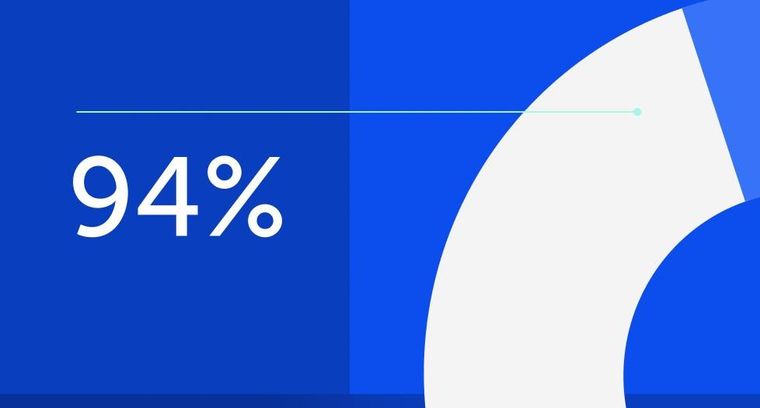
94% of researchers rate our articles as excellent or good
Learn more about the work of our research integrity team to safeguard the quality of each article we publish.
Find out more
ORIGINAL RESEARCH article
Front. Chem., 06 October 2020
Sec. Nanoscience
Volume 8 - 2020 | https://doi.org/10.3389/fchem.2020.00741
This article is part of the Research TopicBioresponsive Nanomaterials for Multimodality Imaging and TherapyView all 10 articles
Molybdenum disulfide (MoS2), a transition metal dichalcogenide material, possesses great potential in biomedical applications such as chemical/biological sensing, drug/gene delivery, bioimaging, phototherapy, and so on. In particular, monolayer MoS2 has more extensive applications because of its superior physical and chemical properties; for example, it has an ultra-high surface area, is easily modified, and has high biodegradability. It is important to prepare advanced monolayer MoS2 with enhanced energy exchange efficiency (EEE) for the development of MoS2-based nanodevices and therapeutic strategies. In this work, a monolayer MoS2 film was first synthesized through a chemical vapor deposition method, and the surface of MoS2 was further modified via a baking process to develop p-type doping of monolayer MoS2 with high EEE, followed by confirmation by X-ray photoelectron spectroscopy and Raman spectroscopy analysis. The morphology, surface roughness, and layer thickness of monolayer MoS2 before and after baking were thoroughly investigated using atomic force microscopy. The results showed that the surface roughness and layer thickness of monolayer MoS2 modified by baking were obviously increased in comparison with MoS2 without baking, indicating that the surface topography of the monolayer MoS2 film was obviously influenced. Moreover, a photoluminescence spectrum study revealed that p-type doping of monolayer MoS2 displayed much greater photoluminescence ability, which was taken as evidence of higher photothermal conversion efficiency. This study not only developed a novel MoS2 with high EEE for future biomedical applications but also demonstrated that a baking process is a promising way to modify the surface of monolayer MoS2.
Two-dimensional materials (2DMs) have attracted extremely wide attention in the biomedical science field because of their various unique properties (Yin et al., 2016; Liu and Liu, 2018). 2DMs are a large family of materials that include semimetals (graphene), semiconductors (molybdenum disulfide (MoS2), black phosphorus, etc.), insulators (h-BN), superconductors (carbon nanotubes), thermoelectric materials (PbTe), and topological insulators (HgTe quantum wells) (Frindt, 1966; Wilson and Yoffe, 1969; Clement et al., 1978; Abruna and Bard, 1982; Mishra et al., 1997; Prasad and Zabinski, 1997; Poizot et al., 2000; Frey et al., 2003; Kane and Mele, 2005). In recent years, another class of 2DMs, transition metal dichalcogenides (TMDCs), have emerged and also received much attention as next-generation applications in electronics and optoelectronics because of their various unique optical, electrochemical, catalytic, and lubrication properties (Radisavljevic et al., 2011a,b; Choi et al., 2012; Wang et al., 2012; Sundaram et al., 2013). The chemical formula of two-dimensional (2D) TMDCs is MX2, where M stands for a transition metal element, such as Mo, W, etc., and X stands for a chalcogen element, such as S, Se, etc. (Wu et al., 2016). TMDCs are a series of compounds with layered structures, and the bulk MX2 is composed of multiple X–M–X layers that are held in stacks by weak van der Waals forces. The single-layer MX2 has a unique sandwich-like structure, with a plane of M atoms wedged into two planes of X atoms. The M and X atoms are held together by strong covalent bonds (Lee et al., 2012). MoS2 is a typical 2D TMDC compound, with the height of each layer being 0.65 nm (Eda et al., 2011). In its bulk structure, MoS2 is a semiconductor with an indirect bandgap of about 1 eV, while monolayer MoS2 has direct bandgap of 1.8 eV (Li and Zhu, 2015). Because of its outstanding properties, MoS2 has been widely studied and used in various applications, including transistors, sensors, and memory and optoelectronic devices in the biomedical sciences (Kim et al., 2012; Singh et al., 2016). Recently, MoS2-based nanoplatforms have been reported as photothermal agents used for cancer therapy and treatment of bacteria-induced infections because of their good biocompatibility and high photothermal conversion efficiency in the near-infrared region (Chou et al., 2013; Yin et al., 2014, 2016; Gao et al., 2018). Chou et al. (2013) synthesized and prepared MoS2 nanosheets that were used in photothermal therapy (PTT) for cancer. The study showed that MoS2 nanosheets had much higher photothermal conversion efficiency than graphene and gold nanorods, thereby improving the therapeutic efficacy in cancer therapy (Chou et al., 2013). Inspired by the effective photothermal conversion of MoS2, Yin et al. (2016) developed polyethylene glycol-functionalized MoS2-based nanoflowers that showed high antimicrobial activity for Gram-negative ampicillin-resistant Escherichia coli and Gram-positive endospore-forming Bacillus subtilis.
Broadly speaking, the methods to obtain MoS2 films include top-down and bottom-up methods, such as micro-mechanical stripping, lithium-ion intercalation, liquid-phase ultrasonic stripping, and chemical vapor deposition (CVD) (Ramakrishna Matte et al., 2010; Coleman et al., 2011; Lee et al., 2012; Baugher et al., 2013; Zhang et al., 2014). The technology of the micro-mechanical stripping method is completely mature; nevertheless, achieving large-scale production using this method remains a challenge (Baugher et al., 2013). The lithium-ion intercalation method is quite time-consuming and has extremely strict requirements for the preparation conditions (Ramakrishna Matte et al., 2010), while the degree and efficiency of the liquid-state ultrasonic stripping method are relatively low and the resulting single-layer product content is low (Coleman et al., 2011). Therefore, it is necessary to optimize large-area deposition methods for MoS2 films to scale up these technologies. In recent years, a method of growing MoS2 by CVD on insulating substrates has been developed. The CVD method is easy to operate and can achieve high-quality, large-area continuous synthesis batches with high efficiency (Zhang et al., 2014); this method is better suited to industrialization and can quickly adapt to the large-scale application of MoS2. In 2012, Lee and co-workers reported the synthesis of large-area monolayers of MoS2 thin films on silicon dioxide substrates by CVD for the first time (Lee et al., 2012). Indeed, a native n-doping behavior of not intentionally doped MoS2 was confirmed by previous investigations (Mak et al., 2013; Fang et al., 2014) because of hypothetical sulfur vacancies (Qiu et al., 2013; Tongay et al., 2013). The n-type doping limits carrier conduction in MoS2 to its conduction bands, and p-type doping is more desirable for MoS2-based field-effect transistor devices (Zhang et al., 2013). Inspired by this demand, research on the doping of MoS2 has been extensively encouraged (Chuang et al., 2016). The p-type doping of MoS2 has lower resistance and better performance than the original MoS2 (Laskar et al., 2014; Neal et al., 2017), the basic mechanism of which is to suppress n-type conductivity. Moreover, 2DMs also enable new methods such as surface charge transfer (Zhang et al., 2016) and intercalation (Jung et al., 2016) to be used. But these methods are neither easy nor convenient. Another commonly used doping method is chemical doping. Rhenium and chlorine are used as substitution donors, while niobium and phosphorus are used as substitution acceptors (Tiong et al., 1999; Laskar et al., 2014; Suh et al., 2014; Yang et al., 2014; Das et al., 2015; Park et al., 2015). The p-type doping of MoS2 films is carried out by fluorine plasma treatment or the spin-on AuCl3 method (Chen et al., 2013; Liu et al., 2016; Xue et al., 2016). In addition, oxygen is frequently used for doping as pure physical adsorption of oxygen will have only a small effect, which can cause an increase in the threshold voltage and a decrease in the on-current; however, the interaction between oxygen and MoS2 films is too weak to overcome the intrinsic n-type doping. In this case, some of the intrinsic properties of MoS2 can be altered by the oxygen plasma method (Nan et al., 2014), which is widely used to prepare p-type doping of MoS2. However, the aforementioned methods of preparing p-type doping of MoS2 have some limitations. (i) There is residual organic solvent after chemical doping. (ii) Plasma treatment is carried out under harsh reaction conditions and requires careful control of the power to decrease damage to the sample surface. (iii) In addition, spin-on AuCl3 is mainly used for achieving high performance in p-type field-effect transistors (Park et al., 2015; Xue et al., 2016).
Therefore, it is of great importance to efficiently and effectively synthesize and prepare p-type doping of MoS2 films to enhance the energy exchange efficiency (EEE) for PTT in biomedical applications. In this work, we report a simple and convenient method for the preparation of p-type doping of monolayer MoS2 through baking under ambient conditions. The monolayer MoS2 film was synthesized using the CVD method first. Optical microscopy (OM) and atomic force microscopy (AFM) were used to directly image and evaluate the morphology associated with monolayer MoS2 grown by CVD onto a SiO2/Si substrate. Then, the monolayer MoS2 film was modified via baking at different temperatures under ambient conditions. Finally, the physicochemical properties of the modified monolayer MoS2 film were thoroughly investigated using AFM, Raman spectroscopy, photoluminescence (PL), and X-ray photoelectron spectroscopy (XPS). This study not only prepared a p-type doping MoS2 film with high EEE, but also demonstrated that the baking process is a promising way to modify the surface of an MoS2 film, which encourages further investigations for biomedical applications.
N-type silicon covered with 30 nm silicon dioxide was purchased from Suzhou Ruicai Semiconductor Co. Ltd. MoO3 (AR) was obtained from Nanjing Muke Nanotechnology Co. Ltd. S powder (99.95 %) was ordered from Aladdin Reagent Co. Ltd. Ar (99.99%) was purchased from Harbin Dawn Gas. Acetone, anhydrous ethanol, and deionized water were obtained from Wuxi Ledong Microelectronics Co. Ltd. The CVD oven was purchased from Hefei Kejing Material Technology Co. Ltd.
MoS2 films were synthesized using the CVD method. N-type silicon covered with 30 nm silicon dioxide was used as the substrate to grow the MoS2 films. A silicon wafer was cleaned through sonication for 10 min with acetone, anhydrous ethanol, and deionized water, respectively. Subsequently, after drying under argon, the silicon wafer was further cleaned by plasma oxygen cleaner. MoO3 and elemental S powder were used as the precursor and reactant materials, respectively, to grow a single layer of MoS2 at a temperature of 700°C under atmospheric pressure. The whole experiment was carried out under Ar. MoS2 films were obtained after 25 min.
Four monolayer MoS2 samples was prepared under the same conditions using the CVD method. One was used as a control sample without any further treatment. The other three were baked under atmosphere in an oven for 2 h, at 150, 200, and 250°C, respectively.
The control sample and the MoS2 sample baked at 250°C were prepared for XPS. Using X-rays to radiate the samples, spectra can be obtained after software processing. XPS was performed to verify whether MoS2 can form an Mo–O bond via baking.
The number of layers of MoS2 films was confirmed by OM. A silicon wafer with MoS2 films was placed on a glass slide, which was put on the objective stage with two clamps to hold it. An appropriate objective was used to observe the MoS2 films with a clear view. The OM image can be displayed by a CCD camera mounted on the OM.
AFM was carried out on MoS2 films on a silicon wafer to characterize the morphology, roughness, and thickness of the samples. An AFM tapping mode (Dimension FastScan, Bruker) was used to scan the samples.
Raman spectroscopy was carried out on the MoS2/Si samples at room temperature with a 532 nm laser as the excitation light source and the laser power was limited to 50 μW to prevent self-heating-induced damage during the measurement. Raman spectra were observed using a micro-confocal Raman spectrometer (Horiba).
In order to determine the luminescence of MoS2 before and after baking, PL was carried on the monolayer MoS2 at different baking temperatures. PL spectra were observed using the same machine as used for the Raman spectra. The only difference was that during Raman measurement the sample was placed directly in the machine at room temperature under air, while during PL measurement the sample was placed in a cryostat.
The monolayer MoS2 film was prepared by the CVD method, as shown in Figure 1A. The n-type silicon substrate covered with 30 nm silicon dioxide was cleaned by plasma oxygen cleaner, followed by the growth of the monolayer MoS2 film using MoO3 and elemental S powder as the precursor and reactant materials, respectively. The reaction was carried out at 700°C at atmospheric pressure under Ar for 25 min. Representative OM images of the as-deposited samples are shown in Figures 1B,C, demonstrating the presence of large MoS2 domains with uniform and smooth morphology, as indicated by the homogeneous purple color on the silicon substrate (Castellanos-Gomez et al., 2010; Li et al., 2012). Some irregular parts of the silicon wafer (orange) were exposed that were not covered with MoS2 film because of the rupture of the monolayer MoS2 film during the CVD process. Collectively, the monolayer MoS2 film was successfully prepared by the CVD method on the silicon substrate.
Figure 1. (A) A schematic of the synthesis of a monolayer MoS2 film using the chemical vapor deposition (CVD) method. (B,C) Optical micrographs of monolayer MoS2 prepared by the CVD method on a silicon substrate. (B) Scale bar, 20 μm. (C) Scale bar, 5 μm.
Next, we treated the monolayer MoS2 film via baking at 150, 200, or 250°C for 2 h under air, followed by imaging through AFM. To further confirm the morphology and size (especially the thickness) of the monolayer MoS2 film, the border of the MoS2 film and wafer was imaged, as shown in Figure 2. The areas with white dots are the silicon wafer, and the integrated and smooth areas are the monolayer MoS2 film. To further characterize the morphology and size of the samples, the perpendicular height (thickness) of the monolayer MoS2 film and roughness of the area indicated by the white square were measured. Figure 2A shows a higher resolution tapping mode AFM morphological image of an MoS2 domain partially covering the SiO2 surface without the baking process. A uniform and smooth MoS2 film was observed, and the result was consistent with that of OM imaging. The thickness and roughness (Rq) were, respectively, confirmed as 0.706 nm and 0.251, which further proved the successful synthesis of the monolayer MoS2 film (Giannazzo et al., 2020). After baking at 150°C (Figure 2B), the thickness and the Rq of the treated MoS2 film were increased to 0.972 nm and 0.310, respectively. Moreover, after baking at 200°C (Figure 2C) and 250°C (Figure 2D), the thickness was obviously increased to 1.225 nm and 1.590 nm, respectively. In addition, the Rq was significantly increased (0.435 at 200°C and 0.978 at 250°C). These results show that the thickness and roughness of the monolayer MoS2 film were increased with an increase in baking temperature. In addition, the surface topography of the sample was also changed with an increase in the baking temperature. Compared with the original sample, the monolayer MoS2 film baked at 150°C still showed a smooth and clear surface, indicating no obvious changes in the surface topography. However, some white dots were observed in the sample after baking at 200°C, and denser and larger white dots appeared on the surface of the sample after baking at 250°C. These changes possibly resulted in the increase in the roughness of the monolayer MoS2 film. As reported previously, the white dots could be the etched parts of the monolayer MoS2 flake, which would break down at 300°C (Zhou et al., 2013). This can be explained by the composition of the surface of the MoS2 film being disturbed or changed, possibly as a result of a chemical reaction that led to the adsorption or formation of elements. In this etching process, some sulfur vacancies might arise, followed by adsorption and reaction with the oxygen in the air and water vapor, finally resulting in p-type doping of the monolayer MoS2 film. In summary, the thickness and surface topography of the monolayer MoS2 film were obviously influenced by the baking process and were dependent on the baking temperature.
Figure 2. Atomic force microscopy images of the monolayer MoS2 film before and after baking at different temperatures for 2 h under air. (A) The original monolayer MoS2 film prepared by the chemical vapor deposition method. The monolayer MoS2 film baked at (B) 150°C, (C) 200°C, and (D) 250°C. The yellow line indicates the surface height of the monolayer MoS2 film. The area indicated with the white square was measured to confirm the roughness. Scale bar, 1 μm.
To further investigate the effect of baking on the monolayer MoS2 film, the Raman spectra of samples before and after baking at different temperatures were measured, as shown in Figure 3. Two characteristic vibrational peaks were observed for the MoS2 film, namely the in-plane vibration mode (385 cm−1) and the out-of-plane vibration mode A1g (403 cm−1) (Figure 3A). The wavenumber difference (Δ = 17.9 cm−1) between the peaks' positions is consistent with the presence of the monolayer MoS2 domains (Kim et al., 2018). Figures 3B,C shows the changes in the frequency shift and full width at half-maximum (FWHM) of two modes with the increase in the baking temperature, respectively. With the increase in the baking temperature, no obvious shift was observed for the mode, whereas the A1g mode showed a right shift with an increase of ~2 cm−1, from about 403 cm−1 to 405 cm−1 (Figures 3A,B). The different changes in the and A1g modes demonstrated the presence of p-type doping of MoS2 (Chakraborty et al., 2012; Mao et al., 2013). Moreover, the FWHM of the A1g mode was obviously decreased with the increase in the baking temperature. However, the mode showed negligible changes compared with those of the A1g mode with the increase in the baking temperature. As reported previously, both the doping and strain showed an effect on the vibrational modes of the Raman spectra of the monolayer MoS2 film (Scalise et al., 2014). If the changes were induced by strain, Raman modes and A1g would shift together, compressive strain could cause blue shift of and A1g, tensile strain could cause red shift of and A1g, therefore there was no strain effect in above results. In contrast, if the changes were caused by doping, the wavenumber and the FWHM of the A1g mode were obviously changed, and the mode was maintained stable. Furthermore, blue shift of the A1g mode and a decrease in the FWHM corresponded to p-type doping, and red shift and an increase in the FWHM corresponded to n-type doping (Chakraborty et al., 2012). In this case, the results revealed that the p-type doping of the MoS2 film was caused by the baking process.
Figure 3. (A) Raman spectra of the samples before and after baking at different temperatures for 2 h under air. The wavelength number (B) and full width at half-maximum (C) of the A1g and modes before and after baking at 150, 200, and 250°C for 2 h under air.
To further confirm the p-type doping of MoS2 after baking, XPS spectra of samples in the Mo(3d) and O(1s) regions before and after baking at 250°C for 2 h under air were monitored, as shown in Figures 4A,B, respectively. First, the peaks at S 2s, Mo4+ 3d5/2, and Mo4+ 3d3/2 were observed (Figure 4A), showing the successful synthesis of the monolayer MoS2 film. After the baking process, the signals of the Mo4+ 3d3/2 and Mo6+ peaks were significantly enhanced (Figure 4A), indicating the generation of MoO3, which proved the p-type doping of MoS2. Furthermore, the signal of the O(1s) peak of the sample after baking was also significantly enhanced in comparison with that of the original sample (Figure 4B), implying p-type doping of MoS2 after the baking process. This can be explained by elemental oxygen being adsorbed on the surface of the sample, followed by reaction with the S vacancy during the baking process. In summary, the results of the XPS spectra further confirmed the presence of p-type doping of MoS2 after the baking process.
Figure 4. X-ray photoelectron spectroscopy (XPS) spectra of the monolayer MoS2 film before and after baking at 250°C for 2 h under air. (A) The XPS spectra of Mo(3d) atoms in the samples before and after baking. (B) The XPS spectra of O(1s) atoms in the samples before and after baking.
Since we successfully synthesized the monolayer MoS2 film and prepared the p-type doping of the MoS2 film, we next evaluated whether the luminescence of MoS2 was enhanced after the baking process using PL measurement (Figure 5). Figure 5A shows the PL spectrum of the monolayer MoS2 film before and after baking at different temperatures. There are two major peaks in the PL spectrum, which correspond to A1 and B1 excitons, respectively. The two peaks are associated with the direct excitonic transitions at the Brillouin zone K point. The energy difference between the two peaks is due to the spin-orbital splitting of the valence band. These PL characteristics are consistent with previous work (Coehoorn et al., 1987a,b; Mak et al., 2010; Splendiani et al., 2010). Therefore, the PL spectra of the samples were divided into two peaks, where (X, Y) represents the nature of the curve after each subpeak. X represents the center of the peak, and Y represents the overall height of the peak as well as the luminous intensity of the sample, as shown in Figures 5B–E. For the first peak in the PL spectra, the PL intensity was enhanced step by step with the increase in the baking temperature. In particular, the PL intensity was obviously enhanced after baking at 250°C, demonstrating a 2.7-fold higher intensity than that of the original sample. The shape of the PL spectrum also changed after baking, becoming sharper with the baking treatment. The first peak energy was right (blue) shifted with an increase in the baking temperature. The right (blue) shift and the enhancement of the PL intensity could be explained by the contributions of the exciton and the charged exciton (trion) in the first peak. As previous works have shown, the charged exciton transformed to an exciton after the baking process, and the exciton was dominant in the first peak, which induced the changes in the PL spectrum. The combination of the two peaks corresponding to the A1 and B1 excitons improved the PL capacity compared with the original sample (Mak et al., 2013; Mouri et al., 2013). In addition, the unique sandwich-like structure (S–Mo–S triatomic layer) also increased the PL ability of MoS2 after baking (Splendiani et al., 2010; Coleman et al., 2014). As mentioned above, during the baking process, the surface of the MoS2 was active for air adsorption, which was manifested in the active physical adsorption of oxygen and water vapor. In addition, Mo–O bonds and MoO3 might be generated by the chemical reactions, facilitating the transformation of the charged exciton to the exciton, thereby leading to the enhanced PL intensity and higher EEE (Wei et al., 2014). In conclusion, these results proved that the p-type doping of MoS2 prepared by the baking process exhibited much higher EEE than the control sample, in which p-type doping of MoS2 might be a promising nanoplatform for PTT in biomedical science.
Figure 5. (A) Photoluminescence (PL) spectra of the monolayer MoS2 film before and after baking at different temperatures. PL peak fitting spectra of the original sample (B) and after baking at 150°C (C), 200°C (D), and 250°C (E).
In this work, we successfully prepared a monolayer MoS2 film using the CVD method. Following this, OM and AFM were used to characterize the morphology of the sample. Furthermore, a baking process was utilized to treat the monolayer MoS2 film at different temperatures under air. The surface topography of the monolayer MoS2 film before and after the baking process was investigated by AFM, especially the thickness and roughness. Subsequently, the Raman and XPS spectra of the samples were used to confirm p-type doping of the monolayer MoS2 film and the mechanism of generation through baking. To further evaluate the energy exchange capacity, the PL of the samples before and after the baking process was measured, and the results showed that p-type doping of the monolayer MoS2 film exhibited much higher EEE than the original control. This study not only developed a monolayer MoS2 film with high EEE that might be a promising platform for PTT or imaging in biomedical applications, but also showed that the baking process could be a convenient method to prepare p-type doping of MoS2 with improved properties by surface modification.
The raw data supporting the conclusions of this article will be made available by the authors. Additional data related to this paper may be requested from the authors.
YW and YM: methodology and investigation. XY: software. JL and HZ: validation. YW and JL: formal analysis. YW: resources. JS: data curation. YW and JS: writing-original draft preparation. CZ: writing-review and editing. YW and XY: visualization. JL, KJ, and CZ: supervision. JL: project administration and funding acquisition. All authors contributed to the article and approved the submitted version.
This work was financially supported by the Beijing Institute of Technology Research Fund Program for Young Scholars and the National Natural Science Foundation of China (no. 21902012).
The authors declare that the research was conducted in the absence of any commercial or financial relationships that could be construed as a potential conflict of interest.
Abruna, H. D., and Bard, A. J. (1982). Semiconductor electrodes. 44. photoelectrochemistry at polycrystalline p-Type WSe2 films. J. Electrochem. Soc. 129, 673–675. doi: 10.1149/1.2123949
Baugher, B. W., Churchill, H. O., Yang, Y., and Jarillo-Herrero, P. (2013). Intrinsic electronic transport properties of high-quality monolayer and bilayer MoS2. Nano Lett. 13, 4212–4216. doi: 10.1021/nl401916s
Castellanos-Gomez, A., Agraït, N., and Rubio-Bollinger, G. (2010). Optical identification of atomically thin dichalcogenide crystals. Appl. Phys. Lett. 96:213116. doi: 10.1063/1.3442495
Chakraborty, B., Bera, A., Muthu, D., Bhowmick, S., Waghmare, U. V., and Sood, A. (2012). Symmetry-dependent phonon renormalization in monolayer MoS2 transistor. Phys. Rev. B 85:161403. doi: 10.1103/PhysRevB.85.161403
Chen, M., Nam, H., Wi, S., Ji, L., Ren, X., Bian, L., et al. (2013). Stable few-layer MoS2 rectifying diodes formed by plasma-assisted doping. Appl. Phys. Lett. 103:142110. doi: 10.1063/1.4824205
Choi, W., Cho, M. Y., Konar, A., Lee, J. H., Cha, G. B., Hong, S. C., et al. (2012). High-detectivity multilayer MoS2 phototransistors with spectral response from ultraviolet to infrared. Adv. Mater. 24, 5832–5836. doi: 10.1002/adma.201201909
Chou, S. S., Kaehr, B., Kim, J., Foley, B. M., De, M., Hopkins, P. E., et al. (2013). Chemically exfoliated MoS2 as near-infrared photothermal agents. Angew. Chem. Int. Edn. 52, 4160–4164. doi: 10.1002/anie.201209229
Chuang, H.-J., Chamlagain, B., Koehler, M., Perera, M. M., Yan, J., Mandrus, D., et al. (2016). Low-resistance 2D/2D ohmic contacts: a universal approach to high-performance WSe2, MoS2, and MoSe2 transistors. Nano Lett. 16, 1896–1902. doi: 10.1021/acs.nanolett.5b05066
Clement, R. P., Davies, W. B., Ford, K. A., Green, M. L., and Jacobson, A. J. (1978). Organometallic intercalates of the layered transition-metal dichalcogenides tantalum sulfide (TaS2) and zirconium sulfide. Inorg. Chem. 17, 2754–2758. doi: 10.1021/ic50188a013
Coehoorn, R., Haas, C., and De Groot, R. (1987a). Electronic structure of MoSe2, MoS2, and WSe2. II. The nature of the optical band gaps. Phys. Rev. B 35:6203. doi: 10.1103/PhysRevB.35.6203
Coehoorn, R., Haas, C., Dijkstra, J., Flipse, C.d, De Groot, R., et al. (1987b). Electronic structure of MoSe2, MoS2, and WSe2. I. Band-structure calculations and photoelectron spectroscopy. Phys. Rev. B 35:6195. doi: 10.1103/PhysRevB.35.6195
Coleman, A. F., Flores, R. L., and Xu, Y.-Q. (2014). Effects of ozone plasma treatment and X-ray irradiation on optical properties of atomically thin molybdenum disulfide. Young Sci. 4:1016. doi: 10.1186/s11671-019-3119-3
Coleman, J. N., Lotya, M., O'Neill, A., Bergin, S. D., King, P. J., Khan, U., et al. (2011). Two-dimensional nanosheets produced by liquid exfoliation of layered materials. Science 331, 568–571. doi: 10.1126/science.1194975
Das, S., Demarteau, M., and Roelofs, A. (2015). Nb-doped single crystalline MoS2 field effect transistor. Appl. Phys. Lett. 106:173506. doi: 10.1063/1.4919565
Eda, G., Yamaguchi, H., Voiry, D., Fujita, T., Chen, M., and Chhowalla, M. (2011). Photoluminescence from chemically exfoliated MoS2. Nano Lett. 11, 5111–5116. doi: 10.1021/nl201874w
Fang, H., Battaglia, C., Carraro, C., Nemsak, S., Ozdol, B., Kang, J. S., et al. (2014). Strong interlayer coupling in van der Waals heterostructures built from single-layer chalcogenides. Proc. Natl. Acad. Sci. U.S.A. 111, 6198–6202. doi: 10.1073/pnas.1405435111
Frey, G. L., Reynolds, K. J., Friend, R. H., Cohen, H., and Feldman, Y. (2003). Solution-processed anodes from layer-structure materials for high-efficiency polymer light-emitting diodes. J. Am. Chem. Soc. 125, 5998–6007. doi: 10.1021/ja020913o
Frindt, R. (1966). Single crystals of MoS2 several molecular layers thick. J. Appl. Phys. 37, 1928–1929. doi: 10.1063/1.1708627
Gao, Q., Zhang, X., Yin, W., Ma, D., Xie, C., Zheng, L., et al. (2018). Functionalized MoS2 nanovehicle with near-infrared laser-mediated nitric oxide release and photothermal activities for advanced bacteria-infected wound therapy. Small 14:1802290. doi: 10.1002/smll.201802290
Giannazzo, F., Bosi, M., Fabbri, F., Schilirò, E., Greco, G., and Roccaforte, F. (2020). Direct probing of grain boundary resistance in chemical vapor deposition-grown monolayer MoS2 by conductive atomic force microscopy. Rapid Res. Lett. 14:1900393. doi: 10.1002/pssr.201900393
Jung, Y., Zhou, Y., and Cha, J. J. (2016). Intercalation in two-dimensional transition metal chalcogenides. Inorgan. Chem. Front. 3, 452–463. doi: 10.1039/C5QI00242G
Kane, C. L., and Mele, E. J. (2005). Quantum spin hall effect in graphene. Phys. Rev. Lett. 95:226801. doi: 10.1103/PhysRevLett.95.226801
Kim, H. J., Kim, D., Jung, S., Bae, M. H., Yun, Y. J., Yi, S. N., et al. (2018). Changes in the Raman spectra of monolayer MoS2 upon thermal annealing. J. Raman Spectrosc. 49, 1938–1944. doi: 10.1002/jrs.5476
Kim, S., Konar, A., Hwang, W.-S., Lee, J. H., Lee, J., Yang, J., et al. (2012). High-mobility and low-power thin-film transistors based on multilayer MoS2 crystals. Nat. Commun. 3:1011. doi: 10.1038/ncomms2018
Laskar, M. R., Nath, D. N., Ma, L., Lee, E. W., Lee, C. H., Kent, T., et al. (2014). p-type doping of MoS2 thin films using Nb. Appl. Phys. Lett. 104:092104. doi: 10.1063/1.4867197
Lee, Y. H., Zhang, X. Q., Zhang, W., Chang, M. T., Lin, C. T., Chang, K. D., et al. (2012). Synthesis of large-area MoS2 atomic layers with chemical vapor deposition. Adv. Mater. 24, 2320–2325. doi: 10.1002/adma.201104798
Li, H., Zhang, Q., Yap, C. C. R., Tay, B. K., Edwin, T. H. T., Olivier, A., et al. (2012). From bulk to monolayer MoS2: evolution of Raman scattering. Adv. Funct. Mater. 22, 1385–1390. doi: 10.1002/adfm.201102111
Li, X., and Zhu, H. (2015). Two-dimensional MoS2: properties, preparation, and applications. J. Materiomics 1, 33–44. doi: 10.1016/j.jmat.2015.03.003
Liu, T., and Liu, Z. (2018). 2D MoS2 nanostructures for biomedical applications. Adv. Healthc. Mater. 7:1701158. doi: 10.1002/adhm.201701158
Liu, X., Qu, D., Ryu, J., Ahmed, F., Yang, Z., Lee, D., et al. (2016). P-type polar transition of chemically doped multilayer MoS2 transistor. Adv.Mater. 28, 2345–2351. doi: 10.1002/adma.201505154
Mak, K. F., He, K., Lee, C., Lee, G. H., Hone, J., Heinz, T. F., et al. (2013). Tightly bound trions in monolayer MoS2. Nat. Mater. 12, 207–211. doi: 10.1038/nmat3505
Mak, K. F., Lee, C., Hone, J., Shan, J., and Heinz, T. F. (2010). Atomically thin MoS2: a new direct-gap semiconductor. Phys. Rev. Lett. 105:136805. doi: 10.1103/PhysRevLett.105.136805
Mao, N., Chen, Y., Liu, D., Zhang, J., and Xie, L. (2013). Solvatochromic effect on the photoluminescence of MoS2 monolayers. Small 9, 1312–1315. doi: 10.1002/smll.201202982
Mishra, S., Satpathy, S., and Jepsen, O. (1997). Electronic structure and thermoelectric properties of bismuth telluride and bismuth selenide. J. Condens. Matter Phys. 9:461. doi: 10.1088/0953-8984/9/2/014
Mouri, S., Miyauchi, Y., and Matsuda, K. (2013). Tunable photoluminescence of monolayer MoS2 via chemical doping. Nano Lett. 13, 5944–5948. doi: 10.1021/nl403036h
Nan, H., Wang, Z., Wang, W., Liang, Z., Lu, Y., Chen, Q., et al. (2014). Strong photoluminescence enhancement of MoS2 through defect engineering and oxygen bonding. ACS Nano 8, 5738–5745. doi: 10.1021/nn500532f
Neal, A. T., Pachter, R., and Mou, S. (2017). P-type conduction in two-dimensional MoS2 via oxygen incorporation. Appl. Phys. Lett. 110:193103. doi: 10.1063/1.4983092
Park, T.-E., Suh, J., Seo, D., Park, J., Lin, D.-Y., Huang, Y.-S., et al. (2015). Hopping conduction in p-type MoS2 near the critical regime of the metal-insulator transition. Appl. Phys. Lett. 107:223107. doi: 10.1063/1.4936571
Poizot, P., Laruelle, S., Grugeon, S., Dupont, L., and Tarascon, J. (2000). Nano-sized transition-metal oxides as negative-electrode materials for lithium-ion batteries. Nature 407:496. doi: 10.1038/35035045
Qiu, H., Xu, T., Wang, Z., Ren, W., Nan, H., Ni, Z., et al. (2013). Hopping transport through defect-induced localized states in molybdenum disulphide. Nat. Commun. 4:2642. doi: 10.1038/ncomms3642
Radisavljevic, B., Radenovic, A., Brivio, J., Giacometti, V., and Kis, A. (2011a). Single-layer MoS2 transistors. Nat. Nanotechnol. 6:147. doi: 10.1038/nnano.2010.279
Radisavljevic, B., Whitwick, M. B., and Kis, A. (2011b). Integrated circuits and logic operations based on single-layer MoS2. ACS Nano 5, 9934–9938. doi: 10.1021/nn203715c
Ramakrishna Matte, H., Gomathi, A., Manna, A. K., Late, D. J., Datta, R., Pati, S. K., et al. (2010). MoS2 and WS2 analogues of graphene. Angew. Chem. Int. Edn. 49, 4059–4062. doi: 10.1002/anie.201000009
Scalise, E., Houssa, M., Pourtois, G., Afanas, V., and Stesmans, A. (2014). First-principles study of strained 2D MoS2. Phys. E Low Dimens. Syst. Nanostruct. 56, 416–421. doi: 10.1016/j.physe.2012.07.029
Singh, V. V., Kaufmann, K., de Ávila, B. E. F., Karshalev, E., and Wang, J. (2016). Molybdenum disulfide-based tubular microengines: toward biomedical applications. Adv. Funct. Mater. 26, 6270–6278. doi: 10.1002/adfm.201602005
Splendiani, A., Sun, L., Zhang, Y., Li, T., Kim, J., Chim, C.-Y., et al. (2010). Emerging photoluminescence in monolayer MoS2. Nano Lett. 10, 1271–1275. doi: 10.1021/nl903868w
Suh, J., Park, T.-E., Lin, D.-Y., Fu, D., Park, J., Jung, H. J., et al. (2014). Doping against the native propensity of MoS2: degenerate hole doping by cation substitution. Nano Lett. 14, 6976–6982. doi: 10.1021/nl503251h
Sundaram, R., Engel, M., Lombardo, A., Krupke, R., Ferrari, A., Avouris, P., et al. (2013). Electroluminescence in single layer MoS2. Nano Lett. 13, 1416–1421. doi: 10.1021/nl400516a
Tiong, K., Liao, P., Ho, C., and Huang, Y. (1999). Growth and characterization of rhenium-doped MoS2 single crystals. J. Cryst. Growth 205, 543–547. doi: 10.1016/S0022-0248(99)00296-1
Tongay, S., Suh, J., Ataca, C., Fan, W., Luce, A., Kang, J. S., et al. (2013). Defects activated photoluminescence in two-dimensional semiconductors: interplay between bound, charged, and free excitons. Sci. Rep. 3:2657. doi: 10.1038/srep02657
Wang, Q. H., Kalantar-Zadeh, K., Kis, A., Coleman, J. N., and Strano, M. S. (2012). Electronics and optoelectronics of two-dimensional transition metal dichalcogenides. Nat. Nanotechnol. 7:699. doi: 10.1038/nnano.2012.193
Wei, X., Yu, Z., Hu, F., Cheng, Y., Yu, L., Wang, X., et al. (2014). Mo-O bond doping and related-defect assisted enhancement of photoluminescence in monolayer MoS2. AIP Adv. 4:123004. doi: 10.1063/1.4897522
Wilson, J. A., and Yoffe, A. (1969). The transition metal dichalcogenides discussion and interpretation of the observed optical, electrical and structural properties. Adv. Phys. 18, 193–335. doi: 10.1080/00018736900101307
Wu, D., Huang, H., Zhu, X., He, Y., Xie, Q., Chen, X., et al. (2016). ERaman mode in thermal strain-fractured CVD-MoS2. Crystals 6:151. doi: 10.3390/cryst6110151
Xue, F., Chen, L., Chen, J., Liu, J., Wang, L., Chen, M., et al. (2016). p-Type MoS2 and n-Type ZnO diode and its performance enhancement by the piezophototronic effect. Adv. Mater. 28, 3391–3398. doi: 10.1002/adma.201506472
Yang, L., Majumdar, K., Liu, H., Du, Y., Wu, H., Hatzistergos, M., et al. (2014). Chloride molecular doping technique on 2D materials: WS2 and MoS2. Nano Lett. 14, 6275–6280. doi: 10.1021/nl502603d
Yin, W., Yan, L., Yu, J., Tian, G., Zhou, L., Zheng, X., et al. (2014). High-throughput synthesis of single-layer MoS2 nanosheets as a near-infrared photothermal-triggered drug delivery for effective cancer therapy. ACS Nano 8, 6922–6933. doi: 10.1021/nn501647j
Yin, W., Yu, J., Lv, F., Yan, L., Zheng, L. R., Gu, Z., et al. (2016). Functionalized nano-MoS2 with peroxidase catalytic and near-infrared photothermal activities for safe and synergetic wound antibacterial applications. ACS Nano 10, 11000–11011. doi: 10.1021/acsnano.6b05810
Zhang, S.-L., Choi, H.-H., Yue, H.-Y., and Yang, W.-C. (2014). Controlled exfoliation of molybdenum disulfide for developing thin film humidity sensor. Curr. Appl. Phys. 14, 264–268. doi: 10.1016/j.cap.2013.11.031
Zhang, X., Shao, Z., Zhang, X., He, Y., and Jie, J. (2016). Surface charge transfer doping of low-dimensional nanostructures toward high-performance nanodevices. Adv. Mater. Weinheim 28, 10409–10442. doi: 10.1002/adma.201601966
Zhang, Y. J., Ye, J. T., Yomogida, Y., Takenobu, T., and Iwasa, Y. (2013). Formation of a stable p-n junction in a liquid-gated MoS2 ambipolar transistor. Nano Lett. 13, 3023–3028. doi: 10.1021/nl400902v
Keywords: MoS2, surface modification, p-type doping, baking, biomedical application
Citation: Wang Y, Ma Y, Shi J, Yan X, Luo J, Zhu H, Jia K, Li J and Zhang CY (2020) Surface Modification of Monolayer MoS2 by Baking for Biomedical Applications. Front. Chem. 8:741. doi: 10.3389/fchem.2020.00741
Received: 29 May 2020; Accepted: 17 July 2020;
Published: 06 October 2020.
Edited by:
Dalong Ni, Shanghai Jiao Tong University, ChinaReviewed by:
Zhimin Wu, Xiangtan University, ChinaCopyright © 2020 Wang, Ma, Shi, Yan, Luo, Zhu, Jia, Li and Zhang. This is an open-access article distributed under the terms of the Creative Commons Attribution License (CC BY). The use, distribution or reproduction in other forums is permitted, provided the original author(s) and the copyright owner(s) are credited and that the original publication in this journal is cited, in accordance with accepted academic practice. No use, distribution or reproduction is permitted which does not comply with these terms.
*Correspondence: Kunpeng Jia, amlha3VucGVuZ0BpbWUuYWMuY24=; Juan Li, amxpQGJpdC5lZHUuY24=; Can Yang Zhang, Y2FueWFuZy56aGFuZ0BzbWFydC5taXQuZWR1
Disclaimer: All claims expressed in this article are solely those of the authors and do not necessarily represent those of their affiliated organizations, or those of the publisher, the editors and the reviewers. Any product that may be evaluated in this article or claim that may be made by its manufacturer is not guaranteed or endorsed by the publisher.
Research integrity at Frontiers
Learn more about the work of our research integrity team to safeguard the quality of each article we publish.