- 1Institute of Advanced Structure Technology, Beijing Institute of Technology, Beijing, China
- 2School of Materials Science and Engineering, Hebei University of Science and Technology, Shijiazhuang, China
SnSe2 nanosheet is a common anode for lithium-ion batteries (LIBs), but its severe agglomeration hinders its practical application. Herein, a three-dimensional (3D) SnSe2 nanoflower (F-SnSe2) composed of non-stacking vertical upward hexagonal nanosheets was prepared through a colloidal method as an anode material for LIBs. Benefiting from the advantages of fast reaction-diffusion kinetics and buffering unavoidable volume variation during cycling, the F-SnSe2 electrode displays remarkable specific capacity of 795 mAh g−1 after 100 cycles at 100 mA g−1 and superior rate performance (282 mAh g−1 at 2,000 mA g−1). This work provides an effective way to get non-stacking nanosheets in energy storage field.
Introduction
In recent years, lithium-ion batteries (LIBs) have been widely employed as an energy storage system for portable electronic devices and electric or hybrid vehicles (EVs) due to their high energy density (Jiang et al., 2019; He et al., 2020). However, current LIBs are still hard to satisfy the stringent demand for security, capacity, and cost (Chu et al., 2019; Wang et al., 2019). The anode material is one of the most crucial components that can determine the battery performance directly. Recently, a variety of electrode materials, such as carbon, alloys, and transition metal chalcogenides (TMDs), have been widely investigated as anode materials for LIBs (Chen K. et al., 2018; Zhang et al., 2019; Li et al., 2020). Typically, as one of the most promising anode candidates, Sn-based materials have attracted great attention due to their high theoretical capacity and abundant resource (Lee and Park, 2017). Besides, Se-based and S-based anodes have been widely investigated for LIBs and sodium ion batteries (NIBs) recently due to their high theoretical capacity, fast ion transportation, and decent redox reversibility (Hu et al., 2019; Han et al., 2020). However, there still remains a great challenge owing to its low electronic conductivity and extremely large volume expansion, resulting in poor cyclic stability (Wei et al., 2018).
SnSe2 as a typical two-dimensional (2D) layered material has attracted intensive attention as a promising anode material for LIBs owing to its high theoretical capacity, tunable spacing structures, and non-toxicity (Du et al., 2016; Huang et al., 2018). However, inherently poor conductivity and high-cost synthesis methods of SnSe2 such as complex reaction routes, high temperature, and some toxic reagents limit practical applications (Chen R. et al., 2018; Ren et al., 2018). One of the most popular approaches is to construct SnSe2 nanomaterials with various morphologies and structure, which can effectively improve ion transport kinetics and reduce unavoidable volume expansion. Therefore, it is necessary to search for an effective method to fabricate functional morphology SnSe2 materials with a good electrochemical performance.
Herein, we report a facile colloidal synthetic method to prepare three-dimensional (3D) flowerlike SnSe2 (F-SnSe2). The as-prepared SnSe2 is composed of non-stacking vertical upward hexagonal nanosheets, which can restrain self-agglomeration during a chemical reaction, improve electron and ion transport, and accommodate volume expansion during lithium-ion intercalation/extraction. When employed as LIB anode, the F-SnSe2 exhibits good lithium-ion storage performance with a high reversible capacity of 795 mAh g−1 after 100 cycles at 100 mA g−1 and superior rate performances, much better than bulk SnSe2 electrode.
Experimental Section
Material Synthesis
F-SnSe2 was synthesized by a simple colloid method. First, 0.2 mmol of stannous chloride was put into a 25-ml single-neck flash along with 10-ml oleylamine (OAm). The mixtures were magnetically stirred for 30 min at 90°C to form a milky suspension. After the solution was cooled down to room temperature, 0.4 mmol dibenzyldiselenide was added into the mixture and then heated to 240°C for 2 h with a heating rate of 8°C min−1. Finally, the obtained black solution was washed with ethanol and cyclohexane several times and vacuum dried at 70°C overnight, and the collected sample was marked as F-SnSe2.
Materials Characterization
X-ray diffraction (XRD) was performed on a Smart-lab using Cu Kα radiation from 10 to 90° at a scan rate of 10° min−1. The field-emission scanning electron microscopy (FESEM, Hitachi SU8010) and transmission electron microscopy (TEM, JEOL JEM-2100F) were used to characterize the morphology of the samples. The atomistic structural information and microtopography were characterized using high-resolution (HR) TEM (JEM-2200FS), selected area electron diffraction (SAED), and equipped with an energy-dispersive spectroscopy (EDS) mapping by high-angle annular dark-field scanning transmission electron microscopy (HAADF-STEM). The specific surface area of samples was degassed for 6 h at 200°C, then nitrogen adsorption was done for 20 h by using the Brunauer–Emmett–Teller (BET) (ASAP 2460) method. Raman spectra (Lab RAM-HR Evolution, 532 nm laser) with a power of 0.2 mW and the exposure time of 1 s and X-ray photoelectron spectroscopy (XPS, AXIS ULTRADLD Scientific) were conducted to reveal the chemical compositions and surface electronic states.
Electrochemical Measurement
The purchased bulk SnSe2 and F-SnSe2 anodes were prepared by pasting a slurry consisting of active material (70 wt%), acetylene black (20 wt%), and polyvinylidene fluoride (PVDF, 10 wt%) in N-methyl-pyrrolidone (NMP) onto a copper foil current collector. After being dried at 80°C in vacuum overnight, they were cut into disks in the diameter of 10 mm with a mass loading of about 1.1 mg cm−2. Then, 2032-type coin cells were assembled in an argon-filled glove box using Whatman GF/D glass fiber filter as a separator, lithium foil as the reference/counter electrode, and electrolyte solution consisted of 1.0 M LiPF6 dissolved in 1:1 volume ratio of ethylene carbonate and diethyl carbonate. A LAND CT2001A multichannel battery tester system was used to measure the Li storage performances within the voltage range of 0.01–2.5 V (vs. Li/Li+). Cyclic voltammetry (CV, at a scan rate of 0.1 mV s−1) tests and the electrochemical impedance spectroscopy (EIS, frequency range of 0.01–100 kHz) were recorded on a CHI 604E electrochemical workstation (Shanghai Chenhua Corp.).
Results and Discussion
Figure 1A shows the XRD pattern of the as-prepared F-SnSe2 which possesses a rhombohedral crystal structure (JCPDS No. 89-3197) (Gurung et al., 2016), and the sharp diffraction peaks reveal the high-crystallinity SnSe2 phase. The morphology and nanostructure of F-SnSe2 were investigated via the field emission scanning electron microscopy (FESEM) and transmission electron microscopy (TEM). Figure 1B shows the SEM image of SnSe2 that presents a uniform nanoflower-like structure, while the purchased bulk SnSe2 shows a multilayer stack structure (Figure S1). Figure 1C shows that the F-SnSe2 nanoflower shows a self-assembly 3D structure with size in the range of 2–3 μm, which is composed of numerous nanosheets. TEM images (Figures 1D,E) illustrate that the size of F-SnSe2 is composed of non-stacking vertical upward hexagonal nanosheets with a size of around 500 nm, which are derived from a preferable growth of SnSe2 along (011) crystal plane (Im et al., 2014). Moreover, the detailed microstructure of F-SnSe2 is further investigated using high-resolution transmission electron microscopy (HRTEM), as shown in Figure 1F. The (101) and (011) crystal planes with lattice spacing of 0.29 nm (Zhang et al., 2016), which is consistent with the corresponding selected area electron diffraction (SAED) pattern (Figure 1G) that shows well-defined spots, suggest a single crystalline nature of the as-grown F-SnSe2. Furthermore, Figures 1H–K display the HAADF-STEM and EDS element mapping of F-SnSe2, confirming that Sn and Se elements are distributed uniformly in the whole nanoflower. As expected, EDS analysis (Figure 1L) of F-SnSe2 demonstrates that the obtained atomic ratio of Sn to Se approaches 1:2.
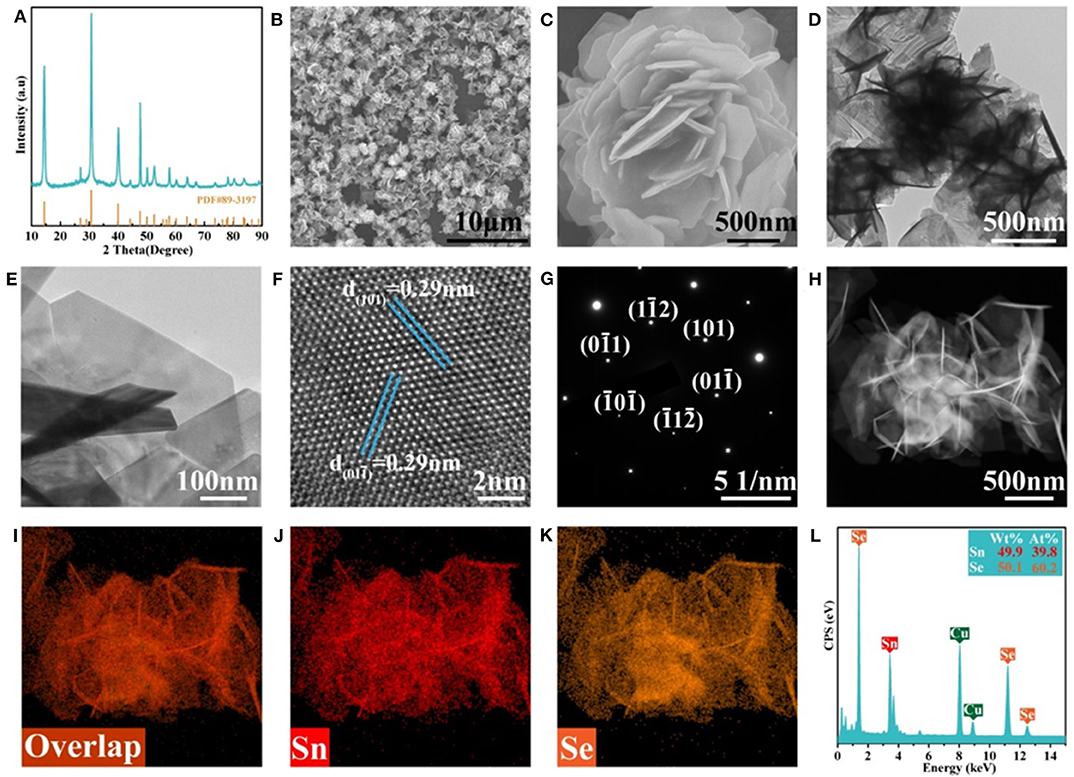
Figure 1. (A) X-ray diffraction (XRD) patterns, (B,C) field-emission scanning electron microscopy (FESEM) images, and (D,E) transmission electron microscopy (TEM) images of F-SnSe2. (F) High-resolution transmission electron microscopy (HRTEM) image of the F-SnSe2 and the corresponding (G) selected area electron diffraction (SAED) pattern. (H) High-angle annular dark-field scanning transmission electron microscopy (HAADF-STEM) image, (I–K) element mapping of Sn and Se and the corresponding (L) energy-dispersive spectroscopy (EDS) spectrum.
Figure 2A exhibits the nitrogen absorption–desorption curve for the F-SnSe2 with type IV isotherms, demonstrating the presence of meso/micropore structures. The corresponding Barrett–Joyner–Halenda (BJH) porosity distribution curve is shown Figure 2B, and the calculated specific pore size distribution of F-SnSe2 is estimated to be 1–10 nm. Raman spectra (Figure 2B) present two characteristic peaks located at 116 and 185 cm−1, corresponding to the Eg and A1g mode of SnSe2, respectively (Chen M. et al., 2018). The surface chemical states of F-SnSe2 were further studied by XPS as shown in Figure S2. Specifically, in the high-resolution XPS spectrum of Sn 3d (Figure 2C), two peaks located at 485.9 and 494 eV are assigned to 3d 5/2 and 3d 3/2 of typical values of Sn4+ ions, respectively (Saha et al., 2016). Correspondingly, two peaks are observed at 53.2 and 54 eV in the Se 3d 5/2 spectrum (Figure 2D), which can be attributed to 3d 5/2 and 3d 3/2 of Se2− in SnSe2 (Zhang et al., 2018).
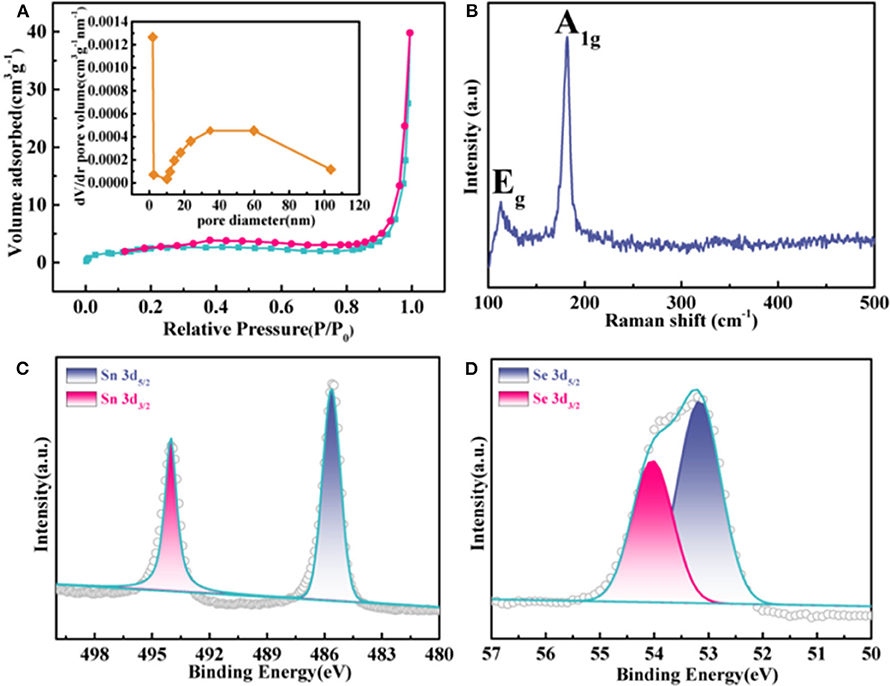
Figure 2. (A) N2 adsorption–desorption isotherms of F-SnSe2; inset is the corresponding pore size distribution curve. (B) Raman spectra and high-resolution X-ray photoelectron spectroscopy (XPS) spectra of (C) Sn 3d and (D) Se 3d in F-SnSe2.
The lithium storage behaviors of F-SnSe2 were evaluated by CV at a scanning rate of 0.1 mV s−1 between 0.01 and 2.5 V (Figure 3A). During the first cathodic scan, two large sharp cathodic peaks at around 1.39 and 0.75 V and some small peaks were observed, which disappear in the subsequent cycles, ascribed to lithium intercalation of SnSe2 interlayers without phase transition and the formation of irreversible solid electrolyte interphase (SEI) film (Du et al., 2016). The cathodic peaks that shift to 2.03, 1.52, and 1.35 V in the following scans can be assigned to the conversion and alloying reactions (Yuan et al., 2018). Conversely, two pairs of delithiation peaks at around 0.52 and 1.18 V in all cycles are almost completely overlapped, implying good reversibility of the F-SnSe2 electrode.
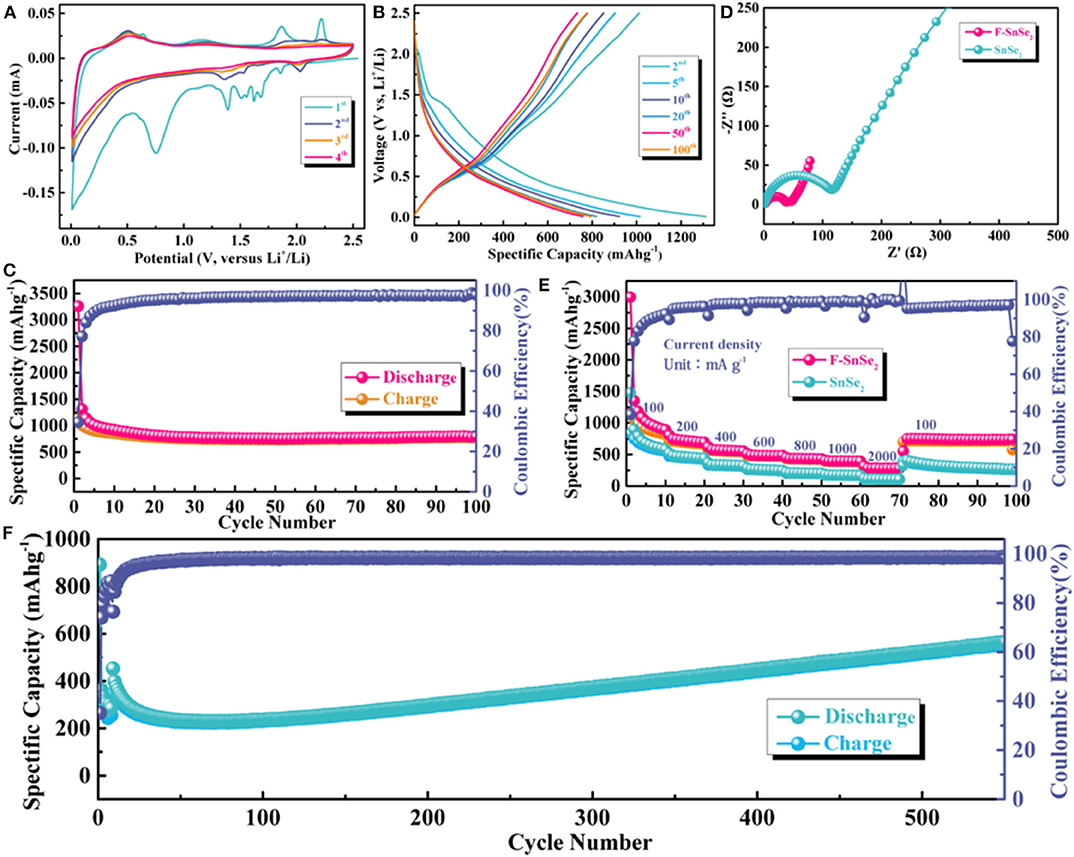
Figure 3. (A) Cyclic voltammogram analysis of F-SnSe2 between 0.01 and 2.5 V at a scan rate of 0.1 mV s−1 for lithium-ion batteries (LIBs). (B) Charge and discharge curves from 2nd to 100th cycles of F-SnSe2 electrode at a current density of 100 mA g−1. (C) Cycling performance of F-SnSe2 electrode at a current density of 100 mA g−1. (D) Comparison of electrochemical impedance spectroscopy plots of bulk SnSe2 and F-SnSe2 electrodes. (E) Comparison of rate capability of bulk SnSe2 and F-SnSe2 electrodes at various current densities from 100 to 2,000 mA g−1, respectively. (F) Long cycling stability of F-SnSe2 electrode at a high current density of 1,000 mA g−1.
Figure S3 and Figure 3B show the galvanostatic charge–discharge profiles of the F-SnSe2 electrode at a current density of 100 mA g−1 from the first cycle to 100 cycles. The F-SnSe2 electrode delivers an extremely high initial capacity (Figure S3), which is attributed to irreversible side reactions with the electrolyte and the formation of the SEI layer on the surface of the F-SnSe2 electrode (Lao et al., 2017). It is worth noting that the initial Coulombic efficiency (CE) should be improved, such as optimizing the electrolyte, pre-lithiation, or compound with carbon matrix to reduce the excess formation of SEI and other irreversible side reactions (Ge et al., 2019; Huang et al., 2020). In addition, the shape of voltage profiles almost overlaps after the initial cycle (Figure 3B), which is consistent with the CV results. As shown in Figure 3C, the F-SnSe2 anode exhibits excellent cyclic stability, which delivers a high reversible discharge specific capacity of 795 mAh g−1 after 100 cycles at 100 mA g−1 with a high CE of nearly 100%, while bulk SnSe2 electrode only exhibits a relatively low capacity of 370 mAh g−1 after 30 cycles (Figure S4).
Furthermore, EIS was performed with F-SnSe2 and bulk SnSe2 anode to investigate the kinetic behavior in LIBs. As indicated in Figure 3D, it can be observed that the semicircle of the F-SnSe2 electrode is much smaller than that of the bulk SnSe2 electrode, which suggests lower charge transfer resistance of the F-SnSe2 electrode (Hong et al., 2019). The improved charge transfer is attributed to 3D flower-like nanostructure of F-SnSe2 that enhances fast ion transfer kinetics. As expected, the rate performance of F-SnSe2 was significantly better than that of bulk SnSe2 (Figure 3E). For the F-SnSe2 electrode, it could deliver invertible capacity of 892, 694, 554, 483, 430, 393, and 282 mA h g−1 at 100, 200, 400, 600, 800, 1,000, and 2,000 mA g−1, respectively. When the current density is switched to 100 mA g−1 again, a reversible capacity of 745 mA h g−1 is recovered. It is necessary to point out that the partial capacity drop in the first 10 cycles for F-SnSe2 electrode can be ascribed to stabilization of the SEI film and activation process as well as some irreversible side reactions (Li et al., 2018; Chu et al., 2019). In contrast, the capacities at 100, 200, 400, 600, 800, 1,000, and 2,000 mA g−1 are 581, 442, 323, 247, 201, 168, and 106 mA h g−1 for bulk SnSe2 electrode, respectively. As shown in Figure 3F, the F-SnSe2 electrode still delivers a high reversible capacity of 611 mA h g−1 after 580 cycles at a high current density of 1,000 mA g−1, demonstrating good long-term cycling stability of F-SnSe2. It is worth mentioning that the capacity fading in the initial 100 cycles can be ascribed to the repetitive volume expansion/contraction that can fracture the SEI layer and expose new active surfaces for SEI growth (Li et al., 2015). Subsequently, high-rate lithiation-induced mechanical degradation can effectively restructure the 3D SnSe2 nanoflower and optimize the SEI, which was defined as reactivation, so the reversible capacity continuously increases during cycling (Qin et al., 2019).
Conclusion
In summary, 3D flower-like SnSe2 has been synthesized via a colloidal method. This nanoflower is composed of non-stacking vertical upward hexagonal SnSe2 nanosheets, which can improve the ion transfer speed and accommodate the volume expansion during cycling. When evaluated as an anode material for LIBs, the F-SnSe2 electrode delivers significantly enhanced electrochemical performance with high capacity and excellent rate performances. A high capacity of 795 mAh g−1 at 100 mA g−1 after 100 cycles and a remarkable rate capability of up to 282 mA h g−1 at 2 A g−1 are obtained. Therefore, this unique structure shows a promising prospect to solve the agglomeration problem.
Data Availability Statement
The raw data supporting the conclusions of this article will be made available by the authors, without undue reservation.
Author Contributions
BW and QY designed the experiments and wrote manuscript. JW and SH performed all the experiments. JH and YL helped in chemistry experiments and their design, and the chemical analysis. BW, QY, and JW helped in discussion, evaluation, and restructuring of the findings. All authors contributed in manuscript editing.
Funding
This work was supported by the National Natural Science Foundation of China (Grant Nos. 11574060, 51974103), the Natural Science Foundation of Hebei Province (E2019208308, E2019208179), the Science Foundation of University of Hebei Province (BJ2019036, ZD2019062), and the Science Research and Development Program of Shijiazhuang (181790411A).
Conflict of Interest
The authors declare that the research was conducted in the absence of any commercial or financial relationships that could be construed as a potential conflict of interest.
Supplementary Material
The Supplementary Material for this article can be found online at: https://www.frontiersin.org/articles/10.3389/fchem.2020.00590/full#supplementary-material
References
Chen, K., Wang, X., Wang, G., Wang, B., Liu, X., Bai, J., et al. (2018). A new generation of high performance anode materials with semiconductor heterojunction structure of SnSe/SnO2@Gr in lithium-ion batteries. Chem. Eng. J. 347, 552–562. doi: 10.1016/j.cej.2018.04.125
Chen, M., Li, Z., Li, W., Shan, C., Li, W., Li, K., et al. (2018). Large-scale synthesis of single-crystalline self-standing SnSe2 nanoplate arrays for wearable gas sensors. Nanotechnology 29:455507. doi: 10.1088/1361-6528/aade32
Chen, R., Li, S., Liu, J., Li, Y., Ma, F., Liang, J., et al. (2018). Hierarchical Cu doped SnSe nanoclusters as high-performance anode for sodium-ion batteries. Electrochim. Acta 282, 973–980. doi: 10.1016/j.electacta.2018.07.035
Chu, J., Wang, W. A., Feng, J., Lao, C. Y., Xi, K., Xing, L., et al. (2019). Deeply nesting zinc sulfide dendrites in tertiary hierarchical structure for potassium ion batteries: enhanced conductivity from interior to exterior. ACS Nano 13, 6906–6916. doi: 10.1021/acsnano.9b01773
Du, C. F., Li, J. R., and Huang, X. Y. (2016). Microwave-assisted ionothermal synthesis of SnSex nanodots: a facile precursor approach towards SnSe2 nanodots/graphene nanocomposites. RSC Adv. 6, 9835–9842. doi: 10.1039/C5RA24500A
Ge, P., Li, S., Xu, L., Zou, K., Gao, X., Cao, X., et al. (2019). Hierarchical hollow-microsphere metal-selenide@carbon composites with rational surface engineering for advanced sodium storage. Adv. Energy Mater. 9:1803035. doi: 10.1002/aenm.201803035
Gurung, A., Naderi, R., Vaagensmith, B., Varnekar, G., Zhou, Z., Elbohy, H., et al. (2016). Tin selenide—multi-walled carbon nanotubes hybrid anodes for high performance lithium-ion batteries. Electrochim. Acta 211, 720–725. doi: 10.1016/j.electacta.2016.06.065
Han, L., Wu, S., Hu, Z., Chen, M., Ding, J., Wang, S., et al. (2020). Hierarchically porous MoS2-carbon hollow rhomboids for superior performance of the anode of sodium-ion batteries. ACS Appl. Mater. Interfaces 12, 10402–10409. doi: 10.1021/acsami.9b21365
He, D., Li, P., Wang, W., Wan, Q., Zhang, J., Xi, K., et al. (2020). Collaborative design of hollow nanocubes, in situ cross-linked binder, and amorphous void@SiOx@C as a three-pronged strategy for ultrastable lithium storage. Small 16, 1–12. doi: 10.1002/smll.201905736
Hong, Y., Mao, W., Hu, Q., Chang, S., Li, D., Zhang, J., et al. (2019). Nitrogen-doped carbon coated SnO2 nanoparticles embedded in a hierarchical porous carbon framework for high-performance lithium-ion battery anodes. J. Power Sources 428, 44–52. doi: 10.1016/j.jpowsour.2019.04.093
Hu, Z., Tai, Z., Liu, Q., Wang, S.-W., Jin, H., Wang, S., et al. (2019). Ultrathin 2D TiS2 nanosheets for high capacity and long-life sodium ion batteries. Adv. Energy Mater. 9:1803210. doi: 10.1002/aenm.201803210
Huang, H., Xu, R., Feng, Y., Zeng, S., Jiang, Y., Wang, H., et al. (2020). Sodium/potassium-ion batteries: boosting the rate capability and cycle life by combining morphology, defect and structure engineering. Adv. Mater. 32:e1904320. doi: 10.1002/adma.201904320
Huang, Z., Liu, B., Kong, D., Wang, Y., and Yang, H. (2018). SnSe2 quantum dot/rGO composite as high performing lithium anode. Energy Storage Mater. 10, 92–101. doi: 10.1016/j.ensm.2017.08.008
Im, H. S., Lim, Y. R., Cho, Y. J., Park, J., Cha, E. H., and Kang, H. S. (2014). Germanium and tin selenide nanocrystals for high-capacity lithium ion batteries: comparative phase conversion of germanium and tin. J. Phys. Chem. C 118, 21884–21888. doi: 10.1021/jp507337c
Jiang, Y., Song, D., Wu, J., Wang, Z., Huang, S., Xu, Y., et al. (2019). Sandwich-like SnS2/graphene/SnS2 with expanded interlayer distance as high-rate lithium/sodium-ion battery anode materials. ACS Nano 13, 9100–9111. doi: 10.1021/acsnano.9b03330
Lao, M., Zhang, Y., Luo, W., Yan, Q., Sun, W., and Dou, S. X. (2017). Alloy-based anode materials toward advanced sodium-ion batteries. Adv. Mater. 29, 1–23. doi: 10.1002/adma.201700622
Lee, D. H., and Park, C. M. (2017). Tin selenides with layered crystal structures for li-ion batteries: interesting phase change mechanisms and outstanding electrochemical behaviors. ACS Appl. Mater. Interfaces 9, 15439–15448. doi: 10.1021/acsami.7b01829
Li, G., Xu, L., Zhai, Y., and Hou, Y. (2015). Fabrication of hierarchical porous MnCo2O4 and CoMn2O4 microspheres composed of polyhedral nanoparticles as promising anodes for long-life LIBs. J. Mater. Chem. A 3, 14298–14306. doi: 10.1039/C5TA03145A
Li, L., Wang, H., Xie, Z., An, C., Jiang, G., and Wang, Y. (2020). 3D graphene-encapsulated nearly monodisperse Fe3O4 nanoparticles as high-performance lithium-ion battery anodes. J. Alloys Compd. 815:152337. doi: 10.1016/j.jallcom.2019.152337
Li, Z., Zhao, H., Lv, P., Zhang, Z., Zhang, Y., Du, Z., et al. (2018). Watermelon-like structured SiOx-TiO2@C nanocomposite as a high-performance lithium-ion battery anode. Adv. Funct. Mater. 28:1605711. doi: 10.1002/adfm.201605711
Qin, M., Zhang, Z., Zhao, Y., Liu, L., Jia, B., Han, K., et al. (2019). Optimization of von mises stress distribution in mesoporous α-Fe2O3/C hollow bowls synergistically boosts gravimetric/volumetric capacity and high-rate stability in alkali-ion batteries. Adv. Funct. Mater. 29:1902822. doi: 10.1002/adfm.201902822
Ren, X., Wang, J., Zhu, D., Li, Q., Tian, W., Wang, L., et al. (2018). Sn-C bonding riveted SnSe nanoplates vertically grown on nitrogen-doped carbon nanobelts for high-performance sodium-ion battery anodes. Nano Energy 54, 322–330. doi: 10.1016/j.nanoen.2018.10.019
Saha, S., Banik, A., and Biswas, K. (2016). Few layer nanosheets of n-type SnSe2. Chem. A Eur. J. 22, 15634–15638. doi: 10.1002/chem.201604161
Wang, W., Huang, H., Wang, B., Qian, C., Li, P., Zhou, J., et al. (2019). A new dual-ion battery based on amorphous carbon. Sci. Bull. 64, 1634–1642. doi: 10.1016/j.scib.2019.08.021
Wei, Z., Wang, L., Zhuo, M., Ni, W., Wang, H., and Ma, J. (2018). Layered tin sulfide and selenide anode materials for Li- and Na-ion batteries. J. Mater. Chem. A 6, 12185–12214. doi: 10.1039/C8TA02695E
Yuan, H., Jin, Y., Lan, J., Liu, Y., Yu, Y., and Yang, X. (2018). In situ synthesized SnSe nanorods in a SnOx@CNF membrane toward high-performance freestanding and binder-free lithium-ion batteries. Inorg. Chem. Front. 5, 932–938. doi: 10.1039/C7QI00762K
Zhang, F., Xia, C., Zhu, J., Ahmed, B., Liang, H., Velusamy, D. B., et al. (2016). SnSe2 2D anodes for advanced sodium ion batteries. Adv. Energy Mater. 6, 1–10. doi: 10.1002/aenm.201601188
Zhang, Y., Xu, Y., Ji, Y., Wang, X., Li, J., Liu, H., et al. (2019). Constructing radially oriented macroporous spheres with central cavities as ultrastable lithium-ion battery anodes. Energy Storage Mater. 17, 242–252. doi: 10.1016/j.ensm.2018.07.011
Keywords: SnSe2, non-stacking, fast ion transfer, nanosheet, lithium-ion battery
Citation: Yu Q, Wang B, Wang J, Hu S, Hu J and Li Y (2020) Flowerlike Tin Diselenide Hexagonal Nanosheets for High-Performance Lithium-Ion Batteries. Front. Chem. 8:590. doi: 10.3389/fchem.2020.00590
Received: 10 April 2020; Accepted: 08 June 2020;
Published: 29 July 2020.
Edited by:
Yuxin Tang, University of Macau, ChinaReviewed by:
Mingzhe Chen, Korea University, South KoreaXifei Li, Xi'an University of Technology, China
Junchao Zheng, Central South University, China
Copyright © 2020 Yu, Wang, Wang, Hu, Hu and Li. This is an open-access article distributed under the terms of the Creative Commons Attribution License (CC BY). The use, distribution or reproduction in other forums is permitted, provided the original author(s) and the copyright owner(s) are credited and that the original publication in this journal is cited, in accordance with accepted academic practice. No use, distribution or reproduction is permitted which does not comply with these terms.
*Correspondence: Bo Wang, d2FuZ2JvMTk5NkBnbWFpbC5jb20=; Ying Li, Yml0bGl5aW5nQGJpdC5lZHUuY24=