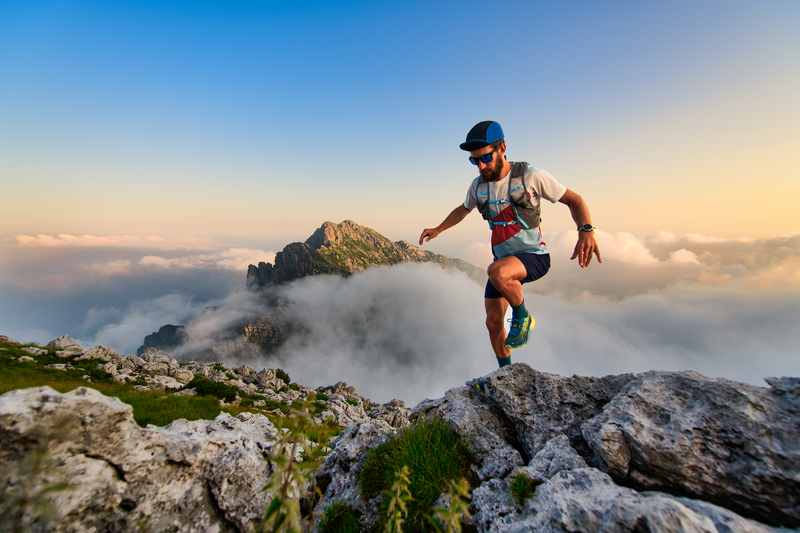
94% of researchers rate our articles as excellent or good
Learn more about the work of our research integrity team to safeguard the quality of each article we publish.
Find out more
MINI REVIEW article
Front. Chem. , 15 May 2020
Sec. Electrochemistry
Volume 8 - 2020 | https://doi.org/10.3389/fchem.2020.00415
This article is part of the Research Topic Low-Cost Electrochemical Energy Storage Devices: Zinc-/Sodium-Ion Batteries View all 9 articles
Clean energy and environmental protection are critical to the sustainable development of human society. The numerous emerged electrode materials for energy storage devices offer opportunities for the development of capacitive deionization (CDI), which is considered as a promising water treatment technology with advantages of low cost, high energy efficiency, and wide application. Conventional CDI based on porous carbon electrode has low salt removal capacity which limits its application in high salinity brine. Recently, the faradaic electrode materials inspired by the researches of sodium-batteries appear to be attractive candidates for next-generation CDI which capture ions by the intercalation or redox reactions in the bulk of electrode. In this mini review, we summarize the recent advances in the development of various faradaic materials as CDI electrodes with the discussion of possible strategies to address the problems present.
Clean energy and environmental protection are critical to the sustainable development of human society (Panwar et al., 2011; Shahzad et al., 2017; Liu et al., 2019a). Over the past decades, great efforts have been devoted to the development of various electrochemical energy storage devices, such as lithium-ion batteries sodium-ion batteries (SIBs) (Zhang et al., 2018; Zubi et al., 2018), SIBs (Chao et al., 2018; Delmas, 2018; Fang et al., 2018), metal-air batteries (Zhang X. et al., 2016), and supercapacitors (Raza et al., 2018; Xu et al., 2018; Han Y. et al., 2019). On the other hand, water desalination has played an essential role in resolving the water crisis. Compared with other existing desalination technologies, capacitive deionization (CDI) has the key advantages of low cost, high energy efficiency, easy regeneration, and wide application (Suss et al., 2015). Beyond the desalination of brackish water, CDI has been also applied in wastewater remediation (Wang Z. J. et al., 2017), water softening (Wang and Lin, 2019), the removal of heavy metals (Huang et al., 2016) and organic pollutants (Bharath et al., 2017), as well as ion separation (Shi et al., 2019a).
The concept of CDI was reported as early as 1960s, but the term of CDI was proposed by Farmer et al. until 1996 (Farmer et al., 1996). In recent decades, the research interests in CDI have exponentially grown with the advances of novel electrode materials, cell configurations, and better understanding of ion electrosorption behaviors (Oladunni et al., 2018; Tang et al., 2019). CDI has many similarities with electrochemical energy storage systems, especially supercapacitors, sodium ion batteries (Kim et al., 2014; Fang et al., 2019), etc. These two fields can find common ground in related researches, such as the design of electrode materials, interfacial electrochemistry, and the ion diffusion process in micro-/nano-structured materials (Shi et al., 2019c).
As shown in Figure 1A, CDI cells are typically composed of two parallel porous electrodes with a channel in between for water flow. The most widely used electrode for traditional CDI is carbon materials. When a voltage difference of generally ~1 V is applied across the CDI cell, ions are capacitively stored in the porous electrode by forming electrical double layers (EDLs), thereby removing the salt ions from the feed water. When the external power supply is removed or the polarity is reversed, the electrode can be regenerated with the adsorbed ions released to the brine. It is worth mentioning that recently some newly developed configurations of CDI cells have adopted faradaic electrode materials used in batteries, such as hybrid CDI (Lee et al., 2014). Differing from carbon electrodes, faradaic materials store ions by intercalation or redox reactions (inset in Figure 1A) (Pasta et al., 2012). The desalination/regeneration process of CDI is very similar to the charge/discharge process of energy storage devices (Figure 1B). In this mini review, we briefly introduce the key features of CDI and aqueous electrochemical energy storage devices in common, in terms of principle and design strategy of electrode materials. In particular, possible strategies and emerging trends to address the challenges present in CDI were discussed. This review may provide a new insight to the development of novel faradaic electrode materials for the next-generation CDI system.
Figure 1. (A) Schematic diagram of capacitive deionization (CDI) structure. (B) Schematic diagram of Sodium-ion battery (SIB) structure.
CDI shares a lot of electrode materials with electrochemical energy storage devices. The CDI and energy storage performances of the representative electrode materials are summarized in Table 1. Among these materials, carbonaceous materials have been widely used in electrochemical sodium storage devices, such as SIBs and sodium ion capacitors (Balogun et al., 2016). They are also the most commonly explored electrodes for conventional CDI, which is based on the EDLs effect of carbon materials to capture ions from salty water (Noonan et al., 2018). Carbon electrode-based CDI has a desalination capacity of about 0.7–14.3 mg g−1 (Porada et al., 2013). Recently developed nano-carbon electrodes, such as graphene (Xu et al., 2015a; Zhang et al., 2019), carbon with functional groups (Gao et al., 2016), and hierarchical carbon materials (Gao et al., 2015), can increase the desalination capacity up to 57.13 mg g−1 (Li et al., 2017).
CDI performance of graphene was first reported by Li et al. showing a relatively low desalination capacity of 4.29 mg g−1 at 2.0 V in 27 mg L−1 NaCl solution (Li et al., 2010). Later works found that building three-dimensional (3D) structure is an effective approach to alleviate the agglomeration of graphene sheets and shorten the diffusion distance (Xu et al., 2015a). For example, Shi et al. developed a hierarchical structure with the interconnected macropores within the graphene networks and nanopores on graphene sheets (Shi et al., 2016, 2019b). A high desalination capacity of 17.1 mg g−1 was achieved at a cell potential of 1.6 V.
Metal-organic frameworks (MOFs), a new family of porous crystalline materials, have shown great promise as precursors of carbon-based materials. MOF-derived porous carbon materials have attracted much attentions in both energy storage devices and CDI, due to their large specific surface area and tunable microscopic structures (Xu et al., 2016; Shi et al., 2018). MOF-derived porous carbon was studied as the electrode of CDI for the first time by Pan et al. in 2015. Porous carbon obtained by direct carbonization of ZIF-8 exhibited a desalination capacity of 13.86 mg g−1 at a cell potential of 1.2 V in 500 mg L−1 NaCl solution. Since then, a large amount of MOF-derived carbon and their composites have been developed with further improved performance (Liu et al., 2015; Gao et al., 2019).
In addition, heteroatom doping can improve the wettability and conductivity of electrode materials, which is often adopted as a surface modification approach. Xu et al. synthesized a nitrogen-doped graphene sponge (NGS) as CDI electrode (Xu et al., 2015b). The NGS electrode with high specific surface area and superior electron mobility exhibited an ultrahigh desalination capacity of 21.0 mg g−1 in 500 mg L−1 NaCl solution. In order to get an insight into the mechanism of surface modification, Landon and co-workers synthesized functionalized carbon electrode and used it as a model to investigate the relationship between chemical surface charge and desalination performance (Gao et al., 2016). It was found that carbon electrodes with the optimized chemical surface charge can extend the working voltage window, thereby significantly increasing the desalination capacity of the CDI electrode.
Up to now, a large variety of investigations on structural design and surface modification of carbon-based materials have been conducted. There are many researchers engaged in the design, development, preparation, modification, and application of carbon materials. Undoubtedly, performance of CDI can be further enhanced via a further understanding of interfacial and kinetic properties of porous carbon materials in future.
Inspired by the energy storage systems, various faradaic electrode materials have been actively explored for water desalination in recent years. These materials allow the intercalation or redox reactions, which store ions in the bulk of electrode rather than capacitively stored on the surface. Therefore, they are promising for higher salt removal capability compared with conventional carbon materials (Cai et al., 2018).
Sodium manganese oxide, a layered structured material described as NaxMnO2 (0 < x ≤ 1), has been intensively investigated in SIBs due to their high capacity and low cost. The pioneering work on the application of faradaic electrodes in water desalination was reported in 2012 (Pasta et al., 2012). Pasta et al. proposed a concept of desalination battery which is comprised by a Na2−xMn5O10 nanorod as positive electrode and Ag/AgCl as negative electrode. However, this system was mainly served as an energy storage device, salt removal only performed at static with a few hundreds of microliters of electrolyte. More recently, a hybrid CDI system was developed by combining a battery electrode (Na4Mn9O18) with a capacitive electrode (activated carbon) demonstrating improved desalination capacity of 31.2 mg g−1 as compared to traditional carbon-based CDI system (Lee et al., 2014).
Recently, sodium superionic conductors (NASICONs), such as NaTi2(PO4)3 (NTP) (Huang et al., 2017, 2019; Guo et al., 2018; Wang et al., 2018; Zhang et al., 2020) and Na3V2(PO4)3 (NVP) (Cao J. L. et al., 2019; Cao X. X. et al., 2019), have been considered as promising cathode materials for sodium ion battery due to their high theoretical specific capacity and high Na+ conductivity. Yang and co-workers fabricated a hybrid CDI cell with NaTi2(PO4)3/rGO as positive electrode and activated carbon (AC) as negative electrode. During the desalination process, sodium ions are inserted into the NTP electrode, while chloride ions are adsorbed on the EDLs of AC electrode. An ultrahigh desalination capacity of 140 mg g−1 in the first cycle was achieved at a current density of 100 mA g−1, decreasing to 120 mg g−1 after 100 cycle.
Na3V2(PO4)3 exhibits a high theoretical capacity (117.6 mAh g−1) and good thermal stability. Cao et al. applied Na3V2(PO4)3@C as a novel faradaic electrode in a hybrid CDI system showing a desalination capacity of 137.30 mg g−1 at a constant voltage of 1.0 V in 100 mM NaCl solution (Cao J. L. et al., 2019). Similar work was reported by Yang and co-workers, who synthesized a conductive polydopamine coated NVP (NVP@C) (Zhao et al., 2018). A faradaic CDI system was fabricated by coupling NVP@C as Na storage electrode and AgCl as Cl storage electrode via conversion reactions. Soon after, they reported a binder-free electrode for CDI, which is prepared from Na3V2(PO4)3/graphene hybrid aerogel (Zhao W. Y. et al., 2019).
Due to different sodium capture process of battery electrode as compared to carbon-based materials, battery electrode-based CDI can achieve higher desalination capacity and is also suitable to salty water with high concentration. Another distinct advantage of using battery electrode for CDI is that the co-ion effects of carbon electrode can be eliminated. Therefore, ion-exchange-membrane-free CDI can be achieved which are more compact and possible for miniature-size design. It is worth to mention that composite materials may be considered in future research. Based on the studies of SIBs, reasonable composite materials can improve the efficiency of ion and electron transport (Xu et al., 2017; Cai et al., 2018; Zhou et al., 2018). This strategy may be applied to enhance salt removal performance in CDI. Especially, some composite electrodes may be capable of combining the faradaic and EDLs adsorption principles to increase the amount of salt removal (Yue et al., 2019a). In addition, the concept of integrated and/or self-supported electrodes in SIBs can also be applied to the electrode design of CDI, which makes the preparation process simple and is possible for large-scale electrode production.
Prussian blue (PB) and its analogs (PBAs) with a general formula of NaxM1[M2(CN)6]y·nH2O (M: transition metal ions) are a family of coordination compounds with open framework structure. They have been widely investigated as alternative host materials in sodium ion capacitors, SIBs, and zinc-ion batteries in recent years (Qian et al., 2018). Benefiting from their unique large ionic channels and facile preparation, PB-based materials are promising electrode materials for CDI.
Lee et al. first designed a novel desalination system consisting two PBAs electrodes, i.e., the NaxNi[Fe(CN)6] and NaxFe[Fe(CN)6] electrodes with an anion exchange membrane in between. Based on the working principle of rocking chair battery, this system achieved efficient desalination during charging and discharging, with a desalination capacity of 59.9 mg g−1 (Lee et al., 2017a).
Soon after, Guo et al. assembled a hybrid CDI cell using FeFe(CN)6 crystals as positive electrode and AC as negative electrode. The PB crystals prepared by a simple chemical precipitation method undergo reversible Na+ insertion/extraction process as the following redox reaction.
The hybrid CDI cell delivered a high removal capacity of 101.7 mg g−1 at a current density of 0.125 mA g−1 and good cycling stability (Guo et al., 2017).
Later, Choi et al. conducted a similar study to synthesize a CuFe-PBA framework (K0.03Cu[Fe(CN)6]0.65·0.43H2O) which is coupled with porous carbon in a hybrid CDI cell. Besides Na+ ions, effective removal of various cations such as K+, Mg2+, and Ca2+ was also demonstrated, showing potential application toward desalination of complex feed waters (Choi et al., 2018).
Along with the exploration of new PB crystals, various strategies of structural and morphological modifications have also been developed to further improve their CDI performance. For example, to enhance the electronic conductivity of the PBs, Vafakhah et al. reported a PB/rGO aerogel by embedding PB nanocubes in rGO 3D networks and applied them in a hybrid CDI system. Compared with pristine PB particles, the PB/rGO composite exhibited a noticeably higher desalination capacity of 130 mg g−1 at the current density of 100 mA g−1 and higher rate capability. At the same time, the reversible intercalation/de-intercalation mechanism of PB-based electrode was further confirmed by in-situ XRD analysis (Vafakhah et al., 2019). In addition, Zhao et al. designed a core–shell structured CuFe-PBA@NiFe-PBA, which combines the high capacity of CuFe-PBA core with highly stable NiFe-PBA shell. This core-shelled PBA material delivered 71.8 ± 2.0 mg g−1 at 0.5 mA cm−2, which is over 32% higher than those of CuFe-PBA and NiFe-PBA, respectively (Zhao Y. B. et al., 2019).
Although their potential advantages, PB-based materials suffer from unsatisfactory performance which is mainly caused by a large amount of lattice defects and coordinated water. The defects largely reduce the utilization of redox-active sites in the PB framework that allow sodium storage, thus leading to a low salt removal capacity. Therefore, in the future research of PB-based CDI electrodes, efforts can be made to develop high-crystallinity PB electrode materials. In addition, related researches on SIBs have revealed that particle size of PBA crystals has significant influence on ion diffusion rate and the rate performance. This finding is also important for the desalination rate of CDI. Unfortunately, nano-sized PB normally has higher surface defect density, a possible solution is to rationally construct composite electrode with the integrity of crystal structure of PBA maintaining.
The breakthrough of graphene has promoted intensive researches on two-dimensional (2D) nanomaterials in past decades. The emerging new types of 2D nanomaterials and their composites have been widely used in various fields including electrochemical energy storage (Liu et al., 2019b, 2020; Zhao H. W. et al., 2019; Wu et al., 2020). MXene, first reported by Naguib et al., is a family of layer structured metal carbide/nitride which shares a general formula of Mn+1AXn (M is early transition metal, A is an element of group IIIA and IVA, X is C and/or N). After selective etching of A layers, the exfoliated MXene layer with ultrathin thickness is a promising 2D material (Naguib et al., 2011). Compared with other 2D materials such as graphene, MXene has unique advantages of abundant functional groups and tunable properties owing to various chemical composition and controllable interlayer space (VahidMohammadi et al., 2019). MXenes have been proved promising for efficient sodium storage, especially in SIBs and capacitors, delivering specific capacities of 30–76.2 mAh g−1 (Zhu et al., 2017; Guan et al., 2020).
Srimuk et al. first reported the application of MXene in a symmetrical CDI cell prepared by direct casting of Ti3C2 onto a porous separator without addition of binder. The fabricated CDI cell demonstrated a desalinization capacity of more than 13 mg g−1 in 5 mM NaCl solution, which is comparable to most of conventional carbon materials for CDI (Srimuk et al., 2016). The relatively low capacity maybe due to the restacking of MXene sheets.
Later, Bao et al. prepared an aerogel-like porous Ti3C2Tx MXene electrode by vacuum freeze-drying process. Impressively, the porous MXene demonstrated a high volumetric salt removal capacity of 118 mg cm−3 which is attributed to effective utilization of the 2D surfaces (Bao et al., 2018). Similarly, Ma et al. prepared a free-standing Ti3C2Tx MXene film as binder-free electrode for CDI (Sriramulu and Yang, 2019). Recently, it was found that the introduction of heteroatom dopants to MXene can effectively improve the electronic conductivity and reduce the risk of restacking as well (Amiri et al., 2020).
As a CDI electrode, MXene has the following competitive advantages. First, MXene has intrinsically high volumetric capacitance, enabling high desalination capacity. Second, MXene is accessible to other types of ions beyond Na, which makes it possible for applications in real feedwater (Lukatskaya et al., 2013; Come et al., 2015). In addition, as a typical 2D layered material, MXene has the common feature of strong attraction between layers. Therefore, it can be used as a binder-free electrode, which has appealing advantage for miniature and portable CDI devices. However, most of etching processes of MXene involve the toxic HF which limits the large-scale preparation of MXene in CDI. In future, the etching method need to be further developed to fulfill the requirements of commercial applications.
Another attractive 2D material is few-layered MoS2 sheets or flakes, which have been insensitively studied as anode material for SIBs (Sun et al., 2018). Recently, CDI performance of chemically exfoliated MoS2 (ce-MoS2) nanosheets were investigated by Cao and co-workers (Xing et al., 2017). The ce-MoS2 nanosheets demonstrated a good cycling stability and a desalination capacity of 8.81 mg g−1 at 1.2 V in 400 mM NaCl solution. MoS2 as CDI electrode cannot give a satisfactory performance, possibly due to the poor conductivity and low accessible active sites. Shortly afterward, various low dimensional nanomaterials such as CNT (Srimuk et al., 2017), graphene (Han J. L. et al., 2019; Peng et al., 2020), or g-C3N4 (Tian et al., 2020) were employed to further improve the performance of MoS2.
MoS2 can be obtained from a naturally abundant mineral by a simple exfoliation method, which is a low-cost electrode for CDI. However, it is difficult to control the number of layers of the obtained MoS2 sheets, which greatly affects the ion diffusion process and leads to rapid capacity fading. Further work is recommended to investigate the relationship between thickness and desalination performance of MoS2.
In addition, other commonly used battery electrode materials have also been applied in CDI, such as Na2FeP2O7 (Kim et al., 2016), multi walled CNT/hV2O5 composite (Lee et al., 2017b), and MnO2 (Hand and Cusick, 2017).
In summary, CDI is an emerging water treatment technology which shares many similarities with electrochemical energy storage systems. In the near future, the CDI research community is expected to grow larger with the development of novel electrode materials and cell architectures. Obviously, CDI has many advantages over other desalination technologies, including mild operation conditions with no high pressure and heat source required, simple cell configuration, low-cost and high energy efficiency for low salinity streams. However, it is still facing great challenges of application in real feedwaters. More efforts can be made in future to the investigation of electrode materials capable of capturing multivalent ions. Moreover, the adsorption behavior of soluble organics in water is more complicated, which may cause biofouling and affect the desalination performance and service life of CDI.
Another future direction of CDI technology may be the combination with renewable energy resources, such as solar energy and wind energy, to reduce the energy consumption. For example, a recent work demonstrated a flow-electrode CDI system with low energy consumption (Desai et al., 2018). It is also promising to develop miniaturized and portable desalination devices which may have potential applications in exploration and disaster-affected area.
The adsorption of chloride ions will also affect the desalination performance of the whole device. Although silver has been investigated for Cl− ion storage (Yue et al., 2019b), high cost of silver limits its large-scale application in water treatment. Other types of materials, such as bismuth, may be considered. Moreover, researchers may also pay attention to relevant achievements in the recent developed chloride ion battery (Pasta et al., 2012; Nam and Choi, 2017).
In addition, the salt removal mechanism of many recently developed battery electrode materials is different from the generally accepted EDLs principle. Therefore, combining in-situ technology to reveal the correlation between the structure of battery electrode materials and its corresponding desalination performance, as well as the desalination mechanism of novel battery materials is another interesting research direction.
WS, XG, JM, XQ, WL, FW, HL, ZZ, JS, and XC contributed to the discussion and writing in this work.
This work was supported by the financial support from the project of Ningxia key RD plan (218BEE03013), National Natural Science Foundation of China (51702286, 51972286, 21905246), Zhejiang Provincial Natural Science Foundation of China (LR19E020003, LQ20B010011), and General Scientific Research Project of the Department of Education of Zhejiang Province, China (Y201839638, Y201941079), and the Thousand Talent Program and Qianjiang Scholars program of Zhejiang Province in China.
The authors declare that the research was conducted in the absence of any commercial or financial relationships that could be construed as a potential conflict of interest.
Amiri, A., Chen, Y. J., Teng, C. B., and Naraghi, M. (2020). Porous nitrogen-doped MXene-based electrodes for capacitive deionization. Energy Storage Mater. 25, 731–739. doi: 10.1016/j.ensm.2019.09.013
Balogun, M. S., Luo, Y., Qiu, W. T., Liu, P., and Tong, Y. X. (2016). A review of carbon materials and their composites with alloy metals for sodium ion battery anodes. Carbon N.Y. 98, 162–178. doi: 10.1016/j.carbon.2015.09.091
Bao, W. Z., Tang, X., Guo, X., Choi, S., Wang, C. Y., Gogotsi, Y., et al. (2018). Porous cryo-dried mxene for efficient capacitive deionization. Joule 2, 778–787. doi: 10.1016/j.joule.2018.02.018
Bharath, G., Alhseinat, E., Ponpandian, N., Khan, M. A., Siddiqui, M. R., Ahmed, F., et al. (2017). Development of adsorption and electrosorption techniques for removal of organic and inorganic pollutants from wastewater using novel magnetite/porous graphene-based nanocomposites. Sep. Purif. Technol. 188, 206–218. doi: 10.1016/j.seppur.2017.07.024
Cai, Y. S., Cao, X. X., Luo, Z. G., Fang, G. Z., Liu, F., Zhou, J., et al. (2018). Caging Na3V2(PO4)2F3 microcubes in cross-linked graphene enabling ultrafast sodium storage and long-term cycling. Adv. Sci. 5:10. doi: 10.1002/advs.201800680
Cao, J. L., Wang, Y., Wang, L., Yu, F., and Ma, J. (2019). Na3V2(PO4)3@C as faradaic electrodes in capacitive deionization for high-performance desalination. Nano Lett. 19, 823–828. doi: 10.1021/acs.nanolett.8b04006
Cao, X. X., Pan, A. Q., Yin, B., Fang, G. Z., Wang, Y. P., Kong, X. Z., et al. (2019). Nanoflake-constructed porous Na3V2(PO4)3/C hierarchical microspheres as a bicontinuous cathode for sodium-ion batteries applications. Nano Energy 60, 312–323. doi: 10.1016/j.nanoen.2019.03.066
Chao, D. L., Ouyang, B., Liang, P., Huong, T. T. T., Jia, G. C., Huang, H., et al. (2018). C-Plasma of hierarchical graphene survives SnS bundles for ultrastable and high volumetric Na-Ion storage. Adv. Mater. 30:6. doi: 10.1002/adma.201804833
Chodankar, N. R., Dubal, D. P., Gund, G. S., and Lokhande, C. D. (2015). Flexible all-solid-state MnO2 thin films based symmetric supercapacitors. Electrochim. Acta 165, 338–347. doi: 10.1016/j.electacta.2015.02.246
Choi, B. G., Yang, M., Hong, W. H., Choi, J. W., and Huh, Y. S. (2012). 3D macroporous graphene frameworks for supercapacitors with high energy and power densities. ACS Nano 6, 4020–4028. doi: 10.1021/nn3003345
Choi, S., Chang, B., Kim, S., Lee, J., Yoon, J., and Choi, J. W. (2018). Battery electrode materials with omnivalent cation storage for fast and charge-efficient ion removal of asymmetric capacitive deionization. Adv. Funct. Mater. 28:9. doi: 10.1002/adfm.201802665
Come, J., Black, J. M., Lukatskaya, M. R., Naguib, M., Beidaghi, M., Rondinone, A. J., et al. (2015). Controlling the actuation properties of MXene paper electrodes upon cation intercalation. Nano Energy 17, 27–35. doi: 10.1016/j.nanoen.2015.07.028
Delmas, C. (2018). Sodium and sodium-ion batteries: 50 years of research. Adv. Energy Mater. 8:9. doi: 10.1002/aenm.201703137
Desai, D., Beh, E. S., Sahu, S., Vedharathinam, V., van Overmeere, Q., de Lannoy, C. F., et al. (2018). Electrochemical desalination of seawater and hypersaline brines with coupled electricity storage. Acs Energy Lett. 3, 375–379. doi: 10.1021/acsenergylett.7b01220
Dong, J., Zhang, G. M., Wang, X. G., Zhang, S., and Deng, C. (2017). Cross-linked Na2VTi(PO4)3@C hierarchical nanofibers as high-performance bi-functional electrodes for symmetric aqueous rechargeable sodium batteries. J. Mater. Chem. A 5, 18725–18736. doi: 10.1039/C7TA05361D
Fang, G. Z., Wang, Q. C., Zhou, J., Lei, Y. P., Chen, Z. X., Wang, Z. Q., et al. (2019). Metal organic framework-templated synthesis of bimetallic selenides with rich phase boundaries for sodium-ion storage and oxygen evolution reaction. ACS Nano 13, 5635–5645. doi: 10.1021/acsnano.9b00816
Fang, G. Z., Wu, Z. X., Zhou, J., Zhu, C. Y., Cao, X. X., Lin, T. Q., et al. (2018). Observation of pseudocapacitive effect and fast ion diffusion in bimetallic sulfides as an advanced sodium-ion battery anode. Adv. Energy Mater. 8:10. doi: 10.1002/aenm.201703155
Farmer, J. C., Fix, D. V., Mack, G. V., Pekala, R. W., and Poco, J. F. (1996). Capacitive deionization of NaCl and NaNO3 solutions with carbon aerogel electrodes. J. Electrochem. Soc. 143, 159–169. doi: 10.1149/1.1836402
Gao, T., Li, H. B., Zhou, F., Gao, M. M., Liang, S., and Luo, M. (2019). Mesoporous carbon derived from ZIF-8 for high efficient electrosorption. Desalination 451, 133–138. doi: 10.1016/j.desal.2017.06.021
Gao, X., Omosebi, A., Landon, J., and Liu, K. L. (2015). Surface charge enhanced carbon electrodes for stable and efficient capacitive deionization using inverted adsorption-desorption behavior. Energy Environ. Sci. 8, 897–909. doi: 10.1039/C4EE03172E
Gao, X., Porada, S., Omosebi, A., Liu, K. L., Biesheuvel, P. M., and Landon, J. (2016). Complementary surface charge for enhanced capacitive deionization. Water Res. 92, 275–282. doi: 10.1016/j.watres.2016.01.048
Ghaly, H. A., El-Deen, A. G., Souaya, E. R., and Allam, N. K. (2019). Asymmetric supercapacitors based on 3D graphene-wrapped V2O5 nanospheres and Fe3O4@3D graphene electrodes with high power and energy densities. Electrochim. Acta 310, 58–69. doi: 10.1016/j.electacta.2019.04.071
Guan, Y. F., Jiang, S., Cong, Y., Wang, J. P., Dong, Z. J., Zhang, Q., et al. (2020). A hydrofluoric acid-free synthesis of 2D vanadium carbide (V2C) MXene for supercapacitor electrodes. 2D Mater. 7:9. doi: 10.1088/2053-1583/ab6706
Guo, L., Mo, R. W., Shi, W. H., Huang, Y. X., Leong, Z. Y., Ding, M., et al. (2017). A prussian blue anode for high performance electrochemical deionization promoted by the faradaic mechanism. Nanoscale 9, 13305–13312. doi: 10.1039/C7NR03579A
Guo, Z. W., Ma, Y. Y., Dong, X. L., Hou, M. Y., Wang, Y. G., and Xia, Y. Y. (2018). Integrating desalination and energy storage using a saltwater-based hybrid sodium-ion supercapacitor. ChemSusChem 11, 1741–1745. doi: 10.1002/cssc.201800517
Han, J. L., Yan, T. T., Shen, J. J., Shi, L. Y., Zhang, J. P., and Zhang, D. S. (2019). Capacitive Deionization of saline water by using MoS2-graphene hybrid electrodes with high volumetric adsorption capacity. Environ. Sci. Technol. 53, 12668–12676. doi: 10.1021/acs.est.9b04274
Han, Y., Lu, Y. Z., Shen, S. H., Zhong, Y., Liu, S., Xia, X. H., et al. (2019). Enhancing the capacitive storage performance of carbon fiber textile by surface and structural modulation for advanced flexible asymmetric supercapacitors. Adv. Funct. Mater. 29:9. doi: 10.1002/adfm.201806329
Hand, S., and Cusick, R. D. (2017). Characterizing the impacts of deposition techniques on the performance of MnO2 cathodes for sodium electrosorption in hybrid capacitive deionization. Environ. Sci. Technol. 51, 12027–12034. doi: 10.1021/acs.est.7b03060
Huang, Y. X., Chen, F. M., Guo, L., and Yang, H. Y. (2017). Ultrahigh performance of a novel electrochemical deionization system based on a NaTi2(PO4)3/rGO nanocomposite. J. Mater. Chem. A 5, 18157–18165. doi: 10.1039/C7TA03725B
Huang, Y. X., Chen, F. M., Guo, L., Zhang, J., Chen, T. P., and Yang, H. Y. (2019). Low energy consumption dual-ion electrochemical deionization system using NaTi2(PO4)3-AgNPs electrodes. Desalination 451, 241–247. doi: 10.1016/j.desal.2018.02.006
Huang, Z., Lu, L., Cai, Z. X., and Ren, Z. J. (2016). Individual and competitive removal of heavy metals using capacitive deionization. J. Hazard. Mater. 302, 323–331. doi: 10.1016/j.jhazmat.2015.09.064
Kim, D. J., Ponraj, R., Kannan, A. G., Lee, H. W., Fathi, R., Ruffo, R., et al. (2013). Diffusion behavior of sodium ions in Na0.44MnO2 in aqueous and non-aqueous electrolytes. J. Power Sources 244, 758–763. doi: 10.1016/j.jpowsour.2013.02.090
Kim, H., Hong, J., Park, K. Y., Kim, H., Kim, S. W., and Kang, K. (2014). Aqueous rechargeable Li and Na ion batteries. Chem. Rev. 114, 11788–11827. doi: 10.1021/cr500232y
Kim, S., Lee, J., Kim, C., and Yoon, J. (2016). Na2FeP2O7 as a novel material for hybrid capacitive deionization. Electrochim. Acta 203, 265–271. doi: 10.1016/j.electacta.2016.04.056
Kim, T., Gorski, C. A., and Logan, B. E. (2017). Low energy desalination using battery electrode deionization. Environ. Sci. Technol. Lett. 4, 444–449. doi: 10.1021/acs.estlett.7b00392
Lang, J. W., Yan, X. B., Liu, W. W., Wang, R. T., and Xue, Q. J. (2012). Influence of nitric acid modification of ordered mesoporous carbon materials on their capacitive performances in different aqueous electrolytes. J. Power Sources 204, 220–229. doi: 10.1016/j.jpowsour.2011.12.044
Lee, J., Kim, S., Kim, C., and Yoon, J. (2014). Hybrid capacitive deionization to enhance the desalination performance of capacitive techniques. Energy Environ. Sci. 7, 3683–3689. doi: 10.1039/C4EE02378A
Lee, J., Kim, S., and Yoon, J. (2017a). Rocking chair desalination battery based on prussian blue electrodes. ACS Omega 2, 1653–1659. doi: 10.1021/acsomega.6b00526
Lee, J., Srimuk, P., Aristizabal, K., Kim, C., Choudhury, S., Nah, Y. C., et al. (2017b). Pseudocapacitive desalination of brackish water and seawater with vanadium-pentoxide-decorated multiwalled carbon nanotubes. ChemSusChem 10, 3611–3623. doi: 10.1002/cssc.201701215
Li, G. L., Yang, Z., Jiang, Y., Zhang, W. X., and Huang, Y. H. (2016). Hybrid aqueous battery based on Na3V2(PO4)3/C cathode and zinc anode for potential large-scale energy storage. J. Power Sources 308, 52–57. doi: 10.1016/j.jpowsour.2016.01.058
Li, H. B., Zou, L. D., Pan, L. K., and Sun, Z. (2010). Novel graphene-like electrodes for capacitive deionization. Environ. Sci. Technol. 44, 8692–8697. doi: 10.1021/es101888j
Li, J., Wang, X. X., Wang, H. Q., Wang, S. H., Hayat, T., Alsaedi, A., et al. (2017). Functionalization of biomass carbonaceous aerogels and their application as electrode materials for electro-enhanced recovery of metal ions. Environ. Sci. Nano 4, 1114–1123. doi: 10.1039/C7EN00019G
Li, Z. J., Yang, B. C., Zhang, S. R., and Zhao, C. M. (2012). Graphene oxide with improved electrical conductivity for supercapacitor electrodes. Appl. Surf. Sci. 258, 3726–3731. doi: 10.1016/j.apsusc.2011.12.015
Liu, W., Yu, L., Yin, R., Xu, X., Feng, J., Jiang, X., et al. (2020). Non-3d metal modulation of a 2D Ni-Co heterostructure array as multifunctional electrocatalyst for portable overall water splitting. Small 16:e1906775. doi: 10.1002/smll.201906775
Liu, W. X., Yin, R. L., Shi, W. H., Xu, X. L., Shen, X. H., Yin, Q. C., et al. (2019a). Gram-scale preparation of 2D transition metal hydroxide/oxide assembled structures for oxygen evolution and Zn-Air battery. ACS Appl. Energy Mater. 2, 579–586. doi: 10.1021/acsaem.8b01613
Liu, W. X., Yin, R. L., Xu, X. L., Zhang, L., Shi, W. H., and Cao, X. H. (2019b). Structural engineering of low-dimensional metal-organic frameworks: synthesis, properties, and applications. Adv. Sci. 6:32. doi: 10.1002/advs.201802373
Liu, Y., Xu, X. T., Wang, M., Lu, T., Sun, Z., and Pan, L. K. (2015). Metal-organic framework-derived porous carbon polyhedra for highly efficient capacitive deionization. Chem. Commun. 51, 12020–12023. doi: 10.1039/C5CC03999A
Lukatskaya, M. R., Mashtalir, O., Ren, C. E., Dall'Agnese, Y., Rozier, P., Taberna, P. L., et al. (2013). Cation intercalation and high volumetric capacitance of two-dimensional titanium carbide. Science 341, 1502–1505. doi: 10.1126/science.1241488
Naguib, M., Kurtoglu, M., Presser, V., Lu, J., Niu, J. J., Heon, M., et al. (2011). Two-dimensional nanocrystals produced by exfoliation of Ti3AlC2. Adv. Mater. 23, 4248–4253. doi: 10.1002/adma.201102306
Nakamoto, K., Kano, Y., Kitajou, A., and Okada, S. (2016). Electrolyte dependence of the performance of a Na2FeP2O7//NaTi2(PO4)3 rechargeable aqueous sodium-ion battery. J. Power Sources 327, 327–332. doi: 10.1016/j.jpowsour.2016.07.052
Nam, D. H., and Choi, K. S. (2017). Bismuth as a new chloride-storage electrode enabling the construction of a practical high capacity desalination battery. J. Am. Chem. Soc. 139, 11055–11063. doi: 10.1021/jacs.7b01119
Noonan, O., Liu, Y., Huang, X., and Yu, C. (2018). Layered graphene/mesoporous carbon heterostructures with improved mesopore accessibility for high performance capacitive deionization. J. Mater. Chem. A 6, 14272–14280. doi: 10.1039/C8TA03114B
Oladunni, J., Zain, J. H., Hai, A., Banat, F., Bharath, G., and Alhseinat, E. (2018). A comprehensive review on recently developed carbon based nanocomposites for capacitive deionization: from theory to practice. Sep. Purif. Technol. 207, 291–320. doi: 10.1016/j.seppur.2018.06.046
Panwar, N. L., Kaushik, S. C., and Kothari, S. (2011). Role of renewable energy sources in environmental protection: a review. Ren. Sust. Energy Rev. 15, 1513–1524. doi: 10.1016/j.rser.2010.11.037
Pasta, M., Wessells, C. D., Cui, Y., and La Mantia, F. (2012). A desalination battery. Nano Lett. 12, 839–843. doi: 10.1021/nl203889e
Peng, W. J., Wang, W., Han, G. H., Huang, Y. K., and Zhang, Y. S. (2020). Fabrication of 3D flower-like MoS2/graphene composite as high-performance electrode for capacitive deionization. Desalination 473:11. doi: 10.1016/j.desal.2019.114191
Porada, S., Borchardt, L., Oschatz, M., Bryjak, M., Atchison, J. S., Keesman, K. J., et al. (2013). Direct prediction of the desalination performance of porous carbon electrodes for capacitive deionization. Energy Environ. Sci. 6, 3700–3712. doi: 10.1039/c3ee42209g
Pujari, R. B., Lokhande, A. C., Shelke, A. R., Kim, J. H., and Lokhande, C. D. (2017). Chemically deposited nano grain composed MoS2 thin films for supercapacitor application. J. Colloid Interface Sci. 496, 1–7. doi: 10.1016/j.jcis.2016.11.026
Qian, J., Wu, C., Cao, Y., Ma, Z., Huang, Y., Ai, X., et al. (2018). Prussian blue cathode materials for sodium-ion batteries and other ion batteries. Adv. Energy Mater. 8:1702619. doi: 10.1002/aenm.201702619
Raza, W., Ali, F. Z., Raza, N., Luo, Y. W., Kim, K. H., Yang, J. H., et al. (2018). Recent advancements in supercapacitor technology. Nano Energy 52, 441–473. doi: 10.1016/j.nanoen.2018.08.013
Shahzad, M. W., Burhan, M., Ang, L., and Ng, K. C. (2017). Energy-water-environment nexus underpinning future desalination sustainability. Desalination 413, 52–64. doi: 10.1016/j.desal.2017.03.009
Shi, W. H., Li, H. B., Cao, X. H., Leong, Z. Y., Zhang, J., Chen, T. P., et al. (2016). Ultrahigh performance of novel capacitive deionization electrodes based on a three-dimensional graphene architecture with nanopores. Sci. Rep. 6:9. doi: 10.1038/srep18966
Shi, W. H., Liu, X. Y., Ye, C. Z., Cao, X. H., Gao, C. J., and Shen, J. N. (2019a). Efficient lithium extraction by membrane capacitive deionization incorporated with monovalent selective cation exchange membrane. Sep. Purif. Technol. 210, 885–890. doi: 10.1016/j.seppur.2018.09.006
Shi, W. H., Mao, J., Xu, X. L., Liu, W. X., Zhang, L., Cao, X. H., et al. (2019b). An ultra-dense NiS2/reduced graphene oxide composite cathode for high-volumetric/gravimetric energy density nickel-zinc batteries. J. Mater. Chem. A 7, 15654–15661. doi: 10.1039/C9TA04900B
Shi, W. H., Xu, X. L., Ye, C. Z., Sha, D. Y., Yin, R. L., Shen, X. H., et al. (2019c). Bimetallic metal-organic framework-derived carbon nanotube-based frameworks for enhanced capacitive deionization and Zn-Air battery. Front. Chem. 7:10. doi: 10.3389/fchem.2019.00449
Shi, W. H., Ye, C. Z., Xu, X. L., Liu, X. Y., Ding, M., Liu, W. X., et al. (2018). High-performance membrane capacitive deionization based on metal-organic framework-derived hierarchical carbon structures. ACS Omega 3, 8506–8513. doi: 10.1021/acsomega.8b01356
Song, W. X., Ji, X. B., Zhu, Y. R., Zhu, H. J., Li, F. Q., Chen, J., et al. (2014). Aqueous sodium-ion battery using a Na3V2(PO4)3 electrode. ChemElectroChem 1, 871–876. doi: 10.1002/celc.201300248
Srimuk, P., Kaasik, F., Kruner, B., Tolosa, A., Fleischmann, S., Jackel, N., et al. (2016). MXene as a novel intercalation-type pseudocapacitive cathode and anode for capacitive deionization. J. Mater. Chem. A 4, 18265–18271. doi: 10.1039/C6TA07833H
Srimuk, P., Lee, J., Fleischmann, S., Choudhury, S., Jackel, N., Zeiger, M., et al. (2017). Faradaic deionization of brackish and sea water via pseudocapacitive cation and anion intercalation into few-layered molybdenum disulfide. J. Mater. Chem. A 5, 15640–15649. doi: 10.1039/C7TA03120C
Sriramulu, D., and Yang, H. Y. (2019). Free-standing flexible film as a binder-free electrode for an efficient hybrid deionization system. Nanoscale 11, 5896–5908. doi: 10.1039/C8NR09119F
Sun, D., Ye, D. L., Liu, P., Tang, Y. G., Guo, J., Wang, L. Z., et al. (2018). MoS2/Graphene nanosheets from commercial bulky MoS2 andgraphite as anode materials for high rate sodium-ion batteries. Adv. Energy Mater. 8:11. doi: 10.1002/aenm.201702383
Suss, M., Porada, S., Sun, X., Biesheuvel, P., Yoon, J., and Presser, V. (2015). Water desalination via capacitive deionization: what is it and what can we expect from it? Energy Environ. Sci. 8, 2296–2319. doi: 10.1039/C5EE00519A
Tang, W. W., Liang, J., He, D., Gong, J. L., Tang, L., Liu, Z. F., et al. (2019). Various cell architectures of capacitive deionization: recent advances and future trends. Water Res. 150, 225–251. doi: 10.1016/j.watres.2018.11.064
Tian, S., Zhang, X., and Zhang, Z. (2020). Capacitive deionization with MoS2/g-C3N4 electrodes. Desalination 479:114348. doi: 10.1016/j.desal.2020.114348
Vafakhah, S., Guo, L., Sriramulu, D., Huang, S. Z., Saeedikhani, M., and Yang, H. Y. (2019). Efficient sodium-ion intercalation into the freestanding prussian blue/graphene aerogel anode in a hybrid capacitive deionization system. ACS Appl. Mater. Interfaces 11, 5989–5998. doi: 10.1021/acsami.8b18746
VahidMohammadi, A., Mojtabavi, M., Caffrey, N. M., Wanunu, M., and Beidaghi, M. (2019). Assembling 2D mxenes into highly stable pseudocapacitive electrodes with high power and energy densities. Adv. Mater. 31:9. doi: 10.1002/adma.201806931
Wang, J. G., Zhang, Z. Y., Zhang, X. Y., Yin, X. M., Li, X., Liu, X. R., et al. (2017). Cation exchange formation of prussian blue analogue submicroboxes for high-performance Na-ion hybrid supercapacitors. Nano Energy 39, 647–653. doi: 10.1016/j.nanoen.2017.07.055
Wang, L., and Lin, S. H. (2019). Mechanism of selective ion removal in membrane capacitive deionization for water softening. Environ. Sci. Technol. 53, 5797–5804. doi: 10.1021/acs.est.9b00655
Wang, L. B., Mu, C. N., Li, H. X., and Li, F. J. (2018). A dual-function battery for desalination and energy storage. Inorg. Chem. Front. 5, 2522–2526. doi: 10.1039/C8QI00704G
Wang, S. Q., Xia, L., Yu, L., Zhang, L., Wang, H. H., and Lou, X. W. (2016). Free-standing nitrogen-doped carbon nanofiber films: integrated electrodes for sodium-ion batteries with ultralong cycle life and superior rate capability. Adv. Energy Mater. 6:7. doi: 10.1002/aenm.201670044
Wang, Z., Yan, T. T., Fang, J. H., Shi, L. Y., and Zhang, D. S. (2016). Nitrogen-doped porous carbon derived from a bimetallic metal-organic framework as highly efficient electrodes for flow-through deionization capacitors. J. Mater. Chem. A 4, 10858–10868. doi: 10.1039/C6TA02420C
Wang, Z. J., Gong, H., Zhang, Y., Liang, P., and Wang, K. J. (2017). Nitrogen recovery from low-strength wastewater by combined membrane capacitive deionization (MCDI) and ion exchange (IE) process. Chem. Eng. J. 316, 1–6. doi: 10.1016/j.cej.2017.01.082
Wu, F., Gao, X., Xu, X., Jiang, Y., Gao, X., Yin, R., et al. (2020). MnO2 nanosheet-assembled hollow polyhedron grown on carbon cloth for flexible aqueous zinc-ion batteries. ChemSusChem 13, 1537–1545. doi: 10.1002/cssc.201903006
Xing, F., Li, T., Li, J. Y., Zhu, H. R., Wang, N., and Cao, X. (2017). Chemically exfoliated MoS2 for capacitive deionization of saline water. Nano Energy 31, 590–595. doi: 10.1016/j.nanoen.2016.12.012
Xu, X. L., Shi, W. H., Li, P., Ye, S. F., Ye, C. Z., Ye, H. J., et al. (2017). Facile fabrication of three-dimensional graphene and metal-organic framework composites and their derivatives for flexible all-solid-state supercapacitors. Chem. Mater. 29, 6058–6065. doi: 10.1021/acs.chemmater.7b01947
Xu, X. L., Shi, W. H., Liu, W. X., Ye, S. F., Yin, R. L., Zhang, L., et al. (2018). Preparation of two-dimensional assembled NiMn-C ternary composites for high-performance all-solid-state flexible supercapacitors. J. Mater. Chem. A 6, 24086–24091. doi: 10.1039/C8TA06412A
Xu, X. T., Li, J. L., Wang, M., Liu, Y., Lu, T., and Pan, L. K. (2016). Shuttle-like porous carbon rods from carbonized metal-organic frameworks for high-performance capacitive deionization. ChemElectroChem 3, 993–998. doi: 10.1002/celc.201600051
Xu, X. T., Pan, L. K., Liu, Y., Lu, T., Sun, Z., and Chua, D. H. C. (2015a). Facile synthesis of novel graphene sponge for high performance capacitive deionization. Sci. Rep. 5:9. doi: 10.1038/srep08458
Xu, X. T., Sun, Z., Chua, D. H. C., and Pan, L. K. (2015b). Novel nitrogen doped graphene sponge with ultrahigh capacitive deionization performance. Sci. Rep. 5:9. doi: 10.1038/srep11225
Yue, Z. S., Gao, T., and Li, H. B. (2019a). Robust synthesis of carbon@Na4Ti9O20 core-shell nanotubes for hybrid capacitive deionization with enhanced performance. Desalination 449, 69–77. doi: 10.1016/j.desal.2018.10.018
Yue, Z. S., Ma, Y. L., Zhang, J. W., and Li, H. (2019b). Pseudo-capacitive behavior induced dual-ion hybrid deionization system based on Ag@rGO parallel to Na1.1V3O7.9@rGO. J. Mater. Chem. A 7, 16892–16901. doi: 10.1039/C9TA03570B
Zhang, L., Liu, W. X., Shi, W. H., Xu, X. L., Mao, J., Li, P., et al. (2018). Boosting lithium storage properties of MOF derivatives through a wet-spinning assembled fiber strategy. Chem. Eur. J. 24, 13792–13799. doi: 10.1002/chem.201802826
Zhang, Q., Liao, C. Y., Zhai, T. Y., and Li, H. Q. (2016). A high rate 1.2V aqueous sodium-ion battery based on all NASICON structured NaTi2(PO4)3 and Na3V2(PO4)3. Electrochim. Acta 196, 470–478. doi: 10.1016/j.electacta.2016.03.007
Zhang, Q. C., Man, P., He, B., Li, C. W., Li, Q. L., Pan, Z. H., et al. (2020). Binder-free NaTi2(PO4)3 anodes for high-performance coaxial-fiber aqueous rechargeable sodium-ion batteries. Nano Energy 67:8. doi: 10.1016/j.nanoen.2019.104212
Zhang, X., Wang, X. G., Xie, Z. J., and Zhou, Z. (2016). Recent progress in rechargeable alkali metal-air batteries. Green Energy Environ. 1, 4–17. doi: 10.1016/j.gee.2016.04.004
Zhang, Y., Xia, X. H., Liu, B., Deng, S. J., Xie, D., Liu, Q., et al. (2019). Multiscale graphene-based materials for applications in sodium ion batteries. Adv. Energy Mater. 9:35. doi: 10.1002/aenm.201803342
Zhao, H. W., Chen, X. J., Wang, G. Z., Qiu, Y. F., and Guo, L. (2019). Two-dimensional amorphous nanomaterials: synthesis and applications. 2D Mater. 6:28. doi: 10.1088/2053-1583/ab1169
Zhao, W. Y., Ding, M., Guo, L., and Yang, H. Y. (2019). Dual-ion electrochemical deionization system with binder-free aerogel electrodes. Small 15:8. doi: 10.1002/smll.201805505
Zhao, W. Y., Guo, L., Ding, M., Huang, Y. X., and Yang, H. Y. (2018). Ultrahigh-desalination-capacity dual-ion electrochemical deionization device based on Na3V2(PO4)3@C-AgCl electrodes. ACS Appl. Mater. Interfaces 10, 40540–40548. doi: 10.1021/acsami.8b14014
Zhao, Y. B., Liang, B. L., Wei, X. J., Li, K. X., Lv, C. C., and Zhao, Y. (2019). A core-shell heterostructured CuFe@NiFe Prussian blue analogue as a novel electrode material for high-capacity and stable capacitive deionization. J. Mater. Chem. A 7, 10464–10474. doi: 10.1039/C8TA12433G
Zhou, F., Gao, T., Luo, M., and Li, H. B. (2018). Heterostructured graphene@Na4Ti9O20 nanotubes for asymmetrical capacitive deionization with ultrahigh desalination capacity. Chem. Eng. J. 343, 8–15. doi: 10.1016/j.cej.2018.02.124
Zhu, K., Zhang, H. Y., Ye, K., Zhao, W. B., Yan, J., Cheng, K., et al. (2017). Two-dimensional titanium carbide MXene as a capacitor-type electrode for rechargeable aqueous Li-ion and Na-ion capacitor batteries. ChemElectroChem 4, 3018–3025. doi: 10.1002/celc.201700523
Keywords: energy storage, sodium-ion battery, capacitive deionization, water desalination, faradaic electrode
Citation: Shi W, Gao X, Mao J, Qian X, Liu W, Wu F, Li H, Zeng Z, Shen J and Cao X (2020) Exploration of Energy Storage Materials for Water Desalination via Next-Generation Capacitive Deionization. Front. Chem. 8:415. doi: 10.3389/fchem.2020.00415
Received: 24 March 2020; Accepted: 21 April 2020;
Published: 15 May 2020.
Edited by:
Jiang Zhou, Central South University, ChinaReviewed by:
Huanwen Wang, China University of Geosciences Wuhan, ChinaCopyright © 2020 Shi, Gao, Mao, Qian, Liu, Wu, Li, Zeng, Shen and Cao. This is an open-access article distributed under the terms of the Creative Commons Attribution License (CC BY). The use, distribution or reproduction in other forums is permitted, provided the original author(s) and the copyright owner(s) are credited and that the original publication in this journal is cited, in accordance with accepted academic practice. No use, distribution or reproduction is permitted which does not comply with these terms.
*Correspondence: Haibo Li, bGloYWlib0BueHUuZWR1LmNu; Zhiyuan Zeng, emhpeXplbmdAY2l0eXUuZWR1Lmhr; Jiangnan Shen, c2hlbmpuQHpqdXQuZWR1LmNu; Xiehong Cao, Z2NzY2FveGhAemp1dC5lZHUuY24=
†These authors have contributed equally to this work
Disclaimer: All claims expressed in this article are solely those of the authors and do not necessarily represent those of their affiliated organizations, or those of the publisher, the editors and the reviewers. Any product that may be evaluated in this article or claim that may be made by its manufacturer is not guaranteed or endorsed by the publisher.
Research integrity at Frontiers
Learn more about the work of our research integrity team to safeguard the quality of each article we publish.