihydropyrano[3,2-c]chromene derivatives in the presence of H6P2W18O62·18H2O as a green and recyclable catalyst. Catal. Commun. 10, 272–275. doi: 10.1016/j.catcom.2008.08.023
Heravi, M. M., Khorasani, M., Derikvand, F., Oskooie, H. A., and Bamoharram, F. F. (2007). Highly efficient synthesis of coumarin derivatives in the presence of H14[NaP5W30O110] as a green and reusable catalyst. Catal. Commun. 8, 1886–1890. doi: 10.1016/j.catcom.2007.02.030
Hu, S., Jiang, F., and Hsieh, Y. L. (2015). 1D lignin-based solid acid catalysts for cellulose hydrolysis to glucose and nanocellulose. ACS Sustain. Chem. Eng. 3, 2566–2574. doi: 10.1021/acssuschemeng.5b00780
Huber, G. W., Iborra, S., and Corma, A. (2006). Synthesis of transportation fuels from biomass: chemistry, catalysts, and engineering. Chem. Rev. 106, 4044–4098. doi: 10.1021/cr068360d
Izumi, Y., Matsuo, K., and Urabe, K. (1983). Efficient homogeneous acid catalysis of heteropoly acid and its characterization through ether cleavage reactions. J. Mol. Catal. 18, 299–314. doi: 10.1016/S0304-5102(83)80004-2
Izumi, Y., Ono, M., Kitagawa, M., Yoshida, M., and Urabe, K. (1995). Silica-included heteropoly compounds as solid acid catalysts. Microporous Mater. 5, 255–262. doi: 10.1016/0927-6513(95)00059-3
Kamm, B., and Kamm, M. (2004). Principles of biorefineries. Appl. Microbiol. Biotechnol. 64, 137–145. 10.1007/s00253-003-1537-7 doi: 10.1007/s00253-003-1537-7
Kaur, J., and Kozhevnikov, I. V. (2002). Efficient acylation of toluene and anisole with aliphatic carboxylic acids catalysed by heteropoly salt Cs2.5H0.5PW12O40. Chem. Commun. 8, 2508–2509. doi: 10.1039/b207915c
Klein, M., Pulidindi, I. N., Perkas, N., and Gedanken, A. (2015). Heteropoly acid catalyzed hydrolysis of glycogen to glucose. Biomass Bioenergy 76, 61–68. doi: 10.1016/j.biombioe.2015.02.036
Klein, M., Pulidindi, I. N., Perkas, N., Meltzer-Mats, E., Gruzman, A., and Gedanken, A. (2012). Direct production of glucose from glycogen under microwave irradiation. RSC Adv. 2, 7262–7267. doi: 10.1039/c2ra21066e
Klemm, D., Heublein, B., Fink, H. P., and Bohn, A. (2005). Cellulose: fascinating biopolymer and sustainable raw material. Angew. Chem. Int. Ed. 44, 3358–3393. doi: 10.1002/anie.200460587
Kobayashi, H., Komanoya, T., Hara, K., and Fukuoka, A. (2010). Water-tolerant mesoporous-carbon-supported ruthenium catalysts for the hydrolysis of cellulose to glucose. ChemSusChem 3, 440–443. doi: 10.1002/cssc.200900296
Komanoya, T., Kobayashi, H., Hara, K., Chun, W. J., and Fukuoka, A. (2011). Catalysis and characterization of carbon-supported ruthenium for cellulose hydrolysis. Appl. Catal. A Gen. 407, 188–194. doi: 10.1016/j.apcata.2011.08.039
Kozhevnikov, I. V. (1987). Advances in catalysis by heteropolyacids. Russ. Chem. Rev. 56, 811–825. doi: 10.1070/RC1987v056n09ABEH003304
Kozhevnikov, I. V. (1998). Catalysis by heteropoly acids and multicomponent polyoxometalates in liquid-phase reactions. Chem. Rev. 98, 171–198. doi: 10.1021/cr960400y
Laferty, J. M., and John, M. (1966). Method of Preparing Phosphotungstic Acid. U.S. Patent No. 3288562. Washington, DC: U.S. Patent and Trademark Office.
Lai, D. M., Deng, L., Guo, Q. X., and Fu, Y. (2011). Hydrolysis of biomass by magnetic solid acid. Energy. Environ. Sci. 4, 3552–3557. doi: 10.1039/c1ee01526e
Lanzafame, P., Temi, D. M., Perathoner, S., Spadaro, A. N., and Centi, G. (2012). Direct conversion of cellulose to glucose and valuable intermediates in mild reaction conditions over solid acid catalysts. Catal. Today 179, 178–184. doi: 10.1016/j.cattod.2011.07.018
Leng, Y., Wang, J., Zhu, D., Ren, X., Ge, H., and Shen, L. (2010). Heteropolyanion-based ionic liquids: reaction-induced self-separation catalysts for esterification. Angew. Chem. 48, 168–171. doi: 10.1002/anie.200803567
Li, H., Fang, Z., and Yang, S. (2016). Direct catalytic transformation of biomass derivatives into biofuel component γ-valerolactone with magnetic nickel-zirconium nanoparticles. ChemPlusChem 81, 135–142. doi: 10.1002/cplu.201500492
Li, H., He, X., Zhang, Q., Chang, F., Xue, W., Zhang, Y., et al. (2013). Polymeric ionic hybrid as solid acid catalyst for the selective conversion of fructose and glucose to 5-hydroxymethylfurfural. Energy Technol. 1, 151–156. doi: 10.1002/ente.201200041
Li, H., Liu, X., Yang, T., Zhao, W., Saravanamurugan, S., and Yang, S. (2015). Porous zirconium–furandicarboxylate microspheres for efficient redox conversion of biofuranics. ChemSusChem 10, 1761–1770. doi: 10.1002/cssc.201601898
Li, H., Zhang, Q., Bhadury, P. S., and Yang, S. (2014). Furan-type compounds from carbohydrates via heterogeneous catalysis. Curr. Org. Chem. 18, 547–597. doi: 10.2174/13852728113176660138
Liu, B., and Zhang, Z. H. (2015). Catalytic conversion of biomass into chemicals and fuels over magnetic catalysts. ACS Catal. 6, 326–338. doi: 10.1021/acscatal.5b02094
Liu, Q. Y., Wu, W. L., Wang, J., Ren, X. Q., and Wang, Y. R. (2004). Characterization of 12-tungstophosphoric acid impregnated on mesoporous silica SBA-15 and its catalytic performance in isopropylation of naphthalene with isopropanol. Micropor. Mesopor. Mater. 76, 51–60. doi: 10.1016/j.micromeso.2004.08.001
Liu, X., Li, H., Pan, H., Zhang, H., Huang, S., Yang, K., et al. (2016). Efficient catalytic conversion of carbohydrates into 5-ethoxymethylfurfural over MIL-101-based sulfated porous coordination polymers. J. Energy Chem. 25, 523–530. doi: 10.1016/j.jechem.2016.01.015
Lu, T., Niu, M., Hou, Y., Wu, W., Ren, S., and Yang, F. (2016). Catalytic oxidation of cellulose to formic acid in H5PV2Mo10O40 + H2SO4 aqueous solution with molecular oxygen. Green Chem. 18, 4725–4732. doi: 10.1039/C6GC01271J
Lu, Y., Sun, Z., and Huo, M. (2015). Fabrication of a micellar heteropolyacid with lewis–brønsted acid sites and application for the production of 5-hydroxymethylfurfural from saccharides in water. RSC Adv. 5, 30869–30876. doi: 10.1039/C4RA16952B
Macht, J., Janik, M. J., Neurock, M., and Iglesia, E. (2008). Mechanistic consequences of composition in acid catalysis by polyoxometalate keggin clusters. J. Am. Chem. Sci. 130, 10369–71039. doi: 10.1021/ja803114r
Mastikhin, V. M., Kulikov, S. M., Nosov, A. V., Kozhevnikov, I. V., and Timofeeva, M. N. (1990). 1H and 31P mas nmr studies of solid heteropolyacids and H3PW12O40 supported on SiO2. J. Mol. Catal. A Chem. 60, 65–70. doi: 10.1016/0304-5102(90)85068-S
Mizuno, N., and Misono, M. (1994). Heteropolyanions in catalysis. J. Mol. Catal. 86, 319–342. doi: 10.1016/0304-5102(93)E0155-A
Mrowiec-Białoń, J., Turek, W. A., and Jarzebski, B. (2002). Preparation of highly active heteropolyacid-silica composite catalysts using the sol-gel method. React. Kinet. Catal. Lett. 76, 213–219. doi: 10.1023/A:1016515407161
Nowinska, K., Dudko, D., and Golon, R. (1996). Pd2+Mn2+HPA: a heterogeneous wacker system catalyst. Chem. Commun. 2, 277–297. doi: 10.1039/CC9960000277
Odyakov, V. F., and Zhizhina, E. G. (2009). New process for preparing aqueous solutions of mo-v-phosphoric heteropoly acids. Russ. J. Inorg. Chem. 54, 361–367. doi: 10.1134/S003602360903005X
Ogasawara, Y., Itagaki, S., Yamaguchi, K., and Mizuno, N. (2011). Saccharification of natural lignocellulose biomass and polysaccharides by highly negatively charged heteropolyacids in concentrated aqueous solution. ChemSusChem 4, 519–525. doi: 10.1002/cssc.201100025
Okuhara, T. (2002). Water-tolerant solid acid catalysts. Chem Rev. 102, 3641–3665. doi: 10.1021/cr0103569
Okuhara, T., Nishimura, T., and Misono, M. (1996). Novel microporous solid “superacids”: CsxH3−xPW12O40 (2 < =x < =3). Stud. Surf. Sci. Catal. 101, 581–590. doi: 10.1016/S0167-2991(96)80269-2
Okuhara, T., Nishimura, T., Watanabe, H., and Misono, M. (1992). Insoluble heteropoly compounds as highly active catalysts for liquid-phase reactions. J. Mol. Catal. 74, 247–256. doi: 10.1016/0304-5102(92)80242-9
Okuhara, T., Watanabe, H., Nishimura, T., Inumaru, K., and Misono, M. (2000). Microstructure of cesium hydrogen salts of 12-tungstophosphoric acid relevant to novel acid catalysis. Chem. Mater. 12, 2230–2238. doi: 10.1021/cm9907561
Palkovits, R., Tajvidi, K., Ruppert, A., and Procelewska, J. (2011). Heteropoly acids as efficient acid catalysts in the one-step conversion of cellulose to sugar alcohols. Chem. Commun. 47, 576–578. doi: 10.1039/C0CC02263B
Pan, T., Deng, J., Xu, Q., Zuo, Y., Guo, Q. X., and Fu, Y. (2013). Catalytic conversion of furfural into a 2,5-furandicarboxylic acid-based polyester with total carbon utilization. ChemSusChem 6, 47–50. doi: 10.1002/cssc.201200652
Pang, J., Wang, A., Zheng, M., and Zhang, T. (2010). Hydrolysis of cellulose into glucose over carbons sulfonated at elevated temperatures. Chem.Commun. 46, 6935–6937. doi: 10.1039/c0cc02014a
Parghi, K. D., Satam, J. R., and Jayaram, R. V. (2011). Silica supported heteropolyacid catalyzed dehydration of aldoximes to nitriles and alcohols to alkenes. Green Chem. Lett. Rev. 4, 143–149. doi: 10.1080/17518253.2010.523015
Reddy, B. S., Narasimhulu, G., Lakshumma, P. S., Reddy, Y. V., and Yadav, J. S. (2012). Phosphomolybdic acid: a highly efficient solid acid catalyst for the synthesis of trans-4, 5-disubstituted cyclopentenones. Tetrahedron Lett. 53, 1776–1779. doi: 10.1016/j.tetlet.2012.01.115
Ren, H., Girisuta, B., Zhou, Y., and Liu, L. (2015). Selective and recyclable depolymerization of cellulose to levulinic acid catalyzed by acidic ionic liquid. Carbohydr. Polym. 117, 569–576. doi: 10.1016/j.carbpol.2014.09.091
Rinaldi, R., Palkovits, R., and Schüth, F. (2010). Depolymerization of cellulose using solid catalysts in ionic liquids. Angew. Chem. Int. Ed. 47, 8047–8050. doi: 10.1002/anie.200802879
Shatalov, A. A. (2019). Highly efficient hydrolysis of plant hemicelluloses by mixed-addenda keggin-type (Mo-V-P)-heteropolyacids in diluted aqueous solution. Carbohydr. Polym. 206, 80–85. doi: 10.1016/j.carbpol.2018.10.106
Sheldon, R. A. (2014). Green and sustainable manufacture of chemicals from biomass: state of the art. Green Chem. 16, 950–963. doi: 10.1039/C3GC41935E
Shimizu, K., and Satsuma, A. (2011). Toward a rational control of solid acid catalysis for green synthesis and biomass conversion. Energy Environ. Sci. 4, 3140–3153. doi: 10.1039/c1ee01458g
Song, I. K., and Lee, W. Y. (2004). Heteropolyacid (HPA)-polymer composite films as heterogeneous catalysts and catalytic membranes. Appl. Catal. A Gen. 256, 77–98. doi: 10.1016/S0926-860X(03)00390-9
Su, F., An, S., Song, D., Zhang, X., Lu, B., and Guo, Y. (2014). Heteropoly acid and ZrO2 bifunctionalized organosilica hollow nanospheres for esterification and transesterification. J. Mater. Chem. 2, 14127–14138. doi: 10.1039/C4TA02257B
Sun, Z., Cheng, M., Li, H., Shi, T., Yuan, M., Wang, X., et al. (2012). One-pot depolymerization of cellulose into glucose and levulinic acid by heteropolyacid ionic liquid catalysis. RSC Adv. 2, 9058–9065. doi: 10.1039/c2ra01328b
Sun, Z., Zhang, X., Wang, S., Li, X., Wang, X., and Shi, J. (2015). Hydrolysis and alcoholysis of polysaccharides with high efficiency catalyzed by a (C16TA)xH6−xP2W18O62 nanoassembly. RSC Adv. 5, 94155–94163. doi: 10.1039/C5RA15047G
Tian, J., Fang, C., Cheng, M., and Wang, X. (2011). Hydrolysis of cellulose over CsxH3−xPW12O40 (x = 1–3) heteropoly acid catalysts. Chem. Eng. Technol. 34, 482–486. doi: 10.1002/ceat.201000409
Tian, J., Wang, J. H., Zhao, S., Jiang, C. Y., Zhang, X., and Wang, X. H. (2010). Hydrolysis of cellulose by the heteropoly acid H3PW12O40. Cellulose 17, 587–594. doi: 10.1007/s10570-009-9391-0
To, A. T., Chung, P. W., and Katz, A. (2015). Weak-acid sites catalyze the hydrolysis of crystalline cellulose to glucose in water: importance of post synthetic functionalization of the carbon surface. Angew. Chem. Int. Ed. 127, 11202–11205. doi: 10.1002/ange.201504865
Udayakumar, S., Ajaikumar, S., and Pandurangan, A. (2007). Electrophilic substitution reaction of phenols with aldehydes: enhance the yield of bisphenols by hpa and supported HPA. Catal. Commun. 8, 366–374. doi: 10.1016/j.catcom.2006.05.054
Vázquez, P., Pizzio, L., Cáceres, C., Blanco, M., Thomas, H., Alesso, E., et al. (2000). Silica-supported heteropolyacids as catalysts in alcohol dehydration reactions. J. Mol. Catal. A Chem.16, 223–232. doi: 10.1016/S1381-1169(00)00346-0
Wang, H., Gurau, G., and Rogers, R. D. (2012). Ionic liquid processing of cellulose. Chem. Soc. Rev. 41, 1519–1537. doi: 10.1039/c2cs15311d
Wee, L. H., Bajpe, S. R., Janssens, N., Hermans, I., and Martens, J. A. (2010). Convenient synthesis of Cu3(BTC)2 encapsulated keggin heteropolyacid nanomaterial for application in catalysis. Chem. Commun. 46, 8186–8188. doi: 10.1039/c0cc01447h
Wölfel, R., Taccardi, N., Bösmann, A., and Wasserscheid, P. (2011). Selective catalytic conversion of biobased carbohydrates to formic acid using molecular oxygen. Green Chem. 13, 2759–2763. doi: 10.1039/c1gc15434f
Wu, L., Zhu, B., and Wu, Y. (2017). “Hydrophobic lurylamine modified heteropoly acid as an efficient and recyclable catalyst for the hydrolysis reaction in aqueous solution under microwave,” in The 4th Annual International Conference on Information Technology and Applications (ITA 2017) (Guangzhou), 12:04026. doi: 10.1051/itmconf/20171204026
Wu, Y., Fu, Z., Yin, D., Xu, Q., Liu, F., Lu, C., et al. (2010). Microwave-assisted hydrolysis of crystalline cellulose catalyzed by biomass char sulfonic acids. Green Chem. 12, 696–700. doi: 10.1039/b917807d
Xie, X., Han, J., Wang, H., Zhu, X., Liu, X., Niu, Y., et al. (2014). Selective conversion of microcrystalline cellulose into hexitols over a Ru/[Bmim]3PW12O40 catalyst under mild conditions. Catal. Today 233, 70–76. doi: 10.1016/j.cattod.2013.09.061
Yabushita, M., Kobayashi, H., and Fukuoka, A. (2014). Catalytic transformation of cellulose into platform chemicals. Appl. Catal. B Environ. 145, 1–9. doi: 10.1016/j.apcatb.2013.01.052
Yan, L., Greenwood, A. A., Hossain, A., and Yang, B. (2014). A comprehensive mechanistic kinetic model for dilute acid hydrolysis of switchgrass cellulose to glucose, 5-HMF and levulinic acid. RSC Adv. 4, 23492–23478. doi: 10.1039/c4ra01631a
Yang, L., Qi, Y., Yuan, X., Shen, J., and Kim, J. (2005). Direct synthesis, characterization and catalytic application of SBA-15 containing heteropolyacid H3PW12O40. J. Mol. Catal. A Chem. 22, 199–205. doi: 10.1016/j.molcata.2004.11.024
Yang, P., Kobayashi, H., and Fukuoka, A. (2011). Recent developments in the catalytic conversion of cellulose into valuable chemicals. Chin. J. Catal. 32, 716–722. doi: 10.1016/S1872-2067(10)60232-X
Yu, F., Kong, X. J., Zheng, Y. Y., Ren, Y. P., Long, L. S., Huang, R. B., et al. (2009a). Ph-dependent assembly of 0D to 3D keggin-based coordination polymers: structures and catalytic properties. Dalton Trans. 43, 9503–9509 doi: 10.1039/b911606k
Yu, S., Brown, H. M., Huang, X., Zhou, X.-,d., Amonette, J. E., and Zhang, Z. (2009b). Single-step conversion of cellulose to 5-hydroxymethylfurfural (HMF), a versatile platform chemical. Appl. Catal. A Gen. 361, 117–122. doi: 10.1016/j.apcata.2009.04.002
Yusuke, K., and Urabe, K. (1981). Catalysis of heteropoly acids entrapped in activated carbon. Chem. Lett. 10, 663–666,. doi: 10.1246/cl.1981.663
Zhang, F., Deng, X., Fang, Z., Zeng, H., Tian, X., and Kozinski, J. (2011). Hydrolysis of microcrystalline cellulose over Zn-Ca-Fe oxide catalyst. Petrochem. Technol. 40, 43–48. doi: 10.1016/S1872-5805(11)60067-X
Zhang, H., Li, H., Pan, H., Wang, A., Souzanchi, S., Xu, C., et al. (2018). Magnetically recyclable acidic polymeric ionic liquids decorated with hydrophobic regulators as highly efficient and stable catalysts for biodiesel production. Appl. Energ. 223, 416–429. doi: 10.1016/j.apenergy.2018.04.061
Zhang, H., Zhou, Q., Chang, F., Pan, H., Liu, X., Li, H., et al. (2015). Production and fuel properties of biodiesel from Firmiana platanifolia L.f. as a potential non-food oil source. Ind. Crop. Prod. 76, 768–771. doi: 10.1016/j.indcrop.2015.08.002
Zhang, J., Liu, X., Sun, M., Ma, X., and Han, Y. (2012). Direct conversion of cellulose to glycolic acid with a phosphomolybdic acid catalyst in a water medium. ACS Catal. 2, 1698–1702. doi: 10.1021/cs300342k
Zhang, J., Sun, M., Liu, X., and Han, Y. (2014). Catalytic oxidative conversion of cellulosic biomass to formic acid and acetic acid with exceptionally high yields. Catal. Today 233, 77–82. doi: 10.1016/j.cattod.2013.12.010
Zhang, X., Zhang, D., Sun, Z., Xue, L., Wang, X., and Jiang, Z. (2016). Highly efficient preparation of HMF from cellulose using temperature-responsive heteropolyacid catalysts in cascade reaction. Appl. Catal. B Environ. 196, 50–56. doi: 10.1016/j.apcatb.2016.05.019
Zhang, Y., Zhao, M., Wang, H., Hu, H., Liu, R., Huang, Z., et al. (2019). Damaged starch derived carbon foam-supported heteropolyacid for catalytic conversion of cellulose: improved catalytic performance and efficient reusability. Bioresour. Technol. 288:121532. doi: 10.1016/j.biortech.2019.121532
Zhao, Q., Wang, H., Zheng, H., Sun, Z., Shi, W., Wang, S., et al. (2013). Acid–base bifunctional hpa nanocatalysts promoting heterogeneous transesterification and esterification reactions. Catal. Sci. Tech. 3, 2204–2209. doi: 10.1039/c3cy20868k
Zheng, W., Cui, Y., Xu, Z., Zhao, L., and Sun, W. (2018). Cellulose transformation into methyl glucosides catalyzed by H3PW12O40: enhancement of ionic liquid pretreatment. Can. J. Chem. Eng. 96, 1250–1255. doi: 10.1002/cjce.23057
Keywords: cellulose hydrolysis, heteropoly acid, biomass conversion, green chemistry, sustainable catalysis
Citation: Luo X, Wu H, Li C, Li Z, Li H, Zhang H, Li Y, Su Y and Yang S (2020) Heteropoly Acid-Based Catalysts for Hydrolytic Depolymerization of Cellulosic Biomass. Front. Chem. 8:580146. doi: 10.3389/fchem.2020.580146
Received: 04 July 2020; Accepted: 17 August 2020;
Published: 25 September 2020.
Edited by:
Svetlana Ivanova, University of Seville, SpainCopyright © 2020 Luo, Wu, Li, Li, Li, Zhang, Li, Su and Yang. This is an open-access article distributed under the terms of the Creative Commons Attribution License (CC BY). The use, distribution or reproduction in other forums is permitted, provided the original author(s) and the copyright owner(s) are credited and that the original publication in this journal is cited, in accordance with accepted academic practice. No use, distribution or reproduction is permitted which does not comply with these terms.
*Correspondence: Song Yang, amh6eC5tc21AZ21haWwuY29t; Hu Li, aGxpMTNAZ3p1LmVkdS5jbg==