- 1Department of Chemical Engineering, Khalifa University, Abu Dhabi, United Arab Emirates
- 2Chemical Engineering Program, Texas A&M University at Qatar, Doha, Qatar
- 3School of Chemistry, Physics and Mechanical Engineering, Faculty of Engineering, Queensland University of Technology, Brisbane, QLD, Australia
- 4Institute of Nanoscience and Nanotechnology, National Centre of Scientific Research “Demokritos”, Attica, Greece
- 5Center for Catalysis and Separations (CeCaS), Khalifa University, Abu Dhabi, United Arab Emirates
- 6ADNOC Gas Processing, Department of Research and Engineering R&D, Abu Dhabi, United Arab Emirates
- 7Research and Innovation Center on CO2 and H2 (RICH), Khalifa University, Abu Dhabi, United Arab Emirates
- 8Center for Membranes and Advanced Water Technology (CMAT), Khalifa University, Abu Dhabi, United Arab Emirates
Gas separation and purification using polymeric membranes is a promising technology that constitutes an energy-efficient and eco-friendly process for large scale integration. However, pristine polymeric membranes typically suffer from the trade-off between permeability and selectivity represented by the Robeson's upper bound. Mixed matrix membranes (MMMs) synthesized by the addition of porous nano-fillers into polymer matrices, can enable a simultaneous increase in selectivity and permeability. Among the various porous fillers, metal-organic frameworks (MOFs) are recognized in recent days as a promising filler material for the fabrication of MMMs. In this article, we review representative examples of MMMs prepared by dispersion of MOFs into polymer matrices or by deposition on the surface of polymeric membranes. Addition of MOFs into other continuous phases, such as ionic liquids, are also included. CO2 separation from hydrocarbons, H2, N2, and the like is emphasized. Hybrid fillers based on composites of MOFs with other nanomaterials, e.g., of MOF/GO, MOF/CNTs, and functionalized MOFs, are also presented and discussed. Synergetic effects and the result of interactions between filler/matrix and filler/filler are reviewed, and the impact of filler and matrix types and compositions, filler loading, surface area, porosity, pore sizes, and surface functionalities on tuning permeability are discoursed. Finally, selectivity, thermal, chemical, and mechanical stability of the resulting MMMs are analyzed. The review concludes with a perspective of up-scaling of such systems for CO2 separation, including an overview of the most promising MMM systems.
Introduction
Nowadays, large amounts of CO2 are emitted to the atmosphere associated with fossil fuel consumption to fulfill the energy demands for power generation, transportation, industry needs, and other anthropogenic activities, which are generally accepted as the leading cause of climate change and global warming. In the Paris agreement, it was agreed that the temperature rise should be restricted below 2°C, preferably as low as 1.5°C, to limit the detrimental effects of climate change. To reach this goal, society should strive to lower global CO2 emissions to near zero by 2050 (Hawes, 2019). One of the ways to reduce these discharge is carbon capture utilization and storage (CCUS), which is still regarded as relatively costly, in particular, due to the cost associated with the capture step. The current CO2 capturing at large scale, e.g., from post-combustion mixtures, takes place mostly via regenerative absorption of CO2 using solvents such as alkanol amines, which is the primary process of separation of CO2 and other contaminants from sources such as natural gas and synthesis gas (Kohl and Nielsen, 1997). Natural gas also often contains other unwanted gas impurities such as hydrogen sulfide (H2S), nitrogen (N2) etc., which diminish the NG quality, cause corrosion of process units, and result in environmental issues (Abdulrahman and Sebastine, 2013). These components are almost indeed exclusively removed with absorption using alkanol amine solutions for onshore operations.
On the other hand, off-shore operations, particularly in the far East, use membrane technology for the removal of CO2 and other impurities from natural gas due to the space and weight limitations of the off-shore sites. In general, for the two applications of natural gas treatment and post-combustion capture, significant research efforts are focusing on improving the existing technologies and developing new routes for CO2 removal. Examples of such efforts focus on improved alkanolamines absorption schemes (Vinoba et al., 2013), adsorption (Bhagiyalakshmi et al., 2011; Kueh et al., 2018), chemisorption (Wang et al., 2005; Qaroush et al., 2017), membrane technology (Luis et al., 2012; Adewole et al., 2013; Veziri et al., 2015), cryogenic (Maqsood et al., 2014; Surmi, 2019), and enzymatic (Pierre, 2012; Gundersen et al., 2014) processing.
Both polymeric and inorganic membranes have been used in various industrial applications such as gas separation, microfiltration, reverse osmosis (RO), water purification, heavy metal removal, hemodialysis etc. Indeed, polymeric and inorganic membranes constitute a broad research field for many applications in separation technology. Specifically, for gas separation, the permeance and selectivity coefficients are the main properties that dictate their efficiency and suitability for a specific process. At the same time, porosity, thermal resistance, swelling, and electrical conductivity also play an important role and, depending on the intended application, must be optimized (Labropoulos et al., 2009; Pilatos et al., 2010; Favvas and Papageorgiou, 2013). The advantages of the polymeric membranes include excellent stability, high efficiency, low energy requirement, and ease of operation. Due to these advantages, along with the progress made through R&D activities in academia and industry, several membrane systems have been implemented worldwide over the last decades. Nonetheless, the development of membranes with excellent thermal and mechanical stability combined with good permeability/selectivity properties are essential for industrial integration (Majeed et al., 2012; Stoeger et al., 2012a; Labropoulos et al., 2015).
Membrane technology has intrinsic advantages over the conventional absorption processes due to drawbacks associated with the latter, such as complexity of the process lay-out, corrosivity, and the costly and energy-consuming regeneration (Vinoba et al., 2017). However, a main drawback of polymeric membranes is to withstand higher temperatures, as the latter can be involved in a gas separation process. Recently, thermally stable polymers have received considerable attention in the development of gas separation membranes with durability and process sustainability. For instance, heterocyclic groups incorporated in aromatic polymeric membranes can possess higher thermal and mechanical stability combined with high gas permeance values (Rezakazemi et al., 2018). Membranes have the advantage that at high partial pressure, the flux scales with the concentration. At the same time, amines will get saturated and will require an increasing amount of solvent flow, leading to increased capital and operating cost. Therefore, membrane technology has received promising attention in various industries toward effective CO2 separation (Hamid and Jeong, 2018), and it is also potential candidate for dual conversion and capture capability (Perdikaki et al., 2012, 2016). It is worth noting that the Norwegian Government together with Equinor, Shell, and Total started the Northern Lights project for developing an “open source” service for transport and storage of European CO2 where the separation activities will play a significant role in the overall objectives (Syversen, 2019).
Generally, inorganic polycrystalline membranes and organic membranes are employed for the CO2 separation process, and both systems present advantages but also limitations. Inorganic membranes possess high chemical, thermal, and mechanical stability and long-term durability, and they also exhibit high permeability due to their textural features as compared to polymeric membranes (Pera-Titus, 2014). On the other hand, membrane processability, high cost, brittleness and defects, and scale-up potential, often limit the commercialization of inorganic membranes (Stoeger et al., 2012b; Nasir et al., 2013; Alomair et al., 2015).
Nowadays, the natural gas industry utilizes polymeric membranes for CO2 separation due to their inherent flexibility, cost-effectiveness, easy processability, and high durability (Powell and Qiao, 2006; Kim and Lee, 2013). The separation mechanism of polymeric membranes varies based on glassy and rubber content of the polymer matrix. Polymeric membranes with high glassy content separate based on molecular size mainly using diffusion principles (Wang S. et al., 2016), whereas polymeric membranes with high rubber content separate CO2 via the condensability mechanism (Freeman, 1999). Hence, membrane selectivity and permeability highly depend on the nature of the polymer. Overall, membranes with high glassy content show better CO2 selectivity and permeability (Kapantaidakis et al., 1999). However, the polymeric single-phase membranes have the limitation of the permeability-selectivity trade-off issue as depicted in the Robeson plot (Maier, 1998; Robeson, 2008). As a result, significant research has focused on circumventing the “trade-off” problem in the traditional polymeric membranes. Currently, membrane technology development focuses more on the incorporation of inorganic particulates to yield filled hybrid polymer composites membranes, termed as mixed matrix membranes (MMMs) (Chung et al., 2007; Galizia et al., 2017; He et al., 2018). MMMs are composed of homogeneously interpenetrating polymeric and inorganic particles. Specifically, the incorporation of many types of nanomaterials into the membrane's matrices, which often is a complex process, can provide unique properties to the membrane's structure and affect gas transport behavior (Goh et al., 2011). Generally, the most common dispersed inorganic particles in polymer matrices are metal oxides nanoparticles (e.g., MgO and TiO2) (Sotto et al., 2011), ZnO (Balta et al., 2012), Al2O3 (Yu et al., 2011), Au (Vanherck et al., 2011), zero-valent iron (ZVI) (Xu and Bhattacharyya, 2005), Pd (Pacheco Tanaka et al., 2006), magnetic iron-based nanoparticles (Favvas et al., 2019), as well as zeolites (Varoon et al., 2011), carbon molecular sieves (Vu et al., 2003), silicate materials (Zornoza et al., 2011b), non-porous silica (Sanchez et al., 2005), porous silica (Zhang et al., 2008), both multi-walled (MWCNTs) (Favvas et al., 2014), and single-walled (SWCNTs) carbon nanotubes (Das et al., 2014), clays (Zulhairun et al., 2014), and metal-organic frameworks (MOFs) (Rodenas et al., 2014).
MMMs are the alternative to traditional polymeric membranes, as they could rectify the drawback of the “trade-off” effect in commercial membranes. Several factors, such as type and physicochemical properties of fillers, nature of polymer matrix, filler-matrix interaction, processing methods, etc., influence the performance of the MMMs in the CO2 separation processes. In particular, the selection of filler material is one of the most crucial parameters for designing efficient MMMs. An appropriate filler selection is challenging because filler materials might exhibit a weak adhesion in the polymer matrix, thus leading to poor CO2 separation performance (Dong et al., 2013). Table 1 displays recent developments in the fabrication of MMMs using different porous filler particles exhibiting unique textural and surface properties from porous families such as zeolites (Bastani et al., 2013; Zarshenas et al., 2016), mesoporous silica (Zornoza et al., 2011b; Li et al., 2015), graphene oxide (GO) (Li et al., 2015; He et al., 2019), CNTs (Bakhtin et al., 2015; Dai et al., 2019), and MOFs (Safak Boroglu and Yumru, 2017; Etxeberria-Benavides et al., 2018). Among these fillers, MOFs have received considerable attention, and numerous MOFs and their hybrids have been utilized as the dispersive fillers to fabricate efficient MMMs for CO2 separation. This review provides insights into the role and advantages of MOFs as well as hybrid and functionalized MOFs in MMMs for CO2 separation.
Significance of MOFs in MMMs
Currently, MMMs are effectively contributing to membrane technology for CO2 removal due to their flexibility and multifunctionality. These composite membranes are developed by incorporation of different types of inorganic fillers (porous and non-porous) into the polymer matrices mainly through solution blending, in situ polymerization, and sol-gel processing (Cong et al., 2007). The dispersed fillers can change the diffusion pathway, free volume, and mechanical and thermal properties of the polymer matrix. MMMs containing non-porous fillers typically provide only selective adsorption of particular molecular species, whereas, porous fillers can directly change the MMMs permeability and selectivity based on their porous network within the membrane (Goh et al., 2011; Zhang et al., 2013; Salehi Maleh and Raisi, 2019). A small fraction of inorganic nanofillers can effectively interact with the polymer matrix, e.g., through hydrogen bonding, Van der Waals or covalent bond interactions, which also enhance the chemical, mechanical and thermal stability of the polymeric membranes (Siddique et al., 2014). Combining organic polymers with inorganic fillers can yield MMMs exhibiting significant enhancement of CO2 separation, and it can also effectively help overcome the “trade-off” issues existing in pure polymeric or inorganic membranes (Althues et al., 2007; Mubashir et al., 2018a). Nonetheless, the filler dispersion in the polymer matrix plays a predominant role to acquire defect-free MMMs. Besides, the chemical structure, surface functional groups, aspect ratio, and textural properties are essential factors for the proper dispersion of the filler materials. It is worth noting that surface-modified inorganic fillers lead to a robust interfacial adhesion with the polymer matrix, resulting in effective dispersion and formation of defect-free MMMs (Guo et al., 2015). Though both zeolites and MOFs are actively used as dispersive fillers, the advantages of MOFs include higher porosity, availability in various sizes and shapes, and presence of the organic part which enhances compatibility with the polymer matrix.
MOFs have gained significant interest in various fields, including catalysis, separation, purification, biomedicine, sensors, microelectronics, etc. (Keskin and Kizilel, 2011; Falcaro et al., 2014; Mubashir et al., 2018b; Wang B. et al., 2018). MOFs consist of metal ions or metal-oxide clusters combined with organic ligands, and are highly porous, often hierarchical, chemically flexible, while they can possess multiple chemical architectures (Li Z. et al., 2019; Safaei et al., 2019). MOFs have been recognized as attractive filler materials for the development of MMMs owing to their inherent three-dimensional (3D) coordination network with effective porosity and good interaction with the continuous polymer phase (Cheng et al., 2019). Manipulating the organic ligands and the surface of the MOFs with suitable chemical functionalities gives rise to the formation of microvoids between the organic and inorganic phases and minimizes the selectivity loss (Ordoñez et al., 2010; Dai et al., 2012; Sorribas et al., 2014). Compared to MOFs, other dispersive fillers often have limited chemical flexibility and mutability, which may restrain their dispersion within the polymer matrix. In addition, the MOF frameworks are chemically flexible. Thus they can contribute to selective adsorption of certain molecular species from the gaseous mixture and enhance the separation performance of the membranes (Dechnik et al., 2017). Based on the type and structure of the polymer matrix, a wide range of MOFs are available to be used as fillers with a variety of organic functionalities and chemical flexibility of the organic part of the framework. Besides, suitable post-synthetic modification of MOFs can significantly enhance the polymer-MOF interfacial interaction and increase the separation efficiency (Katayama et al., 2019). Recently, MMMs have also been developed in situ by growing MOFs within the polymer matrix followed by curing of the latter. The as-prepared MMMs often show high separation efficiency as compared to the post-synthesis MMMs (Marti et al., 2018). In addition, MOFs are easily hybridized with other porous filler materials such as CNTs, GO, and mesoporous silica, owing to their intrinsic chemical functionality, which provides a synergetic effect with the other fillers within the polymer matrix (Pilatos et al., 2014; Lin et al., 2015; Naik et al., 2016; Anastasiou et al., 2018; Pokhrel et al., 2018b). Most MOF fillers exhibit higher porosity than zeolites and other porous materials. They can be more compatible with polymer matrices, while tuning of their textural properties can also offer tunability for permeability and selectivity in the resulting MMMs (Janiak and Vieth, 2010).
MOF Based MMMs for CO2 Separation
Pristine MOF—Based MMMs
Numerous MOFs have been developed and studied for various applications (Furukawa et al., 2013), however, only a rather small portion of those as of now, such as HKUST-1, zeolitic imidazole frameworks (ZIFs), MILs, MOF-74, and UiO-66, have been investigated as filler for MMMs. These MOFs are ideal materials for enhancing the membrane performance due to their tunable porosity, gas selectivity and permeability, solubility and diffusivity, and strong interactions with the polymer matrix as to yield defect-free MOF-based MMMs (Erucar et al., 2013; Chen K. et al., 2018; Fan L. et al., 2018; Nabais et al., 2018; Zhao Y. et al., 2019). Nabais et al. fabricated MMMs using Fe(BTC) and Matrimid®5218 for CO2 separation (Nabais et al., 2018). The filler loadings were varied from 0 to 30 wt. % and resulted in enhancing thermal stability and hydrophobicity of the MMMs. Generally, the CO2 permeability increases with the addition of MOF, yet the extent of the permeability increase depends on the nature of MOFs and the degree of functionalization. Contrary to the behavior of other membranes, the selectivity of the MMMs significantly increased with increasing the temperature from 29 to 78°C in that work (Table 2). This is attributed to the higher mobility of the polymeric chains at the higher temperatures and increased free volume that enhance gas diffusivity (Ilyas et al., 2017). Moreover, the MMMs containing 10 and 30 wt. % Fe(BTC) demonstrated better performance in CO2/N2 separation as compared to other commercial membranes.
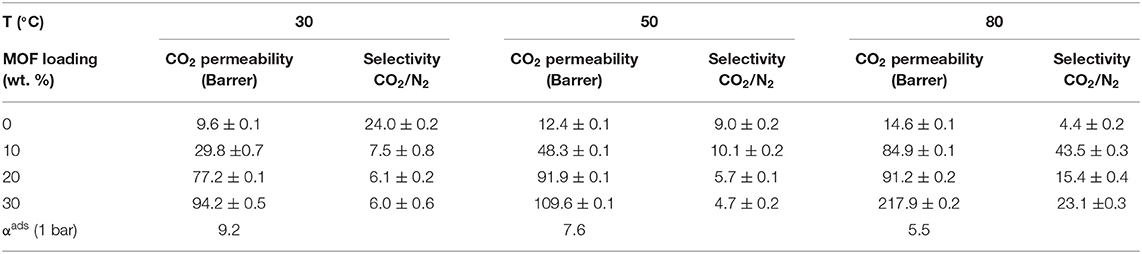
Table 2. Effect of temperature on the CO2 permeability and CO2/N2 ideal selectivity of the Matrimid®5218 based MMMs consisting of 10, 20, and 30 wt. % of Fe (BTC) (Nabais et al., 2018).
Zhao et al. fabricated MMMs using NH2-CAU-1 with polyimide matrix-supported on porous ceramic membrane via solvent casting, and the prepared MMMs demonstrated excellent separation performance in terms of permeation rate and selectivity. 20 wt. % NH2-CAU-1 was the optimum loading as revealed by the significant enhancement in the H2/CO2 selectivity compared to the selectivity of 6.5 for the pristine membrane (Zhao Y. et al., 2019). In another study, Fan et al. incorporated two types of MOFs, i.e., Ni2(L-asp)2bipy and Ni2(L-asp)2pz with different pore sizes into poly(ether-block-amide) (Pebax-1657) and the MMM consisting of 20 wt. % of Ni2(L-asp)2bipy showed maximum CO2 permeability of 120 Barrer compared to the pristine membrane (55 Barrer) (Fan L. et al., 2018). Chen et al. also reported enhancement in the performance of MMMs using KAUST-7 as the nano-filler with narrow size distribution prepared by co-solvent synthesis (Chen K. et al., 2018). Both the CO2/CH4 selectivity and CO2 permeability increased with increasing filler content in the MMM (Figure 1A). The improved performance of the MMMs is partially attributed to the strong interfacial interaction between the filler and the polymer, as can be seen in the SEM images in Figure 1B, where a circular network morphology is recognized (Chen K. et al., 2018).
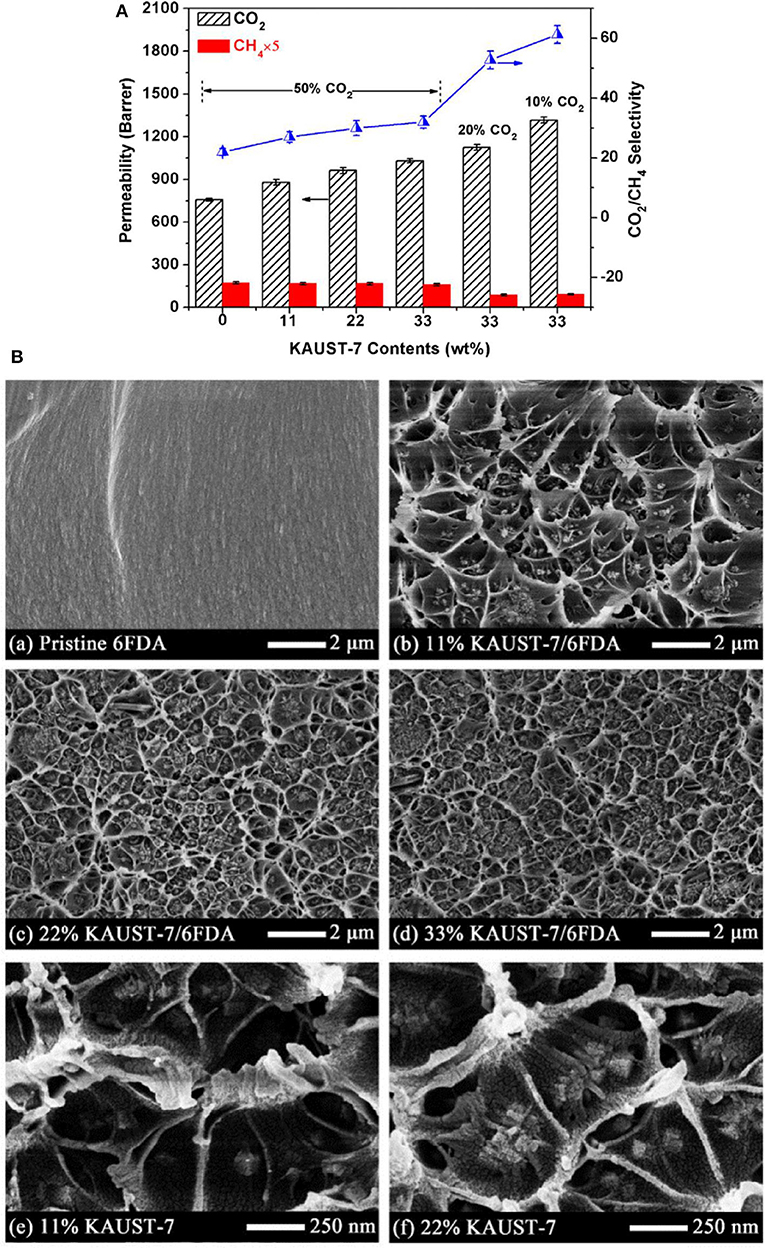
Figure 1. (A) CO2 separation efficiency of 6FDA-Durene based MMMs with various KAUST-7 loadings at 35°C and 2 bar (% is CO2 concentrations in the feed gas). (B) SEM cross-section images of pristine 6FDA membrane and 6FDA based MMMs with wt. % contents of KAUST-7 nanocrystals (~80 nm). Reproduced from Chen K. et al. (2018) with permission from Elsevier.
Cheng et al. prepared ultrathin MMMs using copper 1,4-benzenedicarboxylate nanosheets (CuBDC) and polymers of intrinsic microporosity (PIMs) via spin coating, and they investigated the impact of the membrane thickness and CuBDC loadings on the performance of the MMMs. An optimum filler loading of 10 wt. % of CuBDC comprising MMM with a thickness of 660 nm showed CO2/CH4 selectivity of 15.6 and CO2 permeance of 407 GPU. Furthermore, the stability of the membranes was also studied by carrying out analysis for 100 h, confirming the high stability of the prepared MMM (Cheng et al., 2017). Chi et al. expanded the study of MMMs based on multidimensional MOFs to synergize the advantages of different filler dimensions. A mechanism to form a multidimensional HKUST-1 filler was proposed, based on using a modulator to adjust the MOF nucleation and growth. As a result, a 2.5-fold increase in CO2 permeability was obtained with the MMM containing 30 wt. % of the multidimensional MOF compared to that of the pristine polymer (Chi et al., 2019). Table 3 compares the CO2 separation performance of the above mentioned pristine MOF—based MMMs. MMMs based on hierarchical fillers such as zeolitic type MOF have also received significant attention due to their separation efficiency (Sridhar et al., 2007; MacDowell et al., 2010; Rezakazemi et al., 2014; Alqaheem et al., 2017; Vinoba et al., 2017). In general, several targeted functional groups such as dimers, trimers, tetramers or polyhedral chains can be easily incorporated into MOFs as functional linkers that can be further modified with various organic groups to control the final properties. In general, molecular diffusion mechanism depends on the size of the gas molecule. Thus, the pore diameter is a crucial factor for CO2 separation (Mao and Sinnott, 2001; Sridhar et al., 2007; Du et al., 2012; Alqaheem et al., 2017). Figure 2A shows the relationship between the pore diameter of fillers and various gas molecules for their efficient separation. In addition, different types of MOF fillers together with their respective characteristic pore sizes are shown in Figure 2B. Critical factors such as porosity, pore size, tortuosity, particle size, affinity of MOF fillers with the gas molecules and the matrix, MOF type, geometry, functional groups, and percentage loading of filler influence the transport behavior through the porous membranes (Aykac Ozen and Ozturk, 2019). For instance, small pore sizes of the MOF fillers can selectively allow small molecules to permeate, such as H2, He, and CO2 with kinetic diameters of 2.6, 2.89, and 3.3 Å, respectively, while larger pore size of MOFs can allow transport of larges gas molecule like CH4 (kinetic diameter = 3.8 Å), thus resulting in tailored selectivity (Cheng et al., 2017).
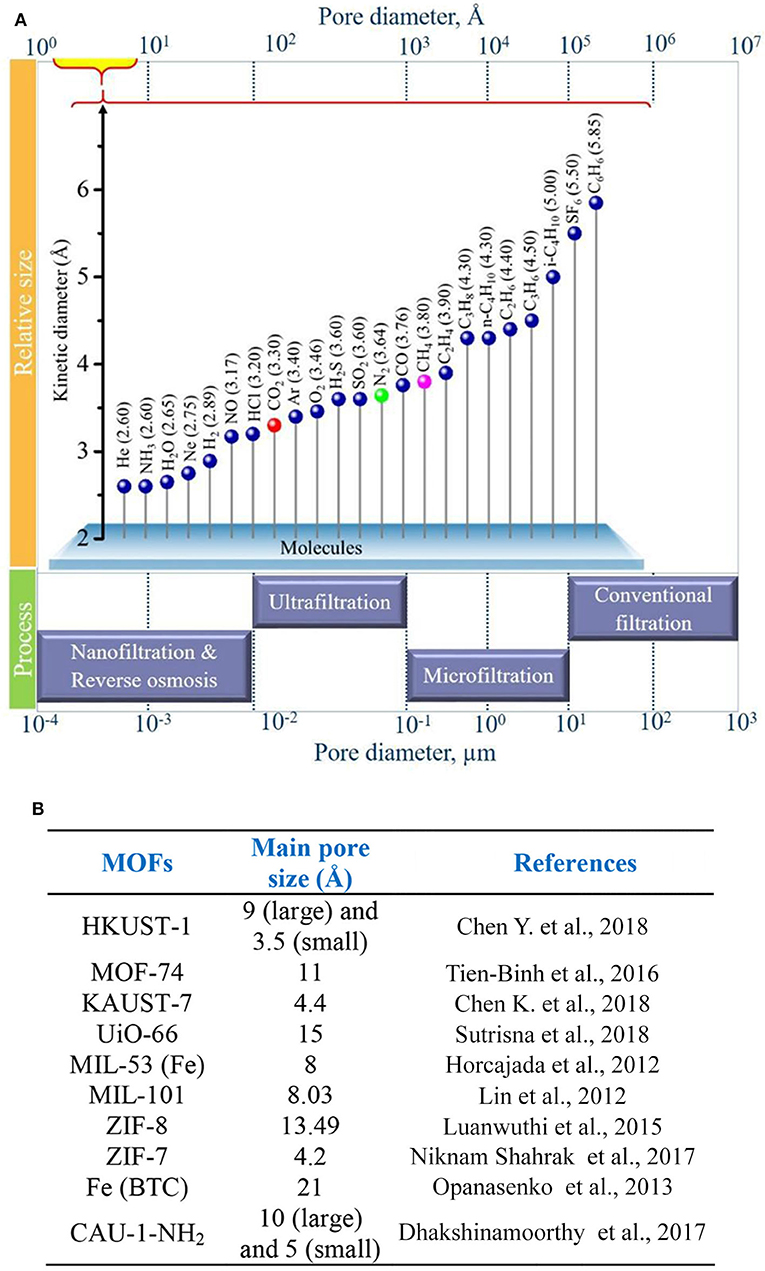
Figure 2. (A) Relationships between pore diameter of fillers and kinetic diameter of gas molecules for different separation processes. Reproduced from Vinoba et al. (2017) with permission from Elsevier. (B) Types of MOFs with respective critical pore sizes.
UiO-66 Based MMMs
UiO-66 constitutes a zirconium-based framework bearing carboxylate linkers and exhibits high thermal stability (up to 550°C) and excellent chemical stability toward the water and organic solvents (Moghaddam et al., 2018). UiO-66 with amine (UiO-66, UiO-66-NH2), and carboxylic (UiO-66-(COOH)2) functional groups (Figure 3) were prepared by Sutrisna et al. and utilized as fillers for the fabrication of MMMs (Sutrisna et al., 2018). The amine-functionalized UiO-66 significantly improved the perm-selectivity of the MMMs. Figure 3 shows SEM images of the cross-sectional area of different MMMs prepared and the effect of the various coating layers from a microscopic point of view. Poly [1-(trimethylsilyl) prop-1-yne] (PTMSP) possessed a high perm-selectivity value. It was used as a smooth gutter layer, which prevented the intrusion of PEBAX into the supportive membrane pores, forming a thin and continuous selective layer. Moreover, the incorporation of UiO-66 resulted in higher stability of the resulting MMMs under high pressure.
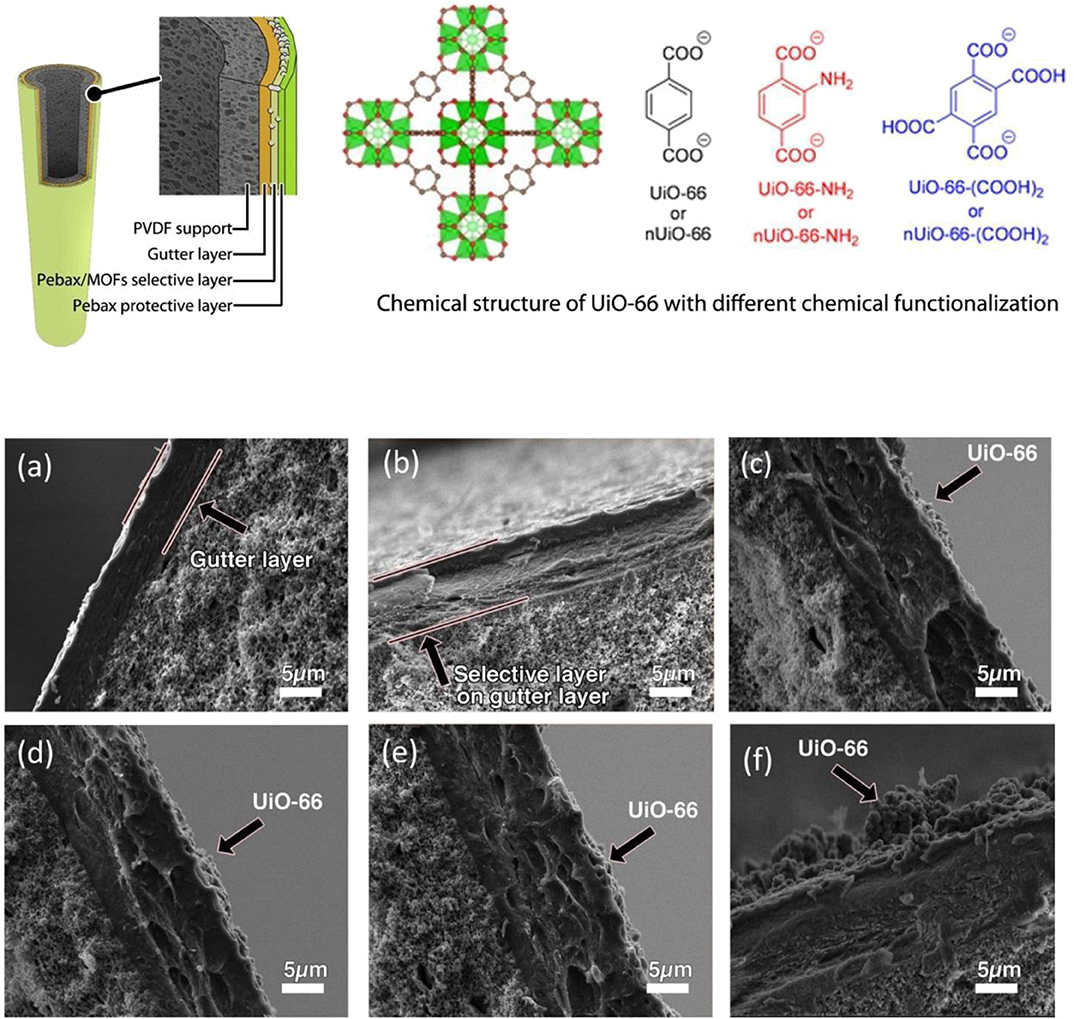
Figure 3. Schematic representation of a UiO-66 MMM and of different chemical functionalization schemes for UiO-66, and effect of different coating layers on the membranes: (a) PTMSP, (b) PTMSP and pure Pebax, (c) PTMSP and 50 wt. % UiO-66 in Pebax, (d) PTMSP and 50 wt. % UiO-66-NH2 in Pebax, (e) PTMSP and 50 wt. % UiO-66-(COOH)2 in Pebax and (f) PTMSP and 80 wt. % UiO-66 in Pebax. Membranes (b–f) have an extra top protective layer. Reproduced from Sutrisna et al. (2018) with permission from RSC.
Other researchers study the use of hydroxylated (-OH), amino (-NH2), nitro (-NO2), and methoxy (-OMe) functionalized UiO-66 structures to investigate the effect of a polar and basic functional group on CO2 separation. Functionalized UiO-66 to exhibit polar surfaces have been shown to improve CO2 adsorption and are attractive for increasing CO2/CH4 and CO2/N2 selectivity (Cmarik et al., 2012; Biswas and Van Der Voort, 2013; Wu et al., 2013). Smith et al. modified UiO-66 with titanium (Ti) via post-ligand exchange functionalization, and the Ti-exchanged UiO-66 material was mixed with PIM-1 matrix, yielding MMMs with enhanced CO2 permeability of 8,200 Barrer and a minor loss of selectivity (Smith et al., 2015). The improved permeability was strongly attributed to the interaction between the filler and polymer matrix, which led to the formation of interfacial free volume and a strong affinity with CO2. Meanwhile, a series of MMMs have been fabricated using UiO-66 with a variety of functional groups, with the latter strongly influencing the CO2 permeability and selectivity in the resulting MMM (Anjum et al., 2015; Smith et al., 2015; Venna et al., 2015; Khdhayyer et al., 2017).
ZIF—Based MMMs
Zeolitic imidazolate frameworks (ZIFs) is a subfamily of MOFs with a structure resembling that of zeolites. ZIFs exhibit tunable properties and possess high thermal and chemical stability due to the synergistic effect of the metal and imidazole groups. Phan et al. have reviewed ZIF based materials for CO2 capture and separation (Phan et al., 2010). ZIFs are made of metal nodes connected to organic imidazole linkers that replace the bridging oxygen of zeolite (Kontos et al., 2014). Meanwhile, various ZIF structures with different organic linkers have been employed as fillers for preparing MMMs. ZIFs can exhibit large cavities and narrow pore size, making them suitable as fillers for gas selective MMMs, particularly for CO2 separation, because the kinetic diameter of CO2 (3.3 Å) is comparable to the pore openings in several ZIFs. Also, ZIFs having the flexibility to adapt a second metal in their structure offer a synergetic effect that can result in higher performance gas separation in MMMs (Mirqasemi et al., 2020). Among the different polymers, Pebax is one of the most promising polymeric matrices due to its good characteristics in terms of flexibility, durability and thermo-mechanical properties. Also, Pebax possesses good selectivity toward polar–non-polar gases, such as CO2/N2. Various ZIFs have been incorporated into Pebax and other polymers to prepare MMMs (Phan et al., 2010; Kontos et al., 2014; Vinoba et al., 2017; Zheng et al., 2019). For example, MMMs were fabricated using ZIF-8 nanoparticles and poly (ether-block-amide) Pebax matrix. A microemulsion method was used for the preparation of the ZIF-8 nanoparticles with different sizes of 40, 60, 90, and 110 nm (ZIF-8-40, ZIF-8-60, ZIF-8-90, and ZIF-8-110) (Zheng et al., 2019). In this work, it was observed that ZIF-8 with smaller sizes possess a strong affinity with the polymer matrix resulting in strong interfacial interactions and defect-free MMMs. Figure 4A shows the effect of ZIF-8 particle size on the gas permeability and selectivity of PEBAX-based MMMs. MMM with 5 wt. % ZIF-8 (90 nm size) shows an increase in both CO2 permeability and CO2/N2 selectivity as compared to the pure Pebax membrane due to increase in the free volume of the polymer facilitated by the larger ZIF-8 particles. However, the CO2/N2 selectivity slightly decreased due to the microphase separation in the MMMs. The size of the ZIF-8 particles strongly influences the separation efficiency of MMMs (Zheng et al., 2019).
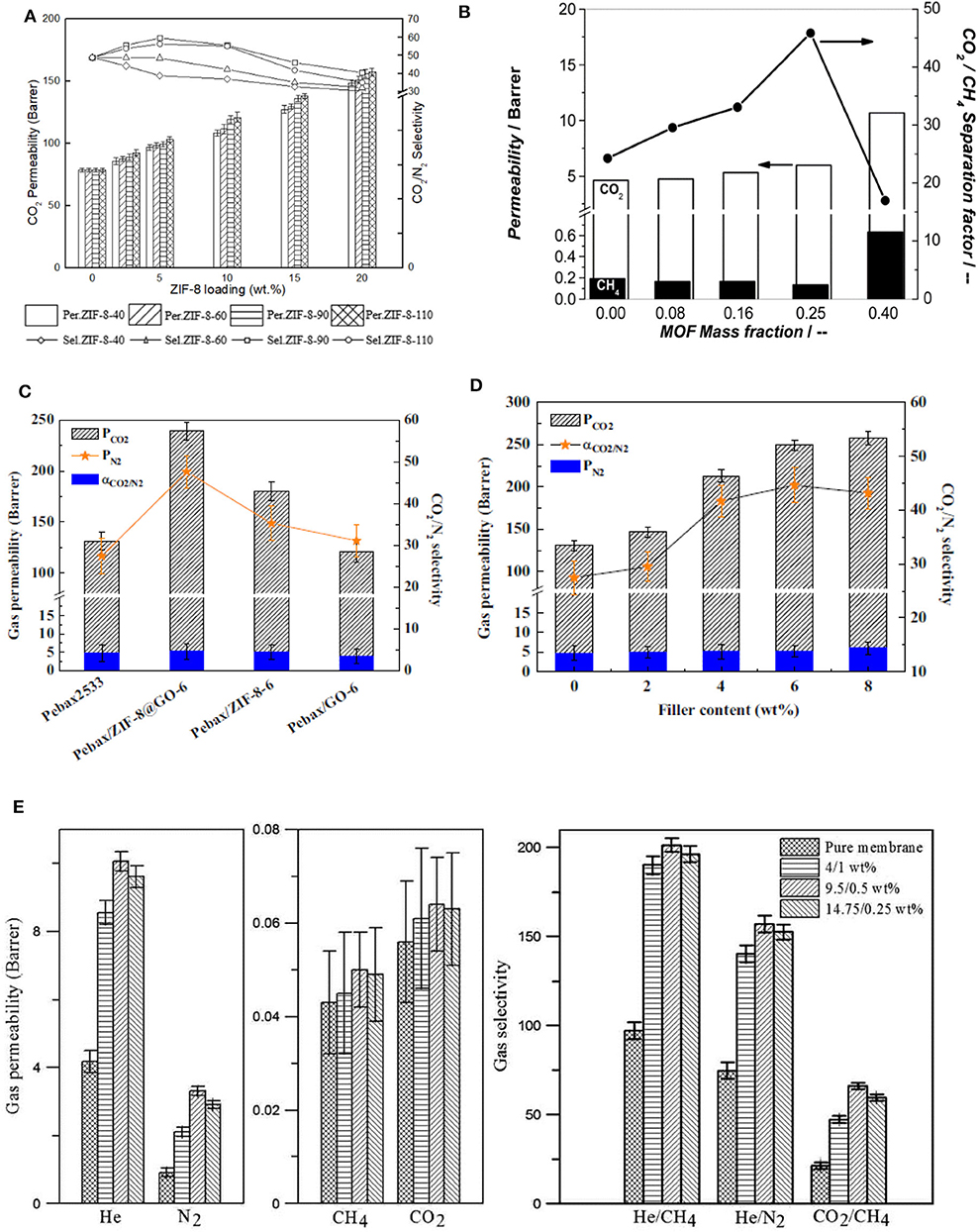
Figure 4. Characteristic examples of MOF filler effects: (A) Effect of ZIF-8 loading and size on CO2 permeability and CO2/N2 selectivity of Pebax-ZIF-8 MMMs. Reproduced from Zheng et al. (2019) with permission from Elsevier. (B) Separation performance of various NH2-MIL-53(Al) MMMs with different MOF loadings at 308 K for a mixture CO2:CH4 = 1:1. Pperm = 1 bar, Pret = 4 bar. CH4 (black bars) and CO2 (white bars) permeability (left y-axis) and CO2/CH4 separation factor (right y-axis). 1 Barrer = 3.348 × 10−19 kmol m/(m2 s Pa). Reproduced from Zornoza et al. (2011a) with permission from RSC. (C) Effect of different Pebax fillers, namely, MMMs with ZIF-8@GO, ZIF-8 and GO, and (D) filler content in Pebax/ZIF-8@GO MMMs. Reproduced from Dong et al. (2016a) with permission from Elsevier. (E) Gas permeability and selectivity of MMMs with different CuBTC/GO loadings. Reproduced from Feijani et al. (2018) with permission from RSC.
Castra-Munoz et al. fabricated MMMs using Matrimid®-PEG 200 matrices and ZIF-8, and incorporation of 30 wt. % of ZIF-8 via the traditional method increased the CO2 permeability to 130 Barrer. On the other hand, the MMM containing 30 wt. % ZIF-8 via the non-dried MOF method resulted in higher CO2/CH4 selectivity of 15 as compared to the MMM prepared by the traditional method (Castro-Muñoz and Fíla, 2019). Benavides et al. prepared high-performance MMMs using ZIF-94 and 6FDA-DAM polymer, and the MMM containing 40 wt. % of ZIF-94 showed the highest CO2 permeability of 2,310 Barrer with the CO2/N2 selectivity of 22 at 1 bar (Etxeberria-Benavides et al., 2018). Li et al. developed MMMs employing porous polyacrylonitrile (PAN) support and using small size ZIF-7 (30–35 nm), Pebax®1657 as the matrix, and PTMSP as the gutting layer to obtain MMMs with a smooth surface. At a lower filler content, both CO2 permeability and selectivity (CO2/CH4 and CO2/N2) of the MMM was higher than those of the pristine membrane. However, higher loading (34 wt. %) of ZIF-7 increased CO2/CH4 selectivity to 44, but reduced the permeability compared to pure Pebax®1657, because the higher ZIF-7 filler loading induced polymer rigidification (Li et al., 2013). Based on these studies, the overall MMM performance is affected by filler type, filler size, filler loading, and incorporation method (Phan et al., 2010; Sorribas et al., 2014; Thompson et al., 2014; Nordin et al., 2015; Dong et al., 2016a; Tanh Jeazet et al., 2016). Interestingly, ZIF-ionic liquid (IL) composite membranes have also been prepared to take advantage of the inherent affinity of several ILs with CO2 (Tzialla et al., 2013).
MIL—Based MMMs
MIL (Materials Institute Lavoisier) is another series of MOFs consisting of organic functional linkers connected with metal ions such as Al, Cr, Ti by corner-sharing. These MOFs possess large pore volume and surface area, and good adsorption capacity, while their framework is comprised of unidirectional, diamond-shaped pore channels. Recently, MILs have been used as fillers for the fabrication of MMMs due to their uniform dispersion and excellent compatibility with various polymers that make them suitable for gas separation. MILs can exist at multiple structures, namely MIL-53(Al), MIL-68(Al), MIL-101(Cr), and MIL-125(Al) (Rezakazemi et al., 2014; Vinoba et al., 2017). Many MILs have been prepared and used as fillers for the fabrication of MMMs (Ordoñez et al., 2010; Opanasenko et al., 2013; Luanwuthi et al., 2015; Niknam Shahrak et al., 2017; Chen Y. et al., 2018). Dorosti et al. fabricated MMMs using Matrid® polymer with different loadings (0–20 wt. %) of MIL-53 (Dorosti et al., 2014). The carboxylate linkage in the MIL-53 possesses a strong affinity with the Matrid® polymer and improves the interfacial adhesion, which resulted in a high separation performance of the MMMs. Individually, MMM containing 15 wt. % of MIL-53 exhibited a CO2/CH4 selectivity of 51.8 compared to 28.2 for the pure Matrid® membrane. Zornoza et al. fabricated MMMs using polysulfone and amine-functionalized (NH2-MIL-53(Al)) MIL. The addition of 25 wt. % of MIL into the polysulfone matrix enhanced CO2 transport and decreased the CH4 permeation, resulting in a significant increase of CO2/CH4 selectivity (Figure 4B). Notably, the optimum loading of the majority of inorganic fillers cannot exceed ~10 wt. % due to the mediocre filler–polymer adhesion/interaction (Zornoza et al., 2011a). For example, higher loading of amino-functionalized MOF suppressed the performance of polysulfone based MMMs, due to the formation of week hydrogen bonding between the amine and sulfone groups (Zornoza et al., 2011a).
MOF-74—Based MMMs
MOF-74 or M+/dobdc, where “M” is the identity of the metal node (Mg, Ni, Co, Zn etc.,), consists of metal ions linked together by 2,5 -dioxido-1,4-benzenedicarboxylate (dobdc) linkers, resulting in a framework of one-dimensional hexagonal morphology with uniform pores in a range of 3–10 Å (Suh et al., 2017). MOF-74 structures are used as adsorbents with high CO2 adsorption capacity as well as fillers for the fabrication of MMMs, resulting in enhanced CO2 separation performance.
Bachman et al. prepared MMMs using various polymer matrices such as cellulose acetate, commercial Matrimid@, 6FDA-DAT, 6FDA-DAM-DAT, 6FDA-DAM, 6FDA-durene with different loadings of Ni2(dobdc) (15–23 wt. %) (Bachman and Long, 2016). Ni(dobdc) possess a strong interaction with the polymer and substantially reduces the plasticization of the MMMs. In particular, the 6FDA-DA/15 wt. % Ni2(dobdc) exhibited the highest selectivity of 51.9 by retaining a permeability of 63.9 Barrer. Bae et al. developed MMMs using Mg2(dobdc) nanocrystals with the glassy polyimide and rubbery polymers. Glassy polyimide-baseded MMMs showed a significant increment in both the CO2 permeability and CO2/N2 selectivity. However, MMMs made with rubbery polymers exhibited a low permeability due to plugging of the outer pore windows of the Mg2(dobdc) pores by the rubbery polymer chains, which have high mobility at room temperature (Bae and Long, 2013). The MOF-74 loading plays a significant role in permselectivity of the MMMs, especially for the CO2/CH4 system (Bachman and Long, 2016; Li W. et al., 2019). Tien-Binh et al. (2016) prepared MMMs using PIM-1 and different loading of Mg(MOF-74) and the 20 wt. % of MOF-74 filler resulted in a defect-free MMM with CO2 permeability of 21.3 Barrer and CO2/CH4 selectivity of 19.1. Table 4 shows the CO2 separation efficiency of MOF-74 based MMMs.
MMMs Based on MOF Hybrids and Composites
It is worth to note that the controlled particle size of MOF crystallites can lead to better dispersion in the polymer matrix and result in defect-free membranes with high separation efficiency. However, the use of MOFs in MMMs still faces challenges such as the need for monodisperse particles and higher doping levels, and the risk of microvoid formation due to weak interfacial adhesion of the fillers with the polymer matrix (Lin et al., 2018). To mitigate these issues, researchers are focusing on the development of hybrid MOF fillers. Hybridizing MOFs with other materials including carbonaceous structures such as GO and CNTs results in new hybrid fillers that can exhibit strong dispersion within the polymer matrix, often increase the stability of the MOF counterparts, and create new porosity features and active sites, thus resulting in MMMs with a higher gas separation efficiency.
MOF/GO Hybrid Based MMMs
GO/MOF hybrids are often developed as fillers for fabricating defect-free MMMs with enhanced gas separation efficiency and multifunctionality. GO is known to have a high aspect ratio, which can play an essential role in increasing the selectivity of the resulting MMMs. The high aspect ratio of GO is responsible for increasing the path length, twists, and turns of the channels in MMMs, often allowing only small molecules to pass and restricting larger ones (Anastasiou et al., 2018). Of course, the absence of GO aggregates is a prerequisite for this behavior. Dong et al. reported the preparation of ZIF-8@GO hybrid via in situ growth of ZIF-8 on the surface of GO. This hybrid was incorporated into Pebax, and the effect of GO and ZIF-8 on the CO2 separation efficiency of the resultant MMM was studied. ZIF-8 filled PEBAX and GO incorporated PEBAX membranes were made as well, and their CO2 separation efficiencies as compared to the hybrid ZIF-8@GO based PEBAX membrane. It was observed that the CO2 permeability improved by 30% in ZIF-8/Pebax, mainly due to the presence of microporosity in the ZIF-8 itself. Moreover, ZIF-8@GO/Pebax showed a higher permeability (by 90%) and a selectivity of 1.7 relative to the pure Pebax due to the presence of GO along with ZIF-8 (Figures 4C,D) (Dong et al., 2016a).
Yang et al. (2019) incorporated ZIF-8@GO hybrid fillers into an ethylcellulose polymer matrix and studied its gas separation performance. The ZIF-8@GO formulation was prepared by the growth of ZIF-8 on the surface of GO through an ultrasonic synthesis method. Increasing the concentration of ZIF-8@GO increased the CO2 permeability of the MMM. The MMM with 15 wt. % of ZIF-8@GO hybrid filler showed the highest CO2 permeability of 203 Barrer and a CO2/N2 selectivity of 35 as compared to pure ethylcellulose membrane. Anastasiou et al. (2018) fabricated MMMs using PSF matrix and ZIF-8/GO hybrid filler through solution casting method and compared to pure PSF, and ZIF-8 filled MMM demonstrating that the ZIF-8/GO hybrid filler showed the highest performance in terms of both selectivity (CO2/N2 and CO2/CH4) and CO2 permeability. The intrinsic microporosity characteristics of the ZIF-8 component was responsible for the enhanced CO2 adsorption and increased CO2 permeability, while the GO component in the hybrid filler creates tortuous diffusion pathways in the MMM, allowing the small CO2 molecules to pass quickly and limiting the passage of larger gas molecules such as N2 and CH4, thus enhancing selectivity (Anastasiou et al., 2018).
Jia et al. synthesized hybrid UiO-66-NH2@GO nanoparticles via growth of UiO-66-NH2 on the surface of GO, and the hybrid nanoparticles were incorporated into a polyimide (PI) matrix to prepare MMMs. The MMM containing 5 wt. % of UiO-66-NH2@GO showed an enhanced CO2/N2 selectivity of 52 and CO2 permeability of 7 Barrer as compared to pure PI membrane (Jia et al., 2019). Feijani et al. prepared a new modified GO/MOF hybrid from CuBTC (copper (II) benzene-1,3,5-tricarboxylate) and GO nanosheets, and incorporated it into PVDF matrix, yielding hybrid MMMs with excellent mechanical and thermal properties, as well as enhanced performance in both selectivity and permeability (Feijani et al., 2018). Various filler concentrations were studied, and the optimum strength was found to be 9.5/0.5 wt. % of CuBTC/GO, resulting in the highest gas permeability and selectivity (Figure 4E). These positive effects are attributed to the filler morphology and uniform dispersion along with the excellent interaction with the polymer matrix (Feijani et al., 2018). Kang et al. prepared a set of thin and flexible MOF/GO composites with the support of Nylon matrix. HKUST-1 (copper-based MOF) was hybridized with GO through a layer-by-layer filtration method and was subsequently integrated into the polymeric membrane through in situ reaction with suitable cross-linkers. The as-fabricated membrane showed a H2/CO2 selectivity of 73 at 25°C and 1 bar (Kang et al., 2018). This approach can be extended to examine other MOFs combined with more flexible membranes. CO2 separation performance of the various MOF/GO based MMMs is shown in Table 5.
MOF/CNT Hybrid Based MMMs
To date, only a few articles report CNT-doped MOFs for MMMs preparation. Lin et al. enabled the effective separation of CO2/CH4 by CNT/MOF filled MMMs (Lin et al., 2015). The CNT/MOF composite was prepared by attaching NH2-MIL-101(Al) to the surface of CNTs and then incorporating it into the polyimide matrix to fabricate the MMMs (Figure 5A). A CNT/MOF hybrid containing CNTs modified with an additional amine group on the surface resulted in a strong adhesion with the polyimide matrix, even at the highest concentration of 15 wt. %. The CNT/MOF hybrid—filled MMMs exhibited both high CO2 permeability and high CO2/CH4 selectivity, while the optimum concentration of 5 wt. % of CNT/MOF hybrid led to CO2/CH4 selectivity of 29 and CO2 permeability of 780 Barrer. Sarfraz et al. developed MMMs by the simultaneous incorporation of ZIF crystals and CNTs into the polysulfone matrix and studied CO2 separation performance from humidified post-combustion gases. The hybrid fillers exhibited a synergetic effect in the MMM resulting in high CO2 permeability and CO2/N2 selectivity, while the MMM containing 18 wt. % ZIF-301 and 6 wt. % CNTs led to the most top separation performance with a CO2 permeability of 19 Barrer and CO2/N2 selectivity of 48 (Sarfraz and Ba-Shammakh, 2018).
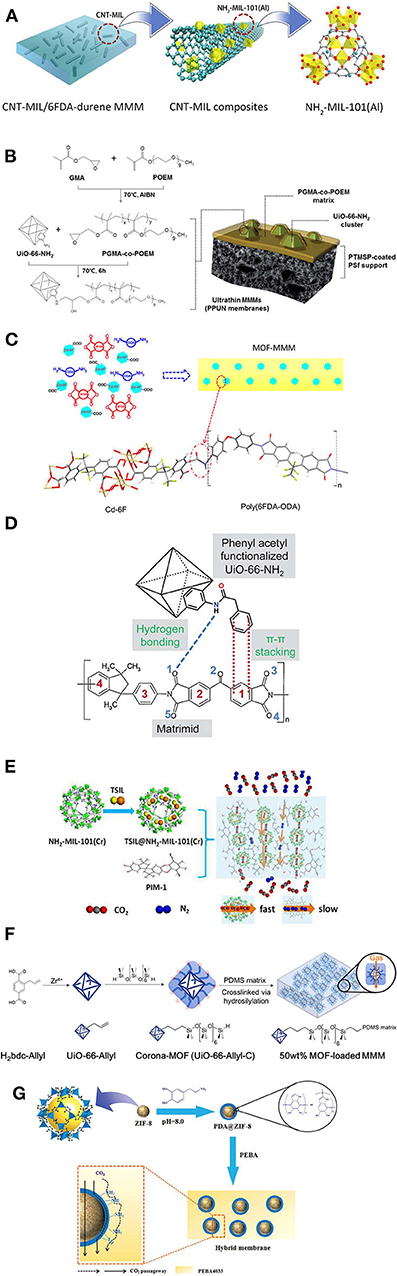
Figure 5. Schematic representations of characteristic examples of MOF MMM formation mechanisms and filler/matrix interactions: (A) NH2-MIL-101(Al)-decorated CNTs filled in 6FDA-durene membranes. Reproduced from Lin et al. (2015) with permission from ACS. (B) Synthesis of the PGMA-co-POEM copolymer and possible epoxide–amine reaction between UiO-66-NH2 and PGMA-co-POEM with illustration of ultrathin membranes. Reproduced from Kim et al. (2019) with permission from RSC. (C) Possible interaction between Cd-6F and 6FDA-ODA in MMMs upon in situ polymerization. Reproduced from Lin et al. (2014) with permission from ACS. (D) Possible interactions between the Matrimid® polymer and -PA modified MOFs. Reproduced from Venna et al. (2015) with permission from RSC. (E) Fabrication of TSIL@NH2-MIL-101 (Cr)/PIM-1 MMMs and the respective gas separation mechanism. Reproduced from Ma et al. (2016) with permission from RSC. (F) Formation of corona-MOFs and the respective corona-MOF loaded PDMS MMM. Reproduced from Katayama et al. (2019) with permission from ACS. (G) Formation of PDA@ZIF-8 and the possible CO2 transport mechanism in Pebax/PDA@ZIF-8 MMMs. Reproduced from Dong et al. (2016b) with permission from RSC.
Functionalized MOFs in MMMs
Although various MOFs have been studied for the fabrication of defect-free MMMs, challenges remain such as control of interface voids and the aggregation of MOF crystals in the polymer matrix (Mahdi and Tan, 2016; Wang Z. et al., 2016). In this regard, functionalized MOFs or post surface-modified MOFs are employed to improve the interfacial interaction between MOFs and the polymer matrix, as well as to tune filler interaction with CO2 (Liu et al., 2018a,b,c). MOF-based MMMs have been prepared using functionalized MOFs and post-synthetic modified (PSM) MOFs through in situ and ex-situ methods. Different functional groups, including (-NH2) (Fu et al., 2017), sulfonic(-SO3H) (Rather and Siddiqui, 2019), fluorine(-F) (Fan W. et al., 2018), bromine (-Br) (Zhang et al., 2016), hydroxyl (-OH) (Dau et al., 2012), and vinyl (Satheeshkumar et al., 2018) have been employed. In general, the resulting MOFs show chemical and physical properties that are substantially distinct from pure MOFs. Most functionalized MOFs are developed using functional organic ligands through in situ approaches. It is worth noting that each functional group possesses different properties and reactivity. In particular, amine-functionalized MOFs have been extensively studied for acid gas separation due to their inherent basic properties (Pokhrel et al., 2018a) based on which they can easily interact with acidic gases and exhibit high adsorption capacity (Pokhrel et al., 2018b). For this reason, amine-functionalized MOFs have gained considerable attention for the fabrication of MMMs as well. Also, amine-functionalized MOFs possess a strong interaction with the polymer matrix, e.g., through hydrogen bonding, resulting in defect-free MMMs. Li et al. developed polyimide (PI) MMMs by incorporating different types of amine-functionalized MOFs (Jiang et al., 2019). Even a high loading of amine-functionalized MOFs (20 wt. %) showed a uniform dispersion and a strong bonding with the PI matrix, yielding defect-free MMMs. A 20 wt. % of NH2-MIL-53 doped PI membrane showed CO2 permeability of 2.8 Barrer and CO2/CH4 and CO2/N2 selectivity of 24 and 25, respectively, at 40°C and 3 bar (Jiang et al., 2019). Shen et al. fabricated MMMs using UiO-66-NH2 nanocrystals and incorporation of these in a polyether block amide (PEBA) matrix. The bonding between the MOF and the polymer matrix provided enhanced structural stability in addition to high CO2 permeability of 130 Barrer and CO2/N2 selectivity of 72 at for 10 wt. % of MOF loading at 20°C and 3 bar (Shen et al., 2016). Kim et al. fabricated MMMs using UiO-66-NH2 and complex poly-glycidyl methacrylate-co-poly (oxyethylene methacrylate) (PGMA-co-POEM) matrix by covalently binding the UiO-66-NH2 particles with the polymer matrix through a facile epoxide-amine reaction resulting in void-free MMMs exhibiting strong interfacial interaction (Kim et al., 2019). Moreover, increasing the UiO-66-NH2 content resulted in a dual pathway in the MMMs, which enhanced the gas permeability of the membrane (Figure 5B). The MMM containing 28.6 wt. % of UiO-66-NH2 nanoparticles displayed CO2 permeance of 488 GPU and a CO2/N2 selectivity of 32 at 25°C (Kim et al., 2019)
Incorporation of Functionalized MOFs Into MMMs via an “in situ” Process
In situ, polymerization is an effective process in which the MOFs are covalently cross-linked to the chains of the polymer matrix. The technique eliminates the formation of non-selective interfacial voids and the agglomeration of MOFs particulates in the MMMs. In situ polymerization is a convenient process for the introduction of MOFs into the polymer matrix because the polymer precursors easily self-distribute among the MOF crystallites resulting in controlled polymerization at the interface. Moreover, a strong and controlled interfacial interaction is achieved by this method. Lin et al. prepared MMMs by the incorporation of 4, 4′-(hexafluoroisopropylidene) diphthalic anhydride (6FDA) linked MOF crystals (Cd-6F) through in situ polymerization of the 6FDA-ODA polyimide. During this thermal polymerization, the presence of the uncoordinated-COO group on the Cd-6F surface interacts with the -NH2 groups of the ODA monomer at the terminal end of poly(6FDA- ODA) chains, yielding a defect-free MMM (Figure 5C). This material showed a CO2 permeability of 38 Barrer and CO2/CH4 selectivity of 45 in 2 bar pressure at 25°C (Lin et al., 2014).
Molavi et al. developed MMMs through in situ radical polymerization using methyl methacrylate with UiO-66, UiO-66-NH2 and vinyl group attached UiO-66. The in-situ polymerization provided substantial dispersion and interaction of UiO-66 nanoparticles within the PMMA matrix via covalent bonding. The polymer formation on UiO-66 nanoparticles reduced the agglomeration, yet resulted in non-selective interfacial voids in the MMMs. However, polymer grafting can partially block the porosity of the UiO-66, thus the gas separation often occurs only through a molecular sieving mechanism. Therefore, polymer grafted UiO-66 based MMMs showed a low CO2 permeability yet high CO2/CH4 (150) and CO2/N2 (110) selectivity due to the rigid interface in the MMMs (Molavi et al., 2018). Kumar et al. developed a MMM using vinyl-functionalized UiO-66, poly(ethylene glycol) divinyl ether (PEO-250), pentaerythritol tetra(3-mercaptopropionate) (PETM), 2,2′-(ethylenedioxy) diethanethiol (EDDT), and 2,2-dimethoxy-2-phenylacetophenone (DMPA) through an in situ thiol-ene photopolymerization. The vinyl-functionalized UiO-66 particles were covalently crosslinked with the polymer matrix through the C–S bond resulting in a strong adhesion of the MOF with the polymer matrix, yielding a void-free interface. Moreover, the photopolymerized MMM showed high flexibility and good mechanical strength. The 60 wt. % vinyl-functionalized UiO-66 MMM showed CO2 permeability of 467 Barrer and CO2/CH4 selectivity of 8 at 25°C and 5 bar (Satheeshkumar et al., 2018). Tien-Binh et al. fabricated MMM by in situ crosslinking of NH2-UiO-66 with a polymer possessing intrinsic microporosity (PIM-1). The in-situ polymerization yielded a high degree of interfacial coupling between the MOF and the polymer matrix. As a result, 20 wt. % of NH2-UiO-66 loaded MMM exhibited CO2 permeability of 12,498 Barrer with CO2/CH4 selectivity of 31.9 at 2 bar and 25°C (Tien-Binh et al., 2018).
Post-synthetically Modified MOFs in MMMs
Post-synthetic modification (PSM) is another strategy of modifying MOFs, in which organic functionalities and cross-linkers are grafted on the surface of the MOF particles to yield a functionalized material. PSM has certain advantages over the pre-functionalization method described in the previous section because a large number of functional groups can be incorporated into the MOFs without affecting the overall stability of the framework (Tanabe and Cohen, 2011). Amine-functionalized MOFs are frequently used for the PSM process because the amine groups, in addition to their affinity with CO2, they are highly reactive to other functional groups such as aldehydes, anhydrides, acyl halides, and epoxy groups thus enhancing the interaction of the funcltionalized MOFs with the polymer matrix. Selection of suitable functional groups depends on the chemistry of the polymer matrix. Post-modified MOFs are expected to possess a high dispersion and strong interfacial interactions with the polymer matrix, ensuing void-free MMMs.
Jiang et al. grafted UiO-66-NH2 with imidazole-2-carbaldehyde (ICA) through the PSM approach, and the modified MOF (UiO-66-NH2@ICA) was incorporated into a Matrimid® polymer (Jiang et al., 2019). The PSM increased the number of nitrogen atoms in the modified MOF via the imidazole group, which increased the dipole-quadrupole interactions between CO2 and nitrogen. The incorporation of 10 wt. % UiO-66-NH2@ICA into the Matrimid® polymer resulted in defect-free interfaces in the MMM, which exhibited a CO2 permeability of 40.1 Barrer and CO2/CH4 selectivity of 64.7 at 25°C and 3 bar (Jiang et al., 2019). Veena et al. modified the surface of UiO-66-NH2 using phenyl acetyl chloride (-PA) cross-linkers, decanoyl chloride, and succinic anhydride producing three different MOFs of aromatic-modified I (IPA), aliphatic C10-modified I (IC10), and acid-modified I (ISA) (Venna et al., 2015). The modified MOFs were incorporated into Matrimid®, and the surface-modified and unmodified MOFs showed nearly similar internal structure and functionality. However, the functional groups on the MOF surface influenced the interfacial adhesion between the polymer and the MOFs. Among the surface-modified MOFs, the -PA functionalized ones (IPA) showed a positive effect in the MMM performance, because of the enhanced π-π interactions between the aromatic groups in both the polymer and the phenyl acetyl (-PA) groups of the MOF structure. The bonding between the imide group of the polymer and the amine groups of IPA (Figure 5D) contributed positively as well. The MMM consisting of 23 wt. % of -PA surface-functionalized MOFs (IPA) exhibited CO2 permeability of 28 Barrer and CO2/N2 selectivity of 37 at 1 bar and room temperature (Venna et al., 2015). Zhu et al. post-modified the surface of MIL(53) using aminopropyltriethoxysilane (APTES), which was then incorporated into poly(ether imide) Ultem1000 to prepare high-quality asymmetric MMMs. The as-modified MIL-53 (S-MIL-53 (Al)) surface contained enriched amine groups, which enabled strong bonding with the amide groups of the polymer, thus enhancing the interfacial interaction with the matrix, favored dipole–quadrupole interactions with the CO2, and consequently increased the CO2 separation efficiency of the resulting MMMs. An asymmetric MMM consisting of 10 wt. % S-MIL-53 (Al) exhibited CO2 permeance of 24 GPU, which is 165% higher than that of the pure membrane (Zhu et al., 2016).
Ionic Liquid Modified MOFs in MMMs
In recent years, ionic liquids (ILs) were studied intensely for CO2 adsorption and separation processes due to their physicochemical properties such as high thermal and electrochemical stability, negligible volatility, and high CO2 solubility and selectivity (Perdikaki et al., 2012, 2016; Tzialla et al., 2013; Dai et al., 2016; Karousos et al., 2017, 2018). Based on these favorable properties, ILs have been applied as binder material for the fabrication of MMMs, which considerably improved the interfacial adhesion between filler/polymer and simultaneously enhanced the gas separation performance (Dai et al., 2016). Also, IL-based composite membranes have the potential of dual-action by combining capture and catalytic conversion performance (Perdikaki et al., 2012, 2016). In polymer-based MMMs however, the direct incorporation of ILs could reside in the free volume of the polymer matrix, thereby decreasing the gas diffusion properties of the resultant MMMs. Therefore, controlled inclusion of ILs is required during the fabrication of the MMMs. Grafting of ILs into the MOFs is one of the post-synthetic modification processes that could increase the interfacial affinity between the MOF and the polymer matrix. As a result, IL-modified MOF based MMMs can show high permeability and selectivity, mainly due to the high CO2 affinity of the ILs (Tzialla et al., 2013; Vicent-Luna et al., 2013; Ma et al., 2016). Lin et al. fabricated MMMs using the 6FDA-Durene polymer and an IL-coated HKUST. The layer of IL in MOF enhanced MOF-polymer interaction resulting in void-free MMMs with high CO2 permeability of 1101.6 Barrer and CO2/CH4 selectivity of 29.3 at 25°C and 2 bar (Lin et al., 2016). Li et al. studied CO2 selectivity and the mechanical properties of MMMs based on ZIF-8 modified by the ionic liquid of [bmim][Tf2N]. Incorporation of 15 wt. % of IL@ZIF-8 into Pebax matrix showed a significant increase in the tensile strength by ~20% and elongation at the break by 280%, which is higher than those of the non-modified membrane. Also, the MMM containing 15 wt. % of IL@ZIF-8 exhibited significant improvement in CO2 permeability to a value of 104.9 Barrer with CO2/CH4 selectivity of 34.8 at 25°C and 1 bar (Li et al., 2016). Ma et al. impregnated the task-specific ionic liquid (TSIL) of [C3NH2bim][Tf2N] on NH2-MIL-101(Cr) through a host-guest interaction approach. The MOF textural features enabled high dispersion of TSIL leading to the large active surface of the TSIL, which significantly enhanced the CO2 selectivity of a gas pair in the resulting MMMs. Furthermore, the NH2-MIL-101(Cr) contributed to the rapid CO2 transport and increased CO2 permeability (Figure 5E). The MMMs were prepared using different loadings of TSIL-modified NH2-MIL-101(Cr) in PIM-1 polymer, and 5 wt. % of TSIL-modified NH2-MIL-101(Cr) in the PIM-1 membrane resulted in CO2 permeability of 2,979 Barrer and CO2/N2 selectivity of 37 at 25°C and 3 bar (Ma et al., 2016). Interestingly, MOF-ionic liquid (IL) composite membranes have also been prepared with the MOF phase constituting most of the membrane volume. Specifically, ZIF-69 membranes were grown on porous α-alumina supports via seeded secondary growth and further functionalized by a CO2-selective tricyanomethanide anion/alkylmethylimidazolium cation-based ionic liquid (IL) to plug the gaps between the ZIF crystals yet leave the framework pores open for gas diffusion. The developed membranes at room temperature and under a 2 bar pressure exhibited CO2 permeance of 5.6 × 10−11 and 3.7 × 10−11 mol m−2 s−1 Pa−1 and CO2/N2 selectivity of 44 and 64 for CO2/N2 mixture, respectively (Tzialla et al., 2013).
Polymer-Coated MOFs in MMMs
A well-controlled interface between MOF/polymer phases could eliminate the non-selective defects in the MMMs and improve the gas separation performance. The interfacial affinity between the MOF/polymer phases depends to a large extent on the structure of the polymer matrix and the surface functionality of the MOFs (Guo et al., 2018; Jiang et al., 2018). Thus, carefully controlled modification of the polymeric phase on the MOF surface is needed for enhancing the permeability and selectivity of the resulting MMMs. Non-controlled polymer growth is unwanted as this may partially block the pores of the MOF, leading to permeability decrease (Li et al., 2006). Occasionally, weak interface adhesion is observed in few of the MOF-based MMMs, particularly involving micron-sized MOF fillers. Reducing the size of MOF particles can promote the interfacial surface area and increase the interfacial interactions (Li et al., 2005). Polymerization reactions such as radical, ionic, oxidative, and photopolymerizations have been employed to modify the surface of MOFs, often also resulting in the controlled growth of polymeric phase into the larger cavities of the MOFs (Zhang et al., 2015; Kitao et al., 2017). Wang et al. prepared covalently grafted polyimide brushes on the surface of various MOFs. These structures behaved as free-standing membranes at high loadings up to 88 wt. % of MOF even without incorporation of the additional polymeric matrix. The modified MMMs have shown high elasticity up to 472% due to a decrease in the matrix chain mobility and exhibited a significant plasticization resistance against CO2 as compared to the conventional MMMs. Also, the modified MMMs showed a simultaneous increase in both permeability and selectivity for CO2/N2 and CO2/CH4 separation (Wang H. et al., 2018). Katayama et al. modified allyl terminated UiO-66 with hydride-terminated poly (dimethylsiloxane), yielding a PDMS-coated MOF (“corona-MOF”). 50 wt. % of corona-MOF particles were subsequently incorporated to the PDMS matrix to fabricate a defect-free MMM (Katayama et al., 2019). Corona-MOF based MMMs possess a robust covalent interaction between both MOF and the polymer phases leading to negligible particle aggregation during the film curing and resulting in free-standing, flexible MMMs of <1 μm thickness (Figure 5F). Moreover, corona-MOF based MMMs did not exhibit any undesired formations such as plugged sieves and sieve-in-a-cage ones, and showed high CO2 permeability (4,859 ± 727 Barrer) without loss in selectivity (CO2/N2 of 10) at 1 bar (Katayama et al., 2019).
Dong et al. developed MMMs using a Pebax matrix with different loadings of polydopamine (PDA)—coated ZIF-8 (Dong et al., 2016b). The PDA coating could eliminate the interfacial defects in the MMM and enhance the interfacial adhesion between ZIF-8 and the polymer matrix. The permeability and selectivity of MMMs often vary in humid or dry conditions, a fact that should be taken into consideration when it comes to practical applications. In humid conditions, the amine groups in PDA@ZIF-8 acted as CO2 adsorption sites at the polymer/MOF interface. The CO2 molecules pass through the membrane via both a transport mechanism and a solution-diffusion mechanism (Figure 5G). A 15 wt. % of PDA@ZIF-8 Pebax membrane showed a CO2 permeation of 267 Barrer and a CO2/N2 selectivity of 62 at 1 bar and 25°C in humid conditions. At dry conditions though, the CO2 permeation follows only a solution-diffusion mechanism resulting in a decrease in CO2 permeability (220 Barrer) and CO2/N2 selectivity (56) at the same pressure and temperature (Dong et al., 2016b). Guo et al. modified the surface of CAU-1 nanoparticles using polyethyleneimine (PEI) with glutaraldehyde (GA) as an intermediate covalent cross-linker. As-modified CAU-1 (PEI-CAU-1) nanoparticles were incorporated into poly (ethylene glycol) diacrylate to fabricate MMM through a photopolymerization reaction. The enriched amine groups in the PEI-CAU-1 acted as CO2 carriers favoring the CO2 transport through the MMMs. The PEI functionalization also reduced the MOF aggregation in the MMM. An MMM consisting of 30 wt. % of PEI-CAU exhibited CO2 permeability of 546 Barrer and CO2/CH4 selectivity 27.8 at 35°C and 3 bar (Guo et al., 2018).
The Trade-Off Curves of MOF-Based MMMs for CO2/CH4 and CO2/N2 Separation
Robeson first reported the general trade-off between permeability and selectivity for polymeric membranes in 1991 (Robeson, 1991), which was further updated with new data in 2008 (Robeson, 2008). The Robeson trade-off curves provide a direction to the researchers toward the development of new efficient membranes to surpass the existing permeability and selectivity performances for common gas pairs. A primary goal of MMMs prepared in a combination of a polymer matrix with various fillers is to achieve better perm-selectivity and surpass the current Robeson trade-off curve. Redefining the Roberson upper bound in 2019 (Comesaña-Gándara et al., 2019) set new aspirational targets for the membrane community to aim more attractive parametric optimization of performance, energy use, and cost-efficiency of the CO2 separation process. The Robeson trade-off line is represented by , where Pi is the permeability of the more mobile gas, αij is the selectivity of component i over component j, k is the constant factor, and n is the slope of the log-log graph of the above equation. The Robeson trade-off line parameters for the CO2/CH4 gas pair is shown in Table 6. The permeability and selectivity values for CO2/CH4 and CO2/N2 separation using MMMs based on this review along with the redefined Robeson upper bounds of 2019 are shown in Figures 6A,B. The data provide an insight into the MMMs development from 1998 to the present. It can be noticed that MMMs based on MOF composite/hybrid fillers as well as on functionalized MOFs show better performance and some of these cross the 2019 redefined Roberson upper bound, as presented in Table 7. As a characteristic example, CNT/MOF composite fillers prepared by attaching NH2-MIL-101(Al) to the surface of CNTs and then incorporated in polyimide showed CO2 permeability of 818 Barrer with CO2/CH4 selectivity of 29.7 (Lin et al., 2015). In addition, MMMs fabricated by in situ crosslinking of NH2-UiO-66 with a polymer of intrinsic microporosity (PIM-1) exhibited a high degree of the interfacial binding between the MOF and the polymer matrix. As a result, 20 wt. % of UiO-66-NH2 MMM showed CO2 permeability of 12,498 Barrer with CO2/CH4 selectivity of 31.9 at 2 bar and 25°C (Tien-Binh et al., 2018). Hence, MMMs comprised of MOF hybrid and functionalized fillers in a suitable polymer matrix constitute promising perm-selectivity membranes for CO2 abundant streams.
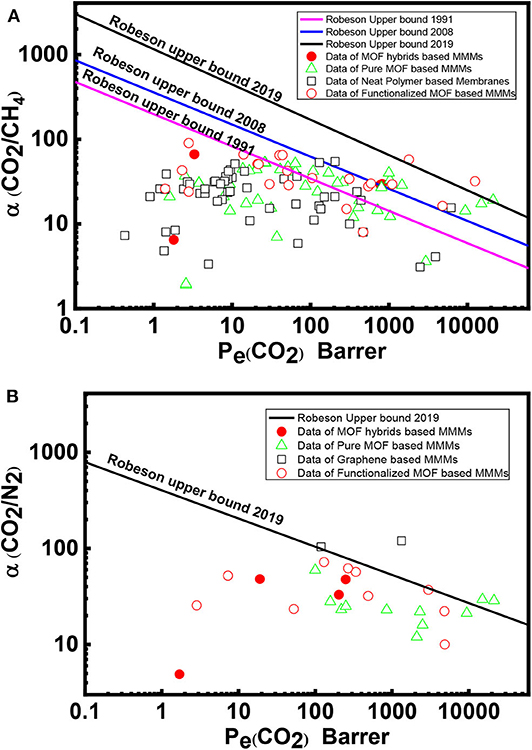
Figure 6. The performance of MOF-based MMMs [HKUST-1 (Car et al., 2006; Hu et al., 2010; Ge et al., 2013; Lin et al., 2016; Feijani et al., 2018; Chi et al., 2019); ZIF-7 (Li et al., 2013; Azizi and Hojjati, 2018); ZIF-8 (Ordoñez et al., 2010; Ban et al., 2015; Shahid et al., 2015; Dong et al., 2016a,b; Jusoh et al., 2016; Lin et al., 2016; Anastasiou et al., 2018; Castro-Muñoz and Fíla, 2019; Yang et al., 2019; Zheng et al., 2019); UiO-66 (Nik et al., 2012; Dong et al., 2016b; Shen et al., 2016; Satheeshkumar et al., 2018; Sutrisna et al., 2018; Tien-Binh et al., 2018; Zamidi Ahmad et al., 2018; Jia et al., 2019; Jiang et al., 2019; Katayama et al., 2019; Kim et al., 2019); MOF-5 (Perez et al., 2009); MIL-53 (Zornoza et al., 2011a; Chen et al., 2013; Hsieh et al., 2014; Ahmadi Feijani et al., 2015; Feijani et al., 2015; Tien-Binh et al., 2015; Mubashir et al., 2018b; Jiang et al., 2019); ZIF-90 (Bae et al., 2010); ZIF-11 (Safak Boroglu and Yumru, 2017); KAUST-7 (Chen K. et al., 2018); CuBDC (Cheng et al., 2017); Mg-MOF-74 (Bae and Long, 2013; Tien-Binh et al., 2016; Smith et al., 2018); Ni-MOF-74 (Bachman and Long, 2016; Yang et al., 2019); ZIF-94 (Etxeberria-Benavides et al., 2018); MIL-101 (Xin et al., 2015; Ma et al., 2016); Bio-MOF-1 (Ishaq et al., 2019); Fe(BTC) (Nabais et al., 2018)] included in this review work for (A) CO2/CH4, and (B) CO2/N2 separation data in conjunction to redefined Robeson upper bounds (2019).
Comesaña-Gándara et al. redefined the Robeson upper bound in 2019 for the CO2/N2 separation with k and n values of 755,580,000 and −3.409, respectively (Comesaña-Gándara et al., 2019). The permeability and selectivity for the CO2/N2 gas pair based on this review along with 2019 redefined Robeson upper bound are shown in Figure 6B. Notably, TSIL-modified NH2-MIL-101(Cr) loaded PIM-1 MMM showed a CO2 permeation of 2,979 Barrer and a CO2/N2 selectivity of 37 (Ma et al., 2016). As a comparison, functionalized graphene-based MMMs can cross or exceed the upper bound. For instance, PEG–PEI–GO (10 wt. %) loaded in Pebax MH 1657 membrane exhibits CO2 permeability of 1,330 Barrer and CO2/N2 selectivity of 120 (Li et al., 2015). Efforts need to continue to further optimize MOF-based MMMs according to the nature of the polymer matrix and the structure of the MOFs paying attention to filler-matrix interaction, processing methods, membrane thermal and mechanical stability and including innovative fabrication of functionalized MOFs and hybrid MOF systems. Designing new MOF-based nanoparticle combinations as fillers could be a promising approach for the development of MMMs to surpass the Robeson upper bound limits.
Perspectives on MMMs and MOF-Based MMMs for Large-Scale Integration
The integration of membranes in commercial applications has taken off in the last decade. For instance, cellulose acetate membranes tend to become the standard practice in the Far East for the removal of CO2 from natural gas. These applications, mostly off-shore, aim to remove CO2 from high-pressure streams (40–70 bar) from typical 10–30% CO2 to pipeline specification (~2–5% CO2), which is regarded as bulk separation. The main advantage of membrane separation over conventional processes is that the membranes do not require bulky columns and heat for regeneration in contrast to the traditional amine absorption processes. Overall, this provides an economic incentive to utilize membranes. Two leading membrane suppliers are UOP and Schlumberger (formerly NATCO) who employ improved versions of celluloses acetate membranes. Another high-pressure application under consideration is the removal of CO2 from synthesis gas (H2 and CO at 15–30 bar), but no related commercial applications are known to the authors. Recently, there has been a considerable interest to apply membranes also for low-pressure flue gas streams. This means that the separation is from nitrogen-rich streams at very high volumetric flowrates and likewise, that the demand on the membrane performance becomes even higher to make such an application economically feasible.
In this paper, developments of a specific type of membranes (MOF-based MMMs) are reviewed. Membrane performance is generally expressed to permeability and selectivity, yet it should be realized more factors are dictating the overall economics of a membrane process. Most importantly, it should be appreciated that the separation is based on the difference in partial pressure of the component over the membrane and this driving force is a limiting factor, either because the partial pressure in the inlet gas is low, or a very tight specification (<1 bar partial pressure) should be met. For high-pressure streams, it means that the application is restricted to bulk separation. For low-pressure streams, it means that compression must be applied at the retentate side or vacuum at the permeate side and this is often an inhibiting factor for the economics of the process.
In general, the permeance will determine the required membrane surface area. Higher permeance leads to lower membrane areas and thus more economical cost. The selectivity will evaluate the losses of the component that should not be permeating. In the case of natural gas treatment, lower selectivity leads to higher loss of methane to the CO2 stream, which is an economic loss. This can be unusually large at high partial pressures of CO2. It should be realized that polymeric membranes tend to lose a significant fraction of their selectivity at high partial pressures of components like CO2 (also H2S) due to a plasticization. So, the performance should be evaluated at the appropriate partial pressure. The selectivity with respect to N2 is less of an issue as loss of N2 has no value consequence, while the permeating CO2 does not have to be ultra-pure. Indicatively, it can contain up to 2% of inerts for enhanced oil recovery (EOR) purposes.
Another limiting factor to selectivity is the pressure ratio between inlet P0 and permeating stream P-L. For instance, the relation trend between permeate vapor concentration and the pressure ratio (Po/PL) indicates that increasing the selectivity above the pressure ratio is not useful. For high-pressure systems, this means that a selectivity above 50 is not entirely useful (inlet pressure 50–60 bar, outlet 1 bar, pressure ratio 50). When CO2 must be injected, it is beneficial to have the CO2 permeating at elevated pressure. This will limit the flux to some extend because it depends on the absolute pressure difference (P0, CO2-PL, CO2) only. The pressure ratio will be strongly affected though. When PL is increased to 4 bar the pressure ratio may go down to around 10, and it is not useful to have a selectivity above this value. For flue gas treatment, the pressure ratio is the most important factor determining the process because both compression and vacuum are needed. As an example, with compression up to 3 bar and vacuum of 0.1 bar at the permeate side the pressure ratio is 30. These are the values proposed by the leading party in this field, MTR. MTR aims a permeance of 1,500 GPU and a selectivity of 25–30 (Baker et al., 2018). This means optimizing flux, and thus permeance is more important than improving selectivity.
The membrane process providers have developed process flow schemes that have improved characteristics of the overall process, such as multi-stage membrane systems, recycle options, and sweep gas. This may influence the optimal parameters of the membrane. To establish that, detailed process design and sensitivity studies need to be executed. However, this will not influence the major conclusion, i.e., that permeance is the most critical factor. Based on this review and the Robeson plots of Figure 6, the most promising systems are the MOF hybrids, functionalized MOFs, and graphene-based MMM systems. Currently, the selectivity is considered well within what is required, so it is advised to look at systems with higher flux, even at a lower selectivity (25–50). It should be noted that polymeric membrane systems are also improving, which means that only time will tell which system will be the winner.
Finally, it should be realized that other factors determine the success of a membrane system as well such as the ease of processing, potting, the lifetime, and resistance to contaminants. Especially the latter requires particular attention and determines the need for pretreatment or heating of the gas flow. That is beyond the purpose of this review, but generally, it requires extensive development. Sometimes these issues only emerge in field trials.
Summary and Outlook
Applications of MMMs in gas separation have attracted great attention in recent years. In this review, through selected examples we analyze the advances made on MOF-based MMMs for CO2 separation. The review provides an overview of the merits of MOF MMMs compared to the polymeric and inorganic membranes, and it documents that MOF-based MMMs show a promising performance and have the potential to overcome the traditional permeability and/or selectivity trade-off barriers. The significance of MOFs on the development of MMMs is highlighted in conjunction with the redefined Robeson upper bounds (2019). The current reports reveal that MOFs are attractive fillers offering unique performance features to the resulting MMMs in terms of high permeability combined with enhanced selectivity as long as the fillers, matrix, and the interaction between these two phases are properly configured. The size, structure, porosity, and nature of organic ligands in the MOFs also play a vital role in the effective dispersion within the polymer matrix, in the separation behavior, as well as in enhancing the membrane robustness.
Interface engineering and surface functionalization can improve adhesion and yield defect-free formulations. Indeed, at high filler loadings in particular, voids may develop resulting in reduction in separation performance. This issue can be addressed by functionalization of the MOF surfaces thus offering a multiple benefit of strong interfacial adhesion between MOFs and the polymer, better dispersion and prevention of agglomeration of the MOF crystallites in the MMM, and tuning of the transport behavior of the permeating gas molecules. In addition, modification of the internal pore surface of the MOF fillers needs to be further investigated. The confinement of organic species into the nanocages of MOFs, e.g., via in-situ or wet post-growth process should be carried out systematically. Such structure can provide advanced porous MOF characteristics and tunable filler performance within the membrane matrix for targeted separations, which combined to the intrinsic pore merits can manipulate diffusion behavior and enhance molecular retention, diffusion, and separation (Smaldone et al., 2010; Hartlieb et al., 2016). Significant attention and promising performance are also associated with hybrid fillers that offer multifunctionality thus serving more targets and specifications compared to mono-fillers. For example, graphene oxide, CNTs, zeolite and silica nanoparticles are often combined with MOFs to tune the permeability, selectivity, filler dispersion, porosity, and the interfacial properties in the resulting MMMs. For instance, in MOF/GO hybrid—based MMMs, MOFs can enhance permeability and GO can enhance selectivity thus both targets are being met.
Overall, MOFs are suitable fillers in MMMs, yet there is still ample room for continued research particularly on functionalization and hybridization of these structures to meet the targets of high permeability and selectivity, good mechanical, chemical, and thermal stability, scalability, and low cost, thus paving the route for the MOF-based MMMs to deliver on their promises in today's demanding CO2 separation needs.
Author Contributions
All authors contributed in the collection of data, critical evaluation, and writing of this review.
Conflict of Interest
FG was employed by the company ADNOC Gas Processing.
The remaining authors declare that the research was conducted in the absence of any commercial or financial relationships that could be construed as a potential conflict of interest.
Acknowledgments
The authors acknowledge support by the Gas Research Center (GRC16001 and GRC16002), and the Center for Catalysis and Separations (CeCaS, award RC2-2018-024) of Khalifa University.
References
Abdulrahman, R. K., and Sebastine, I. M. (2013). Natural gas sweetening process simulation and optimization: a case study of khurmala field in iraqi kurdistan region. J. Nat. Gas Sci. Eng. 14, 116–120. doi: 10.1016/j.jngse.2013.06.005
Adewole, J. K., Ahmad, A. L., Ismail, S., and Leo, C. P. (2013). Current challenges in membrane separation of CO2 from natural gas: a review. Int. J. Greenhouse Gas Control 17, 46–65. doi: 10.1016/j.ijggc.2013.04.012
Afarani, H. T., Sadeghi, M., and Moheb, A. (2018). The gas separation performance of polyurethane–zeolite mixed matrix membranes. Adv. Polym. Technol. 37, 339–348. doi: 10.1002/adv.21672
Ahmadi Feijani, E., Tavasoli, A., and Mahdavi, H. (2015). Improving gas separation performance of poly(vinylidene fluoride) based mixed matrix membranes containing metal–organic frameworks by chemical Modification. Ind. Eng. Chem. Res 54, 12124–12134. doi: 10.1021/acs.iecr.5b02549
Alomair, A. A., Al-Jubouri, S. M., and Holmes, S. M. (2015). A novel approach to fabricate zeolite membranes for pervaporation processes. J. Mater. Chem. A 3, 9799–9806. doi: 10.1039/C5TA00124B
Alqaheem, Y., Alomair, A., and Vinoba, M. (2017). #xe9, A. rez and #xe9, polymeric gas-separation membranes for petroleum, refining. Int. J. Poly. Sci. 2017:19. doi: 10.1155/2017/4250927
Althues, H., Henle, J., and Kaskel, S. (2007). Functional inorganic nanofillers for transparent polymers. Chem. Soc. Rev 36, 1454–1465. doi: 10.1039/b608177k
Anastasiou, S., Bhoria, N., Pokhrel, J., Kumar Reddy, K. S., Srinivasakannan, C., Wang, K., et al. (2018). Metal-organic framework/graphene oxide composite fillers in mixed-matrix membranes for CO2 separation. Mater. Chem. Phys. 212, 513–522. doi: 10.1016/j.matchemphys.2018.03.064
Anjum, M. W., Vermoortele, F., Khan, A. L., Bueken, B., De Vos, D. E., and Vankelecom, I. F. J. (2015). Modulated UiO-66-based mixed-matrix membranes for CO2 separation. ACS Appl. Mater. Interfaces 7, 25193–25201. doi: 10.1021/acsami.5b08964
Aroon, M. A., Ismail, A. F., Montazer-Rahmati, M. M., and Matsuura, T. (2010). Effect of chitosan as a functionalization agent on the performance and separation properties of polyimide/multi-walled carbon nanotubes mixed matrix flat sheet membranes. J. Memb. Sci. 364, 309–317. doi: 10.1016/j.memsci.2010.08.023
Aykac Ozen, H., and Ozturk, B. (2019). Gas separation characteristic of mixed matrix membrane prepared by MOF-5 including different metals. Sep. Puriff. Technol. 211, 514–521. doi: 10.1016/j.seppur.2018.09.052
Azizi, N., and Hojjati, M. R. (2018). Using Pebax-1074/ZIF-7 mixed matrix membranes for separation of CO2 from CH4. Pet. Sci. Technol 36, 993–1000. doi: 10.1080/10916466.2018.1458120
Bachman, J. E., and Long, J. R. (2016). Plasticization-resistant Ni2(dobdc)/polyimide composite membranes for the removal of CO2 from natural gas. Energy Environ. Sci. 9, 2031–2036. doi: 10.1039/C6EE00865H
Bae, T.-H., Lee, J. S., Qiu, W., Koros, W. J., Jones, C. W., and Nair, S. (2010). A high-performance gas-separation membrane containing submicrometer-sized metal–organic framework crystals. Angew. Chem. Int. Ed. 49, 9863–9866. doi: 10.1002/anie.201006141
Bae, T.-H., and Long, J. R. (2013). CO2/N2 separations with mixed-matrix membranes containing Mg2(dobdc) nanocrystals. Energy Environ. Sci. 6, 3565–3569. doi: 10.1039/c3ee42394h
Baker, R. W., Merkel, T., and Freeman, B. C. (2018). Large Pilot Testing of the MTR Membrane Post-Combustion CO2 Capture Process. Available online at: https://www.netl.doe.gov/sites/default/files/netl-file/MTR-Kickoff-Presentation-Public-FE0031587-FOA1788.pdf
Bakhtin, D., Eremin, Y. S., Grekhov, A. M., and Volkov, V. V. (2015). Gas permeability of PVTMS/CNT mixed matrix membranes. Phys. Proc. 72, 166–170. doi: 10.1016/j.phpro.2015.09.049
Balta, S., Sotto, A., Luis, P., Benea, L., Van der Bruggen, B., and Kim, J. (2012). A new outlook on membrane enhancement with nanoparticles: the alternative of ZnO. J. Memb. Sci 389, 155–161. doi: 10.1016/j.memsci.2011.10.025
Ban, Y., Li, Z., Li, Y., Peng, Y., Jin, H., Jiao, W., et al. (2015). Confinement of ionic liquids in nanocages: tailoring the molecular sieving properties of ZIF-8 for membrane-based CO2 capture. Angew. Chem. Int. Ed. 54, 15483–15487. doi: 10.1002/anie.201505508
Bastani, D., Esmaeili, N., and Asadollahi, M. (2013). Polymeric mixed matrix membranes containing zeolites as a filler for gas separation applications: a review. J. Indus. Eng. Chem. 19, 375–393. doi: 10.1016/j.jiec.2012.09.019
Bhagiyalakshmi, M., Hemalatha, P., Ganesh, M., Peng, M. M., and Jang, H. T. (2011). Synthesis of copper exchanged heteropolyacids supported on MCM-48 and its application for CO2 adsorption. J. Indus. Eng. Chem. 17, 628–632. doi: 10.1016/j.jiec.2011.05.010
Biswas, S., and Van Der Voort, P. (2013). A general strategy for the synthesis of functionalised UiO-6 frameworks: characterisation stability, and CO2 adsorption properties. Eur. J. Inorg. Chem. 2013, 2154–2160. doi: 10.1002/ejic.201201228
Car, A., Stropnik, C., and Peinemann, K.- V. (2006). Hybrid membrane materials with different metal-organic frameworks (MOFs) for gas separation. Desalination 200, 424–426. doi: 10.1016/j.desal.2006.03.390
Castro-Muñoz, R., and Fíla, V. (2019). Effect of the ZIF-8 distribution in mixed-matrix membranes based on matrimid® 5218-peg on CO2 separation. Chem. Eng. Technol. 42, 744–752. doi: 10.1002/ceat.201800499
Chen, K., Xu, K., Xiang, L., Dong, X., Han, Y., Wang, C., et al. (2018). Enhanced CO2/CH4 separation performance of mixed-matrix membranes through dispersion of sorption-selective MOF nanocrystals. J. Memb. Sci. 563, 360–370. doi: 10.1016/j.memsci.2018.06.007
Chen, X. Y., Hoang, V.-T., Rodrigue, D., and Kaliaguine, S. (2013). Optimization of continuous phase in amino-functionalized metal–organic framework (MIL-53) based co-polyimide mixed matrix membranes for CO2/CH4 separation. RSC.Adv. 3, 24266–24279. doi: 10.1039/c3ra43486a
Chen, Y., Mu, X., Lester, E., and Wu, T. (2018). High efficiency synthesis of HKUST-1 under mild conditions with high BET surface area and CO2 uptake capacity. Prog. Nat. Sci. Mater. Int. 28, 584–589. doi: 10.1016/j.pnsc.2018.08.002
Cheng, S., Wu, Y., Jin, J., Liu, J., Wu, D., Yang, G., et al. (2019). New multifunctional 3D porous metal–organic framework with selective gas adsorption, efficient chemical fixation of CO2 and dye adsorption. Dalton Trans. 48, 7612–7618. doi: 10.1039/C9DT01249D
Cheng, Y., Wang, X., Jia, C., Wang, Y., Zhai, L., Wang, Q., et al. (2017). Ultrathin mixed matrix membranes containing two-dimensional metal-organic framework nanosheets for efficient CO2/CH4 separation. J. Memb. Sci. 539, 213–223. doi: 10.1016/j.memsci.2017.06.011
Chi, W. S., Sundell, B. J., Zhang, K., Harrigan, D. J., Hayden, S. C., and Smith, Z. P. (2019). Mixed-matrix membranes formed from multi-dimensional metal–organic frameworks for enhanced gas transport and plasticization resistance. ChemSusChem 12, 2355–2360. doi: 10.1002/cssc.201900623
Chung, T.-S., Jiang, L. Y., Li, Y., and Kulprathipanja, S. (2007). Mixed matrix membranes (MMMs) comprising organic polymers with dispersed inorganic fillers for gas separation. Prog. Polym. Sci. 32, 483–507. doi: 10.1016/j.progpolymsci.2007.01.008
Cmarik, G. E., Kim, M., Cohen, S. M., and Walton, K. S. (2012). Tuning the adsorption properties of UiO-66 via ligand functionalization. Langmuir 28, 15606–15613. doi: 10.1021/la3035352
Comesaña-Gándara, B., Chen, J., Bezzu, C. G., Carta, M., Rose, I., Ferrari, M.- C., et al. (2019). Redefining the robeson upper bounds for CO2/CH4 and CO2/N2 separations using a series of ultrapermeable benzotriptycene-based polymers of intrinsic microporosity. Energy Environ. Sci. 12, 2733–2740. doi: 10.1039/C9EE01384A
Cong, H., Radosz, M., Towler, B. F., and Shen, Y. (2007). Polymer–inorganic nanocomposite membranes for gas separation. Sep. Puriff Technol. 55, 281–291. doi: 10.1016/j.seppur.2006.12.017
Dai, Y., Johnson, J. R., Karvan, O., Sholl, D. S., and Koros, W. J. (2012). Ultem®/ZIF and 8 mixed matrix hollow fiber membranes for CO2/N2 separations. J. Memb. Sci. 401–402, 76–82. doi: 10.1016/j.memsci.2012.01.044
Dai, Z., Deng, J., Peng, K.-J., Liu, Y.-L., and Deng, L. (2019). Pebax/PEG grafted CNT hybrid membranes for enhanced CO/N2 separation. Indus. Eng. Chem. Res. 58, 12226–12234. doi: 10.1021/acs.iecr.9b01466
Dai, Z., Noble, R. D., Gin, D. L., Zhang, X., and Deng, L. (2016). Combination of ionic liquids with membrane technology: a new approach for CO2 separation. J. Memb. Sci. 497, 1–20. doi: 10.1016/j.memsci.2015.08.060
Das, R., Ali, M. E. S., Hamid, B. A., Ramakrishna, S., and Chowdhury, Z. Z. (2014). Carbon nanotube membranes for water purification: a bright future in water desalination. Desalination 336, 97–109. doi: 10.1016/j.desal.2013.12.026
Dau, P. V., Tanabe, K. K., and Cohen, S. M. (2012). Functional group effects on metal–organic framework topology. Chem. Commun. 48, 9370–9372. doi: 10.1039/c2cc34938h
Dechnik, J., Gascon, J., Doonan, C. J., Janiak, C., and Sumby, C. J. (2017). Mixed-matrix membranes. Angew. Chem. Int. Ed. 56, 9292–9310. doi: 10.1002/anie.201701109
Dhakshinamoorthy, A., Heidenreich, N., Lenzen, D., and Stock, N. (2017). Knoevenagel condensation reaction catalysed by Al-MOFs with CAU-1 and CAU-10-type structures. CrystEngComm 19, 4187–4193. doi: 10.1039/C6CE02664H
Dong, G., Hou, J., Wang, J., Zhang, Y., Chen, V., and Liu, J. (2016). Enhanced CO2/N2 separation by porous reduced graphene oxide/Pebax mixed matrix membranes. J. Memb. Sci. 520, 860–868. doi: 10.1016/j.memsci.2016.08.059
Dong, G., Li, H., and Chen, V. (2013). Challenges and opportunities for mixed-matrix membranes for gas separation. J. Mater. Chem. A 1, 4610–4630. doi: 10.1039/c3ta00927k
Dong, L., Chen, M., Li, J., Shi, D., Dong, W., Li, X., et al. (2016a). Metal-organic framework-graphene oxide composites: a facile method to highly improve the CO2 separation performance of mixed matrix membranes. J. Memb. Sci. 520, 801–811. doi: 10.1016/j.memsci.2016.08.043
Dong, L., Chen, M., Wu, X., Shi, D., Dong, W., Zhang, H., et al. (2016b). Multi-functional polydopamine coating: simultaneous enhancement of interfacial adhesion and CO2 separation performance of mixed matrix membranes. N. J. Chem. 40, 9148–9159. doi: 10.1039/C6NJ02013E
Dorosti, F., Omidkhah, M., and Abedini, R. (2014). Fabrication and characterization of matrimid/MIL-53 mixed matrix membrane for CO2/CH4 separation. Chem. Eng. Res. Des. 92, 2439–2448. doi: 10.1016/j.cherd.2014.02.018
Du, N., Park, H. B., Dal-Cin, M. M., and Guiver, M. D. (2012). Advances in high permeability polymeric membrane materials for CO2 separations. Energy Environ. Sci. 5, 7306–7322. doi: 10.1039/C1EE02668B
Ebadi Amooghin, A., Omidkhah, M., and Kargari, A. (2015). The effects of aminosilane grafting on NaY zeolite–Matrimid®5218 mixed matrix membranes for CO2/CH4 separation. J. Memb. Sci. 490, 364–379. doi: 10.1016/j.memsci.2015.04.070
Erucar, I., Yilmaz, G., and Keskin, S. (2013). Recent advances in metal–organic framework-based mixed matrix membranes. Chem. Asian J. 8, 1692–1704. doi: 10.1002/asia.201300084
Etxeberria-Benavides, M., David, O., Johnson, T., Łozinska, M. M., Orsi, A., Wright, P. A., et al. (2018). High performance mixed matrix membranes (MMMs) composed of ZIF-94 filler and 6FDA-DAM polymer. J. Memb. Sci. 550, 198–207. doi: 10.1016/j.memsci.2017.12.033
Falcaro, P., Ricco, R., Doherty, C. M., Liang, K., Hill, A. J., and Styles, M. J. (2014). MOF positioning technology and device fabrication. Chem. Soc. Rev. 43, 5513–5560. doi: 10.1039/C4CS00089G
Fan, L., Kang, Z., Shen, Y., Wang, S., Zhao, H., Sun, H., et al. (2018). Mixed matrix membranes based on metal–organic frameworks with tunable pore size for CO2 separation. Cryst. Growth Des. 18, 4365–4371. doi: 10.1021/acs.cgd.8b00307
Fan, W., Liu, X., Wang, X., Li, Y., Xing, C., Wang, Y., et al. (2018). A fluorine-functionalized microporous in-MOF with high physicochemical stability for light hydrocarbon storage and separation. Inorg. Chem. Front. 5, 2445–2449. doi: 10.1039/C8QI00652K
Favvas, E. P., Heliopoulos, N. S., Karousos, D. S., Devlin, E., Papageorgiou, S. K., Petridis, D., et al. (2019). Mixed matrix polymeric and carbon hollow fiber membranes with magnetic iron-based nanoparticles and their application in gas mixture separation. Mater.Chem.Phys. 223, 220–229. doi: 10.1016/j.matchemphys.2018.10.047
Favvas, E. P., Nitodas, S. F., Stefopoulos, A. A., Papageorgiou, S. K., Stefanopoulos, K. L., and Mitropoulos, A. C. (2014). High purity multi-walled carbon nanotubes: preparation, characterization and performance as filler materials in co-polyimide hollow fiber membranes. Sep. Puriff. Technol. 122, 262–269. doi: 10.1016/j.seppur.2013.11.015
Favvas, E. P., and Papageorgiou, S. K. (2013). Polyaniline: A Promising Precursor Material for Membrane Technology Applications, Vol. 2013. Hauppauge, NY: Nova Science Publishers.
Feijani, E. A., Mahdavi, H., and Tavasoli, A. (2015). Poly(vinylidene fluoride) based mixed matrix membranes comprising metal organic frameworks for gas separation applications. Chem. Eng. Res. Des. 96, 87–102. doi: 10.1016/j.cherd.2015.02.009
Feijani, E. A., Mahdavi, H., and Tavassoli, A. (2018). Synthesis and gas permselectivity of CuBTC–GO–PVDF mixed matrix membranes. N. J. Chem. 42, 12013–12023. doi: 10.1039/C8NJ00796A
Freeman, B. D. (1999). Basis of permeability/selectivity tradeoff relations in polymeric gas separation membranes. Macromolecules 32, 375–380. doi: 10.1021/ma9814548
Fu, Q., Ding, J., Wang, W., Lu, J., and Huang, Q. (2017). Carbon dioxide adsorption over amine-functionalized MOFS. Energy Proc. 142, 2152–2157. doi: 10.1016/j.egypro.2017.12.620
Furukawa, H., Cordova, K. E., O'Keeffe, M., and Yaghi, O. M. (2013). The chemistry and applications of metal-organic frameworks. Science 341:1230444. doi: 10.1126/science.1230444
Galizia, M., Chi, W. S., Smith, Z. P., Merkel, T. C., Baker, R. W., and Freeman, B. D. (2017). 50th anniversary perspective: polymers and mixed matrix membranes for gas and vapor separation: a review and prospective opportunities. Macromolecules 50, 7809–7843. doi: 10.1021/acs.macromol.7b01718
Ge, B.-S., Wang, T., Sun, H.-X., Gao, W., and Zhao, H.-R. (2018). Preparation of mixed matrix membranes based on polyimide and aminated graphene oxide for CO2 separation. Polym. Adv. Technol. 29, 1334–1343. doi: 10.1002/pat.4245
Ge, L., Zhou, W., Rudolph, V., and Zhu, Z. (2013). Mixed matrix membranes incorporated with size-reduced Cu-BTC for improved gas separation. J. Mater. Chem. A 1, 6350–6358. doi: 10.1039/c3ta11131h
Ghalei, B., Pournaghshband Isfahani, A., Sadeghi, M., Vakili, E., and Jalili, A. (2018). Polyurethane-mesoporous silica gas separation membranes. Polym. Adv. Technol. 29, 874–883. doi: 10.1002/pat.4198
Goh, P. S., Ismail, A. F., Sanip, S. M., Ng, B. C., and Aziz, M. (2011). Recent advances of inorganic fillers in mixed matrix membrane for gas separation. Sep. Puriff. Technol. 81, 243–264. doi: 10.1016/j.seppur.2011.07.042
Gundersen, M. T., von Solms, N., and Woodley, J. M. (2014). Enzymatically assisted CO2 removal from flue-gas. Energy Proc. 63, 624–632. doi: 10.1016/j.egypro.2014.11.067
Guo, X., Huang, H., Ban, Y., Yang, Q., Xiao, Y., Li, Y., et al. (2015). Mixed matrix membranes incorporated with amine-functionalized titanium-based metal-organic framework for CO2/CH4 separation. J. Memb. Sci. 478, 130–139. doi: 10.1016/j.memsci.2015.01.007
Guo, X., Huang, H., Liu, D., and Zhong, C. (2018). Improving particle dispersity and CO2 separation performance of amine-functionalized CAU-1 based mixed matrix membranes with polyethyleneimine-grafting modification. Chem. Eng. Sci. 189, 277–285. doi: 10.1016/j.ces.2018.06.008
Hamid, M. R. A., and Jeong, H.- K. (2018). Recent advances on mixed-matrix membranes for gas separation: Opportunities and engineering challenges. Korean J. Chem. Eng. 35, 1577–1600. doi: 10.1007/s11814-018-0081-1
Hartlieb, K. J., Holcroft, J. M., Moghadam, P. Z., Vermeulen, N. A., Algaradah, M. M., Nassar, M. S., et al. (2016). CD-MOF: a versatile separation medium. J. Am. Chem. Soc. 138, 2292–2301. doi: 10.1021/jacs.5b12860
Hawes, C. (2019). New CO2 Capture Technology Is Not the Magic Bullet Against Climate Change, in Green Biz. Oakland, CA: Green Biz.
He, R., Cong, S., Wang, J., Liu, J., and Zhang, Y. (2019). Porous graphene oxide/porous organic polymer hybrid nanosheets functionalized mixed matrix membrane for efficient CO2 capture. ACS Appl. Mater. Interfaces 11, 4338–4344. doi: 10.1021/acsami.8b17599
He, Z., Kumar Reddy, K. S., Karanikolos, G., and Wang, K. (2018). “Chapter 5 - CO2/CH4 Separation (natural gas purification) by using mixed matrix membranes,” in Current Trends and Future Developments on (Bio-) membranes, eds A. Basile and E. P. Favvas (Amsterdam: Elsevier), 155–181.
Horcajada, P., Gref, R., Baati, T., Allan, P. K., Maurin, G., Couvreur, P., et al. (2012). Metal–organic frameworks in biomedicine. Chem. Rev. 112, 1232–1268. doi: 10.1021/cr200256v
Hsieh, J. O., Balkus, K. J., Ferraris, J. P., and Musselman, I. H. (2014). MIL-53 frameworks in mixed-matrix membranes. Microp. Mesop. Mater. 196, 165–174. doi: 10.1016/j.micromeso.2014.05.006
Hu, J., Cai, H., Ren, H., Wei, Y., Xu, Z., Liu, H., et al. (2010). Mixed-matrix membrane hollow fibers of Cu3(BTC)2 MOF and polyimide for gas separation and adsorption. Ind. Eng. Chem. Res 49, 12605–12612. doi: 10.1021/ie1014958
Ilyas, A., Muhammad, N., Gilani, M. A., Ayub, K., Vankelecom, I. F. J., and Khan, A. L. (2017). Supported protic ionic liquid membrane based on 3-(trimethoxysilyl)propan-1-aminium acetate for the highly selective separation of CO2. J. Memb. Sci. 543, 301–309. doi: 10.1016/j.memsci.2017.08.071
Ishaq, S., Tamime, R., Bilad, M. R., and Khan, A. L. (2019). Mixed matrix membranes comprising of polysulfone and microporous Bio-MOF-1: preparation and gas separation properties. Sep. Puriff. Technol. 210, 442–451. doi: 10.1016/j.seppur.2018.08.031
Janiak, C., and Vieth, J. K. (2010). MOFs, MILs and more: concepts, properties and applications for porous coordination networks (PCNs). N. J. Chem. 34, 2366–2388. doi: 10.1039/c0nj00275e
Jia, M., Feng, Y., Qiu, J., Zhang, X.-F., and Yao, J. (2019). Amine-functionalized MOFs@GO as filler in mixed matrix membrane for selective CO2 separation. Sep. Puriff. Technol. 213, 63–69. doi: 10.1016/j.seppur.2018.12.029
Jiang, X., Li, S., He, S., Bai, Y., and Shao, L. (2018). Interface manipulation of CO2-philic composite membranes containing designed UiO-66 derivatives towards highly efficient CO2 capture. J. Mate. Chem. A 6, 15064–15073. doi: 10.1039/C8TA03872D
Jiang, Y., Liu, C., Caro, J., and Huang, A. (2019). A new UiO-66-NH2 based mixed-matrix membranes with high CO2/CH4 separation performance. Microp. Mesop. Mater. 274, 203–211. doi: 10.1016/j.micromeso.2018.08.003
Jusoh, N., Yeong, Y. F., Lau, K. K., and Shariff, A. M. (2016). mixed matrix membranes comprising of ZIF-8 nanofillers for enhanced gas transport properties. Proc. Eng. 148, 1259–1265. doi: 10.1016/j.proeng.2016.06.499
Kang, Z., Wang, S., Fan, L., Zhang, M., Kang, W., Pang, J., et al. (2018). In situ generation of intercalated membranes for efficient gas separation. Commun. Chem. 1, 1–8. doi: 10.1038/s42004-017-0002-y
Kapantaidakis, G. C., Kaldis, S. P., Sakellaropoulos, G. P., Chira, E., Loppinet, B., and Floudas, G. (1999). Interrelation between phase state and gas permeation in polysulfone/polyimide blend membranes. J. Polym. Sci. Part B Polym. Phys. 37, 2788–2798. doi: 10.1002/(SICI)1099-0488(19991001)37:<2788::AID-POLB8>3.0.CO;2-L
Karousos, D. S., Labropoulos, A. I., Sapalidis, A., Kanellopoulos, N. K., Iliev, B., Schubert, T. J. S., et al. (2017). Nanoporous ceramic supported ionic liquid membranes for CO2 and SO2 removal from flue gas. Chem. Eng. J. 313, 777–790. doi: 10.1016/j.cej.2016.11.005
Karousos, D. S., Labropoulos, A. I., Tzialla, O., Papadokostaki, K., Gjoka, M., Stefanopoulos, K. L., et al. (2018). Effect of a cyclic heating process on the CO2/N2 separation performance and structure of a ceramic nanoporous membrane supporting the ionic liquid 1-methyl-3-octylimidazolium tricyanomethanide. Sep. Puriff. Technol. 200, 11–22. doi: 10.1016/j.seppur.2018.02.013
Katayama, Y., Bentz, K. C., and Cohen, S. M. (2019). Defect-free MOF-based mixed-matrix membranes obtained by corona cross-linking. ACS Appl. Mater. Interfaces 11, 13029–13037. doi: 10.1021/acsami.9b02539
Keskin, S., and Kizilel, S. (2011). Biomedical applications of metal organic frameworks. Ind. Eng. Chem. Res. 50, 1799–1812. doi: 10.1021/ie101312k
Khan, M. M., Filiz, V., Bengtson, G., Shishatskiy, S., Rahman, M. M., Lillepaerg, J., et al. (2013). Enhanced gas permeability by fabricating mixed matrix membranes of functionalized multiwalled carbon nanotubes and polymers of intrinsic microporosity (PIM). J. Memb. Sci. 436, 109–120. doi: 10.1016/j.memsci.2013.02.032
Khdhayyer, M. R., Esposito, E., Fuoco, A., Monteleone, M., Giorno, L., Jansen, J. C., et al. (2017). Mixed matrix membranes based on UiO-66 MOFs in the polymer of intrinsic microporosity PIM-1. Sep. Puriff. Technol. 173, 304–313. doi: 10.1016/j.seppur.2016.09.036
Kim, N. U., Park, B. J., Lee, J. H., and Kim, J. H. (2019). High-performance ultrathin mixed-matrix membranes based on an adhesive PGMA-co-POEM comb-like copolymer for CO2 capture. J. Mater. Chem. A 7, 14723–14731. doi: 10.1039/C9TA02962A
Kim, S., and Lee, Y. M. (2013). High performance polymer membranes for CO2 separation. Curr. Opin. Chem. Eng. 2, 238–244. doi: 10.1016/j.coche.2013.03.006
Kitao, T., Zhang, Y., Kitagawa, S., Wang, B., and Uemura, T. (2017). Hybridization of MOFs and polymers. Chem. Soc. Rev. 46, 3108–3133. doi: 10.1039/C7CS00041C
Kohl, A. L., and Nielsen, R. B. (1997). “Chapter 2 - alkanolamines for hydrogen sulfide and carbon dioxide removal,” in Gas Purification (5th Edition), eds A. L. Kohl and R. B. Nielsen (Houston: Gulf Professional Publishing), 40–186.
Kontos, A. G., Likodimos, V., Veziri, C. M., Kouvelos, E., Moustakas, N., Karanikolos, G. N., et al. (2014). CO2 captured in zeolitic imidazolate frameworks: raman spectroscopic analysis of uptake and host-guest interactions. ChemSusChem 7, 1696–1702. doi: 10.1002/cssc.201301323
Kueh, B., Kapsi, M., Veziri, C. M., Athanasekou, C., Pilatos, G., Reddy, K. S. K., et al. (2018). Asphaltene-derived activated carbon and carbon nanotube membranes for CO2 separation. Energy Fuels 32, 11718–11730. doi: 10.1021/acs.energyfuels.8b02913
Labropoulos, A., Veziri, C., Kapsi, M., Pilatos, G., Likodimos, V., Tsapatsis, M., et al. (2015). Carbon nanotube selective membranes with subnanometer, vertically aligned pores, and enhanced gas transport properties. Chem. Mater. 27, 8198–8210. doi: 10.1021/acs.chemmater.5b01946
Labropoulos, A. I., Romanos, G. E., Karanikolos, G. N., Katsaros, F. K., Kakizis, N. K., and Kanellopoulos, N. K. (2009). Comparative study of the rate and locality of silica deposition during the CVD treatment of porous membranes with TEOS and TMO. Microporous Mesoporous Mater. 120, 177–185. doi: 10.1016/j.micromeso.2008.08.063
Li, H., Tuo, L., Yang, K., Jeong, H.- K., Dai, Y., He, G., et al. (2016). Simultaneous enhancement of mechanical properties and CO2 selectivity of ZIF-8 mixed matrix membranes: Interfacial toughening effect of ionic liquid. J. Memb. Sci. 511, 130–142. doi: 10.1016/j.memsci.2016.03.050
Li, T., Pan, Y., Peinemann, K.-V., and Lai, Z. (2013). Carbon dioxide selective mixed matrix composite membrane containing ZIF-7 nano-fillers. J. Memb. Sci. 425–426, 235–242. doi: 10.1016/j.memsci.2012.09.006
Li, W., Chuah, C. Y., Nie, L., and Bae, T.-H. (2019). Enhanced CO2/CH4 selectivity and mechanical strength of mixed-matrix membrane incorporated with NiDOBDC/GO composite. J. Ind. Eng. Chem. 74, 118–125. doi: 10.1016/j.jiec.2019.02.016
Li, X., Cheng, Y., Zhang, H., Wang, S., Jiang, Z., Guo, R., et al. (2015). Efficient CO2 capture by functionalized graphene oxide nanosheets as fillers to fabricate multi-permselective mixed matrix membranes. ACS Appl. Mater. Interfaces 7, 5528–5537. doi: 10.1021/acsami.5b00106
Li, Y., Chung, T.-S., Cao, C., and Kulprathipanja, S. (2005). The effects of polymer chain rigidification, zeolite pore size and pore blockage on polyethersulfone (PES)-zeolite a mixed matrix membranes. J. Memb. Sci. 260, 45–55. doi: 10.1016/j.memsci.2005.03.019
Li, Y., Guan, H.-M., Chung, T.-S., and Kulprathipanja, S. (2006). Effects of novel silane modification of zeolite surface on polymer chain rigidification and partial pore blockage in polyethersulfone (PES)–zeolite A mixed matrix membranes. J. Memb. Sci. 275, 17–28. doi: 10.1016/j.memsci.2005.08.015
Li, Z., Xing, X., Meng, D., Wang, Z., Xue, J., Wang, R., et al. (2019). Hierarchical structure with highly ordered macroporous-mesoporous metal-organic frameworks as dual function for CO2 fixation. iScience 15, 514–523. doi: 10.1016/j.isci.2019.05.006
Lin, K.-S., Adhikari, A. K., Su, Y.-H., Shu, C.-W., and Chan, H.-Y. (2012). Synthesis, characterization, and hydrogen storage study by hydrogen spillover of MIL-101 metal organic frameworks. Adsorption 18, 483–491. doi: 10.1007/s10450-012-9438-7
Lin, R., Ge, L., Diao, H., Rudolph, V., and Zhu, Z. (2016). Ionic liquids as the MOFs/polymer interfacial binder for efficient membrane separation. ACS Appl. Mater. Interfaces 8, 32041–32049. doi: 10.1021/acsami.6b11074
Lin, R., Ge, L., Hou, L., Strounina, E., Rudolph, V., and Zhu, Z. (2014). Mixed matrix membranes with strengthened MOFs/polymer interfacial interaction and improved membrane performance. ACS Appl. Mater. Interfaces 6, 5609–5618. doi: 10.1021/am500081e
Lin, R., Ge, L., Liu, S., Rudolph, V., and Zhu, Z. (2015). Mixed-matrix membranes with metal–organic framework-decorated CNT fillers for efficient CO2 separation. ACS Appl. Mater. Interfaces 7, 14750–14757. doi: 10.1021/acsami.5b02680
Lin, R., Villacorta Hernandez, B., Ge, L., and Zhu, Z. (2018). Metal organic framework based mixed matrix membranes: an overview on filler/polymer interfaces. J. Mater. Chem. A 6, 293–312. doi: 10.1039/C7TA07294E
Liu, G., Cadiau, A., Liu, Y., Adil, K., Chernikova, V., Carja, I.-D., et al. (2018a). Enabling fluorinated MOF-based membranes for simultaneous removal of H2S and CO2 from natural gas. Angew. Chem. Int. Ed. 57, 14811–14816. doi: 10.1002/anie.201808991
Liu, G., Chernikova, V., Liu, Y., Zhang, K., Belmabkhout, Y., Shekhah, O., et al. (2018b). Mixed matrix formulations with MOF molecular sieving for key energy-intensive separations. Nat. Mater. 17, 283–289. doi: 10.1038/s41563-017-0013-1
Liu, G., Labreche, Y., Chernikova, V., Shekhah, O., Zhang, C., Belmabkhout, Y., et al. (2018c). Zeolite-like MOF nanocrystals incorporated 6FDA-polyimide mixed-matrix membranes for CO2/CH4 separation. J. Memb. Sci. 565, 186–193. doi: 10.1016/j.memsci.2018.08.031
Luanwuthi, S., Krittayavathananon, A., Srimuk, P., and Sawangphruk, M. (2015). In situ synthesis of permselective zeolitic imidazolate framework-8/graphene oxide composites: rotating disk electrode and Langmuir adsorption isotherm. RSC Adv. 5, 46617–46623. doi: 10.1039/C5RA05950J
Luis, P., Van Gerven, T., and Van der Bruggen, B. (2012). Recent developments in membrane-based technologies for CO2 capture. Prog. Energy Combus. Sci. 38, 419–448. doi: 10.1016/j.pecs.2012.01.004
Ma, J., Ying, Y., Guo, X., Huang, H., Liu, D., and Zhong, C. (2016). Fabrication of mixed-matrix membrane containing metal–organic framework composite with task-specific ionic liquid for efficient CO2 separation. J. Mater. Chem. A 4, 7281–7288. doi: 10.1039/C6TA02611G
MacDowell, N., Florin, N., Buchard, A., Hallett, J., Galindo, A., Jackson, G., et al. (2010). An overview of CO2 capture technologies. Energy Environ. Sci. 3, 1645–1669. doi: 10.1039/c004106h
Mahdi, E. M., and Tan, J.-C. (2016). Mixed-matrix membranes of zeolitic imidazolate framework (ZIF-8)/Matrimid nanocomposite: thermo-mechanical stability and viscoelasticity underpinning membrane separation performance. J. Memb. Sci. 498, 276–290. doi: 10.1016/j.memsci.2015.09.066
Maier, G. (1998). Gas separation with polymer membranes. Angew. Chem. Int. Ed. 37, 2960–2974. doi: 10.1002/(SICI)1521-3773(19981116)37:21<2960::AID-ANIE2960>3.0.CO;2-5
Majeed, S., Fierro, D., Buhr, K., Wind, J., Du, B., Boschetti-de-Fierro, A., et al. (2012). Multi-walled carbon nanotubes (MWCNTs) mixed polyacrylonitrile (PAN) ultrafiltration membranes. J. Memb. Sci. 403–404, 101–109. doi: 10.1016/j.memsci.2012.02.029
Mao, Z., and Sinnott, S. B. (2001). Separation of organic molecular mixtures in carbon nanotubes and bundles: molecular dynamics simulations. J. Phys. Chem. B 105, 6916–6924. doi: 10.1021/jp0103272
Maqsood, K., Mullick, A., Ali, A., Kargupta, K., and Ganguly, S. (2014). Cryogenic carbon dioxide separation from natural gas: a review based on conventional and novel emerging technologies. Rev. Chem. Eng. 30, 453–477. doi: 10.1515/revce-2014-0009
Marti, A. M., Venna, S. R., Roth, E. A., Culp, J. T., and Hopkinson, D. P. (2018). Simple fabrication method for mixed matrix membranes with in situ MOF growth for gas separation. ACS Appl. Mater. Interfaces 10, 24784–24790. doi: 10.1021/acsami.8b06592
Miricioiu, M. G., Iacob, C., Nechifor, G., and V.-,Niculescu, C. (2019). High selective mixed membranes based on mesoporous MCM-41 and MCM-41-NH2 particles in a polysulfone matrix. Front. Chem. 7:332. doi: 10.3389/fchem.2019.00332
Mirqasemi, M. S., Homayoonfal, M., and Rezakazemi, M. (2020). Zeolitic imidazolate framework membranes for gas and water purification. Environ. Chem. Lett. 18, 1–52. doi: 10.1007/s10311-019-00933-6
Moghaddam, Z. S., Kaykhaii, M., Khajeh, M., and Oveisi, A. R. (2018). Synthesis of UiO-66-OH zirconium metal-organic framework and its application for selective extraction and trace determination of thorium in water samples by spectrophotometry. Spectrochim. Acta Part A 194, 76–82. doi: 10.1016/j.saa.2018.01.010
Molavi, H., Shojaei, A., and Mousavi, S. A. (2018). Improving mixed-matrix membrane performance via PMMA grafting from functionalized NH2–UiO-66. J. Mater. Chem. A 6, 2775–2791. doi: 10.1039/C7TA10480D
Mubashir, M., Fong, Y. Y., Leng, C. T., and Keong, L. K. (2018a). Issues and current trends of hollow-fiber mixed-matrix membranes for CO2 separation from N2 and CH4. Chem. Eng. Technol. 41, 235–252. doi: 10.1002/ceat.201700327
Mubashir, M., Yeong, Y. F., Lau, K. K., Chew, T. L., and Norwahyu, J. (2018b). Efficient CO2/N2 and CO2/CH4 separation using NH2-MIL-53(Al)/cellulose acetate (CA) mixed matrix membranes. Sep. Puriff. Technol. 199, 140–151. doi: 10.1016/j.seppur.2018.01.038
Nabais, A. R., Ribeiro, R. P. P. L., Mota, J. P. B., Alves, V. D., Esteves, I. A. A. C., and Neves, L. A. (2018). CO2/N2 gas separation using Fe(BTC)-based mixed matrix membranes: A view on the adsorptive and filler properties of metal-organic frameworks. Sep. Puriff. Technol. 202, 174–184. doi: 10.1016/j.seppur.2018.03.028
Naik, P. V., Wee, L. H., Meledina, M., Turner, S., Li, Y., Van Tendeloo, G., et al. (2016). PDMS membranes containing ZIF-coated mesoporous silica spheres for efficient ethanol recovery via pervaporation. J. Mater. Chem. A 4, 12790–12798. doi: 10.1039/C6TA04700A
Nasir, R., Mukhtar, H., Man, Z., and Mohshim, D. F. (2013). Material advancements in fabrication of mixed-matrix membranes. Chem. Eng. Technol. 36, 717–727. doi: 10.1002/ceat.201200734
Nik, O. G., Chen, X. Y., and Kaliaguine, S. (2012). Functionalized metal organic framework-polyimide mixed matrix membranes for CO2/CH4 separation. J. Memb. Sci. 413–414, 48–61. doi: 10.1016/j.memsci.2012.04.003
Niknam Shahrak, M., Niknam Shahrak, M., Shahsavand, A., Khazeni, N., Wu, X., and Deng, S. (2017). Synthesis, gas adsorption and reliable pore size estimation of zeolitic imidazolate framework-7 using CO2 and water adsorption. Chin. J. Chem. Eng. 25, 595–601. doi: 10.1016/j.cjche.2016.10.012
Nordin, N. A. H., Ismail, A. F., Mustafa, A., Murali, R. S., and Matsuura, T. (2015). Utilizing low ZIF-8 loading for an asymmetric PSf/ZIF-8 mixed matrix membrane for CO2/CH4 separation. RSC Adv. 5, 30206–30215. doi: 10.1039/C5RA00567A
Opanasenko, M., Dhakshinamoorthy, A., Shamzhy, M., Nachtigall, P., Horáček, M., Garcia, H., et al. (2013). Comparison of the catalytic activity of MOFs and zeolites in Knoevenagel condensation. Catal. Sci. Technol. 3, 500–507. doi: 10.1039/C2CY20586F
Ordoñez, M. J. C., Balkus, K. J., Ferraris, J. P., and Musselman, I. H. (2010). Molecular sieving realized with ZIF-8/Matrimid® mixed-matrix membranes. J. Memb. Sci. 361, 28–37. doi: 10.1016/j.memsci.2010.06.017
Pacheco Tanaka, D. A., Llosa Tanco, M. A., Nagase, T., Okazaki, J., Wakui, Y., Mizukami, F., et al. (2006). Fabrication of hydrogen-permeable composite membranes packed with palladium nanoparticles. Adv. Mater. 18, 630–632. doi: 10.1002/adma.200501900
Pera-Titus, M. (2014). Porous inorganic membranes for CO2 capture: present and prospects. Chem. Rev. 114, 1413–1492. doi: 10.1021/cr400237k
Perdikaki, A. V., Labropoulos, A. I., Siranidi, E., Karatasios, I., Kanellopoulos, N., Boukos, N., et al. (2016). Efficient CO oxidation in an ionic liquid-modified, Au nanoparticle-loaded membrane contactor. Chem. Eng. J. 305, 79–91. doi: 10.1016/j.cej.2015.11.111
Perdikaki, A. V., Vangeli, O. C., Karanikolos, G. N., Stefanopoulos, K. L., Beltsios, K. G., Alexandridis, P., et al. (2012). Ionic liquid-modified porous materials for gas separation and heterogeneous catalysis. J. Phys. Chem. C 116, 16398–16411. doi: 10.1021/jp300458s
Perez, E. V., Balkus, K. J., Ferraris, J. P., and Musselman, I. H. (2009). Mixed-matrix membranes containing MOF-5 for gas separations. J. Memb. Sci. 328, 165–173. doi: 10.1016/j.memsci.2008.12.006
Phan, A., Doonan, C. J., Uribe-Romo, F. J., Knobler, C. B., O'Keeffe, M., and Yaghi, O. M. (2010). Synthesis, structure, and carbon dioxide capture properties of zeolitic imidazolate frameworks. Acc. Chem. Res. 43, 58–67. doi: 10.1021/ar900116g
Pierre, A. C. (2012). Enzymatic carbon dioxide capture. ISRN Chem. Eng. 2012:22. doi: 10.5402/2012/753687
Pilatos, G., Vermisoglou, E. C., Perdikaki, A., Devlin, E., Pappas, G. S., Romanos, G. E., et al. (2014). One-step, in situ growth of unmodified graphene – magnetic nanostructured composites. Carbon 66, 467–475. doi: 10.1016/j.carbon.2013.09.023
Pilatos, G., Vermisoglou, E. C., Romanos, G. E., Karanikolos, G. N., Boukos, N., Likodimos, V., et al. (2010). A closer look inside nanotubes: pore structure evaluation of anodized alumina templated carbon nanotube membranes through adsorption and permeability studies. Adv. Funct. Mater. 20, 2500–2510. doi: 10.1002/adfm.200901429
Pokhrel, J., Bhoria, N., Anastasiou, S., Tsoufis, T., Gournis, D., Romanos, G., et al. (2018a). CO2 adsorption behavior of amine-functionalized ZIF-8, graphene oxide, and ZIF-8/graphene oxide composites under dry and wet conditions. Microp. Mesop. Mater. 267, 53–67. doi: 10.1016/j.micromeso.2018.03.012
Pokhrel, J., Bhoria, N., Wu, C., Reddy, K. S. K., Margetis, H., Anastasiou, S., et al. (2018b). Cu- and Zr-based metal organic frameworks and their composites with graphene oxide for capture of acid gases at ambient temperature. J. Solid State Chem. 266, 233–243. doi: 10.1016/j.jssc.2018.07.022
Powell, C. E., and Qiao, G. G. (2006). Polymeric CO2/N2 gas separation membranes for the capture of carbon dioxide from power plant flue gases. J. Memb. Sci. 279, 1–49. doi: 10.1016/j.memsci.2005.12.062
Qaroush, A. K., Assaf, K. I., Bardaweel, S. K., Alsoubani, F., Al-Ramahi, E., Masri, M., et al. (2017). Chemisorption of CO2 by chitosan oligosaccharide/DMSO: organic carbamato–carbonato bond formation. Green Chem. 19, 4305–4314. doi: 10.1039/C7GC01830D
Rather, R. A., and Siddiqui, Z. N. (2019). Sulfonic acid functionalized metal–organic framework (S-IRMOF-3): a novel catalyst for sustainable approach towards the synthesis of acrylonitriles. RSC Adv. 9, 15749–15762. doi: 10.1039/C9RA01012B
Rezakazemi, M., Ebadi Amooghin, A., Montazer-Rahmati, M. M., Ismail, A. F., and Matsuura, T. (2014). State-of-the-art membrane based CO2 separation using mixed matrix membranes (MMMs): an overview on current status and future directions. Prog. Polym. Sci. 39, 817–861. doi: 10.1016/j.progpolymsci.2014.01.003
Rezakazemi, M., Sadrzadeh, M., and Matsuura, T. (2018). Thermally stable polymers for advanced high-performance gas separation membranes. Prog. Energy Combus. Sci. 66, 1–41. doi: 10.1016/j.pecs.2017.11.002
Robeson, L. M. (1991). Correlation of separation factor versus permeability for polymeric membranes. J. Memb. Sci. 62, 165–185. doi: 10.1016/0376-7388(91)80060-J
Robeson, L. M. (2008). The upper bound revisited. J. Memb. Sci. 320, 390–400. doi: 10.1016/j.memsci.2008.04.030
Rodenas, T., Luz, I., Prieto, G., Seoane, B., Miro, H., Corma, A., et al. (2014). Metal–organic framework nanosheets in polymer composite materials for gas separation. Nat. Mater. 14:48. doi: 10.1038/nmat4113
Safaei, M., Foroughi, M. M., Ebrahimpoor, N., Jahani, S., Omidi, A., and Khatami, M. (2019). A review on metal-organic frameworks: Synthesis and applications. TrAC Trends Anal. Chem. 118, 401–425. doi: 10.1016/j.trac.2019.06.007
Safak Boroglu, M., and Yumru, A. B. (2017). Gas separation performance of 6FDA-DAM-ZIF-11 mixed-matrix membranes for H2/CH4 and CO2/CH4 CO2/CH4 separation. Sep. Puriff. Technol. 173, 269–279. doi: 10.1016/j.seppur.2016.09.037
Salehi Maleh, M., and Raisi, A. (2019). Comparison of porous and nonporous filler effect on performance of poly (ether-block-amide) mixed matrix membranes for gas separation applications. Chem. Eng. Res. Des. 147, 545–560. doi: 10.1016/j.cherd.2019.05.038
Sanchez, C., Julián, B., Belleville, P., and Popall, M. (2005). Applications of hybrid organic–inorganic nanocomposites. J. Mater. Chem. 15, 3559–3592. doi: 10.1039/b509097k
Sarfraz, M., and Ba-Shammakh, M. (2018). Harmonious interaction of incorporating CNTs and zeolitic imidazole frameworks into polysulfone to prepare high performance MMMs for CO2 separation from humidified post combustion gases. Br. J. Chem. Eng. 35, 217–228. doi: 10.1590/0104-6632.20180351s20150595
Satheeshkumar, C., Yu, H. J., Park, H., Kim, M., Lee, J. S., and Seo, M. (2018). Thiol–ene photopolymerization of vinyl-functionalized metal–organic frameworks towards mixed-matrix membranes. J. Mater. Chem. A 6, 21961–21968. doi: 10.1039/C8TA03803A
Shahid, S., Nijmeijer, K., Nehache, S., Vankelecom, I., Deratani, A., and Quemener, D. (2015). MOF-mixed matrix membranes: Precise dispersion of MOF particles with better compatibility via a particle fusion approach for enhanced gas separation properties. J. Memb. Sci. 492, 21–31. doi: 10.1016/j.memsci.2015.05.015
Shen, J., Liu, G., Huang, K., Li, Q., Guan, K., Li, Y., et al. (2016). UiO-66-polyether block amide mixed matrix membranes for CO2 separation. J. Memb. Sci. 513, 155–165. doi: 10.1016/j.memsci.2016.04.045
Siddique, H., Rundquist, E., Bhole, Y., Peeva, L. G., and Livingston, A. G. (2014). Mixed matrix membranes for organic solvent nanofiltration. J. Memb. Sci. 452, 354–366. doi: 10.1016/j.memsci.2013.10.012
Smaldone, R. A., Forgan, R. S., Furukawa, H., Gassensmith, J. J., Slawin, A. M. Z., Yaghi, O. M., et al. (2010). Metal–organic frameworks from edible natural products, Angew. Chem. Int. Ed. 49, 8630–8634. doi: 10.1002/anie.201002343
Smith, S. J. D., Ladewig, B. P., Hill, A. J., Lau, C. H., and Hill, M. R. (2015). Post-synthetic Ti exchanged UiO-66 metal-organic frameworks that deliver exceptional gas permeability in mixed matrix membranes. Sci. Rep. 5:7823. doi: 10.1038/srep07823
Smith, Z. P., Bachman, J. E., Li, T., Gludovatz, B., Kusuma, V. A., Xu, T., et al. (2018). Increasing M2(dobdc) loading in selective mixed-matrix membranes: a rubber toughening approach. Chem. Mater. 30, 1484–1495. doi: 10.1021/acs.chemmater.7b02908
Sorribas, S., Zornoza, B., Téllez, C., and Coronas, J. (2014). Mixed matrix membranes comprising silica-(ZIF-8) core–shell spheres with ordered meso–microporosity for natural- and bio-gas upgrading. J. Memb. Sci. 452, 184–192. doi: 10.1016/j.memsci.2013.10.043
Sotto, A., Boromand, A., Balta, S., Kim, J., and Van der Bruggen, B. (2011). Doping of polyethersulfone nanofiltration membranes: antifouling effect observed at ultralow concentrations of TiO2 nanoparticles. J. Mater. Chem. 21, 10311–10320. doi: 10.1039/c1jm11040c
Sridhar, S., Smitha, B., and Aminabhavi, T. M. (2007). Separation of carbon dioxide from natural gas mixtures through polymeric membranes—a review. Sep. Puriff. Rev. 36, 113–174. doi: 10.1080/15422110601165967
Stoeger, J. A., Palomino, M., Agrawal, K. V., Zhang, X., Karanikolos, G. N., Valencia, S., et al. (2012a). Oriented CoSAPO-5 membranes by microwave-enhanced growth on TiO 2-coated porous alumina. Angew. Chem. Int. Ed. 51, 2470–2473. doi: 10.1002/anie.201108042
Stoeger, J. A., Veziri, C. M., Palomino, M., Corma, A., Kanellopoulos, N. K., Tsapatsis, M., et al. (2012b). On stability and performance of highly c-oriented columnar AlPO4-5 and CoAPO-5 membranes. Microp. Mesop. Mater. 147, 286–294. doi: 10.1016/j.micromeso.2011.06.028
Suh, B. L., Lee, S., and Kim, J. (2017). Size-matching ligand insertion in, MOF74 for enhanced CO2 capture under humid conditions. J. Phys. Chem. C 121, 24444–24451. doi: 10.1021/acs.jpcc.7b08239
Sun, H., Wang, T., Xu, Y., Gao, W., Li, P., and Niu, Q. J. (2017). Fabrication of polyimide and functionalized multi-walled carbon nanotubes mixed matrix membranes by in-situ polymerization for CO2 separation. Sep. Puriff. Technol. 177, 327–336. doi: 10.1016/j.seppur.2017.01.015
Surmi, A. (2019). “Process integration and optimization of CO2 removal from natural gas using cryogenic distillation system,” in SPE Middle East Oil and Gas Show and Conference (Society of Petroleum Engineers: Manama), 13.
Surya Murali, R., Ismail, A. F., Rahman, M. A., and Sridhar, S. (2014). Mixed matrix membranes of Pebax-1657 loaded with 4A zeolite for gaseous separations. Sep. Puriff. Technol. 129, 1–8. doi: 10.1016/j.seppur.2014.03.017
Sutrisna, P. D., Hou, J., Zulkifli, M. Y., Li, H., Zhang, Y., Liang, W., et al. (2018). Surface functionalized UiO-66/Pebax-based ultrathin composite hollow fiber gas separation membranes. J. Mater. Chem. A 6, 918–931. doi: 10.1039/C7TA07512J
Syversen, O. A. (2019). Northern Lights project: CCS infrastructure for European CO2 storage and a hydrogen production enabler. (accessed April 25, 2019).
Tanabe, K. K., and Cohen, S. M. (2011). Postsynthetic modification of metal–organic frameworks—a progress report. Chem. Soc. Rev. 40, 498–519. doi: 10.1039/C0CS00031K
Tanh Jeazet, H. B., Sorribas, S., Román-Marín, J. M., Zornoza, B., Téllez, C., Coronas, J., et al. (2016). Increased selectivity in CO2/CH4 separation with mixed-matrix membranes of polysulfone and mixed-MOFs MIL-101(Cr) and ZIF-8. Eur. J. Inorg. Chem. 2016, 4363–4367. doi: 10.1002/ejic.201600190
Thompson, J. A., Vaughn, J. T., Brunelli, N. A., Koros, W. J., Jones, C. W., and Nair, S. (2014). Mixed-linker zeolitic imidazolate framework mixed-matrix membranes for aggressive CO2 separation from natural gas. Microp. Mesop. Mater. 192, 43–51. doi: 10.1016/j.micromeso.2013.06.036
Tien-Binh, N., Rodrigue, D., and Kaliaguine, S. (2018). In-situ cross interface linking of PIM-1 polymer and UiO-66-NH2 for outstanding gas separation and physical aging control. J. Memb. Sci. 548, 429–438. doi: 10.1016/j.memsci.2017.11.054
Tien-Binh, N., Vinh-Thang, H., Chen, X. Y., Rodrigue, D., and Kaliaguine, S. (2015). Polymer functionalization to enhance interface quality of mixed matrix membranes for high CO2/CH4 gas separation. J. Mater. Chem. A 3, 15202–15213. doi: 10.1039/C5TA01597A
Tien-Binh, N., Vinh-Thang, H., Chen, X. Y., Rodrigue, D., and Kaliaguine, S. (2016). Crosslinked MOF-polymer to enhance gas separation of mixed matrix membranes. J. Memb. Sci. 520, 941–950. doi: 10.1016/j.memsci.2016.08.045
Tzialla, O., Veziri, C., Papatryfon, X., Beltsios, K. G., Labropoulos, A., Iliev, B., et al. (2013). Zeolite imidazolate framework-ionic liquid hybrid membranes for highly selective CO2 separation. J. Phys. Chem. C 117, 18434–18440. doi: 10.1021/jp4051287
Vanherck, K., Vankelecom, I., and Verbiest, T. (2011). Improving fluxes of polyimide membranes containing gold nanoparticles by photothermal heating. J. Memb. Sci. 373, 5–13. doi: 10.1016/j.memsci.2011.02.010
Varoon, K., Zhang, X., Elyassi, B., Brewer, D. D., Gettel, M., Kumar, S., et al. (2011). Dispersible exfoliated zeolite nanosheets and their application as a selective membrane. Science 334:72. doi: 10.1126/science.1208891
Venna, S. R., Lartey, M., Li, T., Spore, A., Kumar, S., Nulwala, H. B., et al. (2015). Fabrication of MMMs with improved gas separation properties using externally-functionalized MOF particles. J. Mater. Chem. A 3, 5014–5022. doi: 10.1039/C4TA05225K
Veziri, C., Labropoulos, A., Karanikolos, G. N., and Kanellopoulos, N. K. (2015). “Recent Developments in Membrane Technologies for CO2 Separation,” in Small-Scale Gas to Liquid Fuel Synthesis (Boca Raton, FL: CRC Press), 85–133.
Vicent-Luna, J. M., Gutiérrez-Sevillano, J. J., Anta, J. A., and Calero, S. (2013). Effect of room-temperature ionic liquids on CO2 separation by a Cu-BTC metal–organic framework. J. Phys. Chem. C 117, 20762–20768. doi: 10.1021/jp407176j
Vinoba, M., Bhagiyalakshmi, M., Alqaheem, Y., Alomair, A. A., Pérez, A., and Rana, M. S. (2017). Recent progress of fillers in mixed matrix membranes for CO2 separation: A review. Sep. Puriff. Technol. 188, 431–450. doi: 10.1016/j.seppur.2017.07.051
Vinoba, M., Bhagiyalakshmi, M., Grace, A. N., Chu, D. H., Nam, S. C., Yoon, Y., et al. (2013). CO2 absorption and sequestration as various polymorphs of CaCO3 using sterically hindered amine. Langmuir 29, 15655–15663. doi: 10.1021/la403671y
Vu, D. Q., Koros, W. J., and Miller, S. J. (2003). Mixed matrix membranes using carbon molecular sieves: preparation, I., experimental results. J. Memb. Sci. 211, 311–334. doi: 10.1016/S0376-7388(02)00429-5
Wang, B., Xie, L.-H., Wang, X., Liu, X.-M., Li, J., and Li, J.-R. (2018). Applications of metal–organic frameworks for green energy and environment: new advances in adsorptive gas separation, storage and removal. Green Energy Environ. 3, 191–228. doi: 10.1016/j.gee.2018.03.001
Wang, H., He, S., Qin, X., Li, C., and Li, T. (2018). Interfacial engineering in metal–organic framework-based mixed matrix membranes using covalently grafted polyimide brushes. J. Am. Chem. Soc. 140, 17203–17210. doi: 10.1021/jacs.8b10138
Wang, S., Li, X., Wu, H., Tian, Z., Xin, Q., He, G., et al. (2016). Advances in high permeability polymer-based membrane materials for CO2 separations. Energy Environ. Sci. 9, 1863–1890. doi: 10.1039/C6EE00811A
Wang, S.-G., Cao, D.-B., Li, Y.-W., Wang, J., and Jiao, H. (2005). Chemisorption of CO2 on Nickel Surfaces. J. Phys. Chem. B 109, 18956–18963. doi: 10.1021/jp052355g
Wang, Z., Wang, D., Zhang, S., Hu, L., and Jin, J. (2016). Interfacial design of mixed matrix membranes for improved gas separation performance. Adv. Mater. 28, 3399–3405. doi: 10.1002/adma.201504982
Wu, H., Chua, Y. S., Krungleviciute, V., Tyagi, M., Chen, P., Yildirim, T., et al. (2013). Unusual and highly tunable missing-linker defects in zirconium metal–organic framework UiO-66 and their important effects on gas adsorption. J. Am. Chem. Soc. 135, 10525–10532. doi: 10.1021/ja404514r
Xin, Q., Ouyang, J., Liu, T., Li, Z., Li, Z., Liu, Y., et al. (2015). Enhanced interfacial interaction and CO2 separation performance of mixed matrix membrane by incorporating polyethylenimine-decorated metal–organic frameworks. ACS Appl. Mater. Interfaces 7, 1065–1077. doi: 10.1021/am504742q
Xu, J., and Bhattacharyya, D. (2005). Membrane-based bimetallic nanoparticles for environmental remediation: Synthesis and reactive properties. Environ. Prog. 24, 358–366. doi: 10.1002/ep.10106
Yang, K., Dai, Y., Zheng, W., Ruan, X., Li, H., and He, G. (2019). ZIFs-modified GO plates for enhanced CO2 separation performance of ethyl cellulose based mixed matrix membranesf. Sep. Puriff. Technol. 214, 87–94. doi: 10.1016/j.seppur.2018.04.080
Yu, S. L., Shi, W. X., Lu, Y., and Yang, J. X. (2011). Characterization and anti-fouling performance of nano-Al2O3/PVDF membrane for songhua river raw water filtration. Water Sci. Technol. 64, 1892–1897. doi: 10.2166/wst.2011.138
Zamidi Ahmad, M., Navarro, M., Lhotka, M., Zornoza, B., Téllez, C., Fila, V., et al. (2018). Enhancement of CO2/CH4 separation performances of 6FDA-based co-polyimides mixed matrix membranes embedded with UiO-66 nanoparticles. Sep. Puriff. Technol. 192, 465–474. doi: 10.1016/j.seppur.2017.10.039
Zarshenas, K., Raisi, A., and Aroujalian, A. (2016). Mixed matrix membrane of nano-zeolite NaX/poly (ether-block-amide) for gas separation applications. J. Memb. Sci. 510, 270–283. doi: 10.1016/j.memsci.2016.02.059
Zhang, X., Shen, B., Zhu, S., Xu, H., and Tian, L. (2016). UiO-66 and its Br-modified derivates for elemental mercury removal. J. Hazard. Mater. 320, 556–563. doi: 10.1016/j.jhazmat.2016.08.039
Zhang, Y., Balkus, K. J., Musselman, I. H., and Ferraris, J. P. (2008). Mixed-matrix membranes composed of Matrimid® and mesoporous ZSM-5 nanoparticles. J. Memb. Sci. 325, 28–39. doi: 10.1016/j.memsci.2008.04.063
Zhang, Y., Feng, X., Li, H., Chen, Y., Zhao, J., Wang, S., et al. (2015). Cover picture: photoinduced postsynthetic polymerization of a metal–organic framework toward a flexible stand-alone membrane (angew. chem. int. ed. 14/2015). Angew. Chem. Int. Ed. 54, 4127–4127. doi: 10.1002/anie.201501643
Zhang, Y., Sunarso, J., Liu, S., and Wang, R. (2013). Current status and development of membranes for CO2/CH4 separation: a review. Int. J. Greenhouse Gas Control 12, 84–107. doi: 10.1016/j.ijggc.2012.10.009
Zhao, J., Xie, K., Liu, L., Liu, M., Qiu, W., and Webley, P. A. (2019). Enhancing plasticization-resistance of mixed-matrix membranes with exceptionally high CO2/CH4 selectivity through incorporating ZSM-25 zeolite. J. Memb. Sci. 583, 23–30. doi: 10.1016/j.memsci.2019.03.073
Zhao, Y., Jung, B. T., Ansaloni, L. W, and Ho, S. W. (2014). Multiwalled carbon nanotube mixed matrix membranes containing amines for high pressure CO2/H2 separation. J. Memb. Sci. 459, 233–243. doi: 10.1016/j.memsci.2014.02.022
Zhao, Y., Zhao, D., Kong, C., Zhou, F., Jiang, T., and Chen, L. (2019). Design of thin and tubular MOFs-polymer mixed matrix membranes for highly selective separation of H2 and CO2. Sep. Puriff. Technol. 220, 197–205. doi: 10.1016/j.seppur.2019.03.037
Zheng, W., Ding, R., Yang, K., Dai, Y., Yan, X., and He, G. (2019). ZIF-8 nanoparticles with tunable size for enhanced CO2 capture of pebax based MMMs. Sep. Puriff. Technol. 214, 111–119. doi: 10.1016/j.seppur.2018.04.010
Zhu, H., Wang, L., Jie, X., Liu, D., and Cao, Y. (2016). Improved interfacial affinity and CO2 separation performance of asymmetric mixed matrix membranes by incorporating postmodified MIL-53(Al). ACS Appl. Mater. Interfaces 8, 22696–22704. doi: 10.1021/acsami.6b07686
Zornoza, B., Martinez-Joaristi, A., Serra-Crespo, P., Tellez, C., Coronas, J., Gascon, J., et al. (2011a). Functionalized flexible MOFs as fillers in mixed matrix membranes for highly selective separation of CO2 from CH4 at elevated pressures. Chem. Commun. 47, 9522–9524. doi: 10.1039/c1cc13431k
Zornoza, B., Téllez, C., and Coronas, J. (2011b). Mixed matrix membranes comprising glassy polymers and dispersed mesoporous silica spheres for gas separation. J. Memb. Sci. 368, 100–109. doi: 10.1016/j.memsci.2010.11.027
Keywords: membranes, permeability, selectivity, MOF, CO2, separation, polymers, mixture
Citation: Muthukumaraswamy Rangaraj V, Wahab MA, Reddy KSK, Kakosimos G, Abdalla O, Favvas EP, Reinalda D, Geuzebroek F, Abdala A and Karanikolos GN (2020) Metal Organic Framework — Based Mixed Matrix Membranes for Carbon Dioxide Separation: Recent Advances and Future Directions. Front. Chem. 8:534. doi: 10.3389/fchem.2020.00534
Received: 23 October 2019; Accepted: 25 May 2020;
Published: 03 July 2020.
Edited by:
Yatao Zhang, Zhengzhou University, ChinaReviewed by:
Gongping Liu, Nanjing Tech University, ChinaXuezhong He, Norwegian University of Science and Technology, Norway
Copyright © 2020 Muthukumaraswamy Rangaraj, Wahab, Reddy, Kakosimos, Abdalla, Favvas, Reinalda, Geuzebroek, Abdala and Karanikolos. This is an open-access article distributed under the terms of the Creative Commons Attribution License (CC BY). The use, distribution or reproduction in other forums is permitted, provided the original author(s) and the copyright owner(s) are credited and that the original publication in this journal is cited, in accordance with accepted academic practice. No use, distribution or reproduction is permitted which does not comply with these terms.
*Correspondence: Georgios N. Karanikolos, Z2Vvcmdpb3Mua2FyYW5pa29sb3MmI3gwMDA0MDtrdS5hYy5hZQ==