- 1School of Science, Southwest University of Science and Technology, Mianyang, China
- 2College of Science, Zhejiang University of Technology, Hangzhou, China
- 3Research Center for Photonic Technology, Fujian Key Laboratory for Advanced Micro-Nano Photonics Technology and Devices & Key Laboratory of Information Functional Material for Fujian Higher Education, Quanzhou Normal University, Fujian, China
- 4Basic Teaching Department, Neusoft Institute Guangdong, Foshan, China
Because of energy storage limitations and the high demand for energy, aqueous rechargeable lithium batteries (ARLBs) are receiving widespread attention due to their excellent performance and high safety. Lithium titanium phosphate (LiTi2(PO4)3) exhibits the potential to serve as anodes for ARLBs because it has a three-dimensional channel and a stable structure. We employed an anion (Cl−) doping strategy to boost the lithium storage performance of LiTi2(PO4)3. A series of LiTi2(PO4)3/C composites doped with Cl− on were successfully synthesized with a sol-gel technique as anodes for ARLBs. The effects of chlorine doping with different content on the properties of LiTi2(PO4)3−xCl3x/C (x = 0.05, 0.10, and 0.15) were investigated systematically. The doping of chlorine in appropriate amounts did not significantly impact the main structure and morphology of LiTi2(PO4)3/C. However, chlorine doping greatly increased the performance of LiTi2(PO4)3/C. LiTi2(PO4)2.9Cl0.3/C (LCl-10) showed the best electrochemical properties. It delivered a discharge capacity of 108.5 and 85.5 mAh g−1 at 0.5 and 15°C, respectively, with an increase of 13.2 and 43.3 mAh g−1 compared to blank LiTi2(PO4)3 (LCl). In addition, the discharge capacity of LCl-10 was maintained at 61.3% after 1,000 cycles at 5°C, implying an apparent improvement compared to LCl (35.3%). Our study showed that a chlorine-doped LiTi2(PO4)3/C composite is a potential anode for high-performance ARLBs.
Introduction
With the advancement of modern society, environmental pollution, and energy shortages have become more serious (He et al., 2019; Jiang et al., 2019; Zhou et al., 2019; Huan et al., 2020; Li et al., 2020a,b). Electrical energy can be derived from various other forms of energy, however, as an effective means to solve these problems. The secondary battery system is a necessary device for energy conversion and storage (Wu et al., 2017; Fang et al., 2019; Yang et al., 2019; Lu et al., 2020). The needs of high-performance electronic devices and electric vehicles have motivated the rapid development of lithium-ion batteries (LIBs) in recent years. The demand for LIBs offering high energy density, low pollution, and long life has rapidly increased (Wang et al., 2012; Zhao et al., 2016; Hua et al., 2018; Zhang et al., 2020). Conventional LIBs typically use organic electrolytes, which have demonstrated poor rate performance and potential safety hazards. ARLBs can efficiently solve these problems because they are environmentally friendly, highly secure, and inexpensive (Zhu et al., 2016; Lakhnot et al., 2019). Indeed, many cathodes of ARLBs exhibit remarkable electrochemical performance, such as LiMn2O4 (Manjunatha et al., 2011), LiFePO4 (He et al., 2011), and LiCoO2 (Ruffo et al., 2011).
Compared to cathodes, the restricted performance of anodes cannot meet the need for high-performance from ARLBs. Excellent anode materials are a limiting factor for the development of ARLB. Currently, vanadium oxide [VO2 (Li et al., 1994)] and vanadate [LiV3O8 (Zhao et al., 2011), NaV3O8 (Zhou et al., 2013)] have been used as anode materials. Nonetheless, these ARLB anodes have problems such as low capacity, poor cycle stability, and low rate performance. In recent times, the NASCION LiTi2(PO4)3 anode for ARLBs has shown great potential because it has a three-dimensional channel and a stable structure (Huang et al., 2015). A NASCION-type structure is marked by an open framework, which accelerates the diffusion of Li+ ions in crystal. TiO6 octahedra and PO4 tetrahedra comprise the LiTi2(PO4)3 framework unit. The TiO6 octahedron is connected to the PO4 tetrahedron, and the structure contains a large gap channel (Vidal-Abarca et al., 2012). Wessells et al. (2011) fabricated LiTi2(PO4)3 and demonstrated its potential as an ARLB anode. Luo and Xia (2009) reported a supercapacitor with LiTi2(PO4)3 coated with carbon as the negative electrode, delivering a discharge capacity of 30 mAh g−1.
However, the electrochemical performance of a LiTi2(PO4)3-based electrode must be increased to meet the requirements of practical applications. Carbon coating, size reduction, and the introduction of a conductive agent are efficient strategies for boosting performance (Sun et al., 2015). Moreover, lattice doping is available as a means of raising anode performance (Mao et al., 2019). Wang et al. (2017) partly replaced F− on a site for LiTi2(PO4)3 as an ARLB anode, with an energy density of 43.7 Wh Kg1. Liang et al. (2019) prepared Ga-doped LiTi2(PO4)3 film via a hydrothermal method and found favorable capacity and cycling performance. Doping with anions such as F− and Cl− can promote the electrokinetics of electrodes by influencing electron configuration, further increasing the electrical and ionic conductivity (Yue et al., 2013; Qi et al., 2015). In this paper, a Cl−1 doping strategy was employed to boost the lithium storage performance of LiTi2(PO4)3/C. LiTi2(PO4)3−xCl3x/C composites were successfully prepared with a facile sol-gel method. The lithium ion storage performance of anion-doped composites was systematically studied.
Experimental
Synthesis
All analytically pure chemical reagents were directly used without further treatment. The synthesis process of the materials is illustrated in Figure 1. Pristine LiTi2(PO4)3/C was synthesized via a sol-gel technique as follows. Briefly, 1.7921 g of Ti(OC4H9)4 was added to 20 mL of C2H5OH, and the Ti(OC4H9)4/C2H5OH solution was kept at room temperature with magnetic stirring. Then, 0.2659 g of CH3COOLi·2H2O and 0.1700 g of phenolic resin were dissolved in the mixture, sequentially. Following this, 0.8924 g of concentrated H3PO4 was added into 15 mL of C2H5OH and added dropwise to the above mixed solution. The mixed solution was reacted in a sealed beaker at 60°C for 2 h with magnetic stirring. When the reaction was complete, the solution was evaporated at 80°C to obtain a precursor. Finally, the precursor was calcined at 750°C for 5 h in an Ar atmosphere; the prepared LiTi2(PO4)3/C was named LCl. Moreover, LiTi2(PO4)3−xCl3x/C (x = 0.05, 0.10, and 0.15) composites were synthesized by partially replacing with Cl− in stoichiometry with LiCl. The corresponding LiTi2(PO4)3−xClx/C composites were denoted as LCl-05, LCl-10, and LCl-15.
Characterizations
X-ray powder diffraction (XRD, D/MAX2500PC, Rigaku) was carried out to analyze the crystalline phase of the composites. A scanning electron microscope (S-4800, Japan) was used to investigate the morphology of the samples and cycled electrodes. Cycled electrodes were obtained after disassembling the cells, washing them with deionized water, and drying them in an oven. A thermal analyzer (TG50, Shimadzu) was used for thermogravimetric analysis (TGA) of the samples.
Electrochemical Measurements
The electrodes were prepared as follows. The electrodes consisted of an intermixture of acetylene black, active substance, and PTFE (mass ratio: 1:1:8). The intermixture was pressed on a steel mesh with a radius of 7 mm. In cells, LiMn2O4, the synthesized samples, a saturated Li2SO4 solution, and glass fiber were used as the cathode, anode, electrolyte, and separator, respectively. A galvanostatic charge-discharge test for the cells was executed on a CT2001A testing system. Rate performance was evaluated at 0.2, 0.5, 1, 2, 5, 8, 10, 15, and 1°C, respectively. The cycling performance was recorded under 5°C for 1,000 cycles. Cyclic voltammetry and electrochemical impedance spectroscopy were performed to explore the lithium ion storage performance of Li+ ions using a CHI660E electrochemical workstation (Chenhua, Shanghai). Cyclic voltammetry tests were executed with a voltage range of 0–1.85 V. Electrochemical impedance spectroscopy tests for electrochemically activated cells were conducted with a frequency of 105-10−2 Hz and an amplitude of 5 mV.
Results and Discussion
The XRD patterns of the composites are presented in Figure 2. As shown, the main characteristic peaks of all composites were similar and exactly matched those of the NASICON LiTi2(PO4)3 with an R-3c space group (JCPDS#35-0754). This demonstrated that doping with small amount of Cl had no effect on the main structure of LiTi2(PO4)3. In addition, LCl-15 showed an impurity peak at 28.4 degrees, which demonstrated that a high Cl content slightly influenced the crystal structure of Cl-doped composites.
Representative SEM images of LCl and LCl-10 at various magnifications are displayed in Figure 3. As displayed, both materials were constituted by tiny primary particles at a nanometer scale. In addition, there was some agglomeration resulting from the high temperatures reached during the synthesis process. As for the morphology of the LCl-10 composite, it exhibited no obvious differences compared to pristine LCl, further implying that Cl doping had no obvious impact on the composite morphology.
The rate performance of the composites is shown in Figure 4A. Serious electrochemical polarization in a large current resulted in a decrease in the discharge capacity of all composites as the rate increased. The discharge capacity of Cl-doped LiTi2(PO4)3 composites, particularly LCl-10, was increased compared to the original LiTi2(PO3)4/C, indicating an improvement with Cl-doped anode materials. The difference in discharge capacity between Cl-doped LiTi2(PO4)3 composites and the original LiTi2(PO3)4/C became more obvious as the rate was increased. LCl-10 delivered a discharge capacity of 108.5 and 85.5 mAh g−1 at 0.5 and 15°C, respectively, an increase of 13.1 and 44.3 mAh g−1 compared to pristine LCl. Charge-discharge curves for the LCl and LCl-10 composites at disparate rates are displayed in Figures 4B,C, respectively. There were two voltage plateaus for LCl and LCl-10, at about 1.0 and 1.5 V. The voltage plateaus of LCl-10 were wider and more stable compared to those of LCl, revealing the outstanding electrochemical performance of LCl-10.
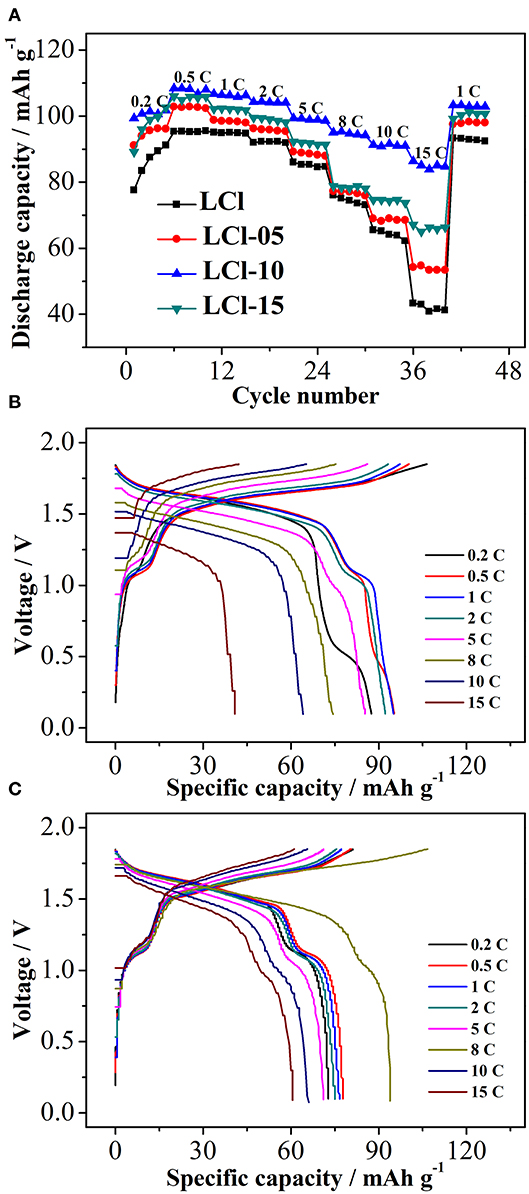
Figure 4. Rate performance of all composites (A), charge-discharge curves for LCl (B) and LCl-10 (C) at different rates.
The cycling properties of LCl and LCl-10 composites were studied at 5°C for 1,000 cycles, and the results are shown in Figure 5A. The discharge capacity of both composites gradually increased during the first several cycles due to electrode activation. The maximal discharge capacity of LCl-10 (95.27 mAh g−1) was higher than that of LCl (84.89 mAh g−1). The discharge capacity of LCl-10 was maintained at 61.3% after 1,000 cycles, which was 25.1% higher than that of LCl (36.2%). Figure 5B shows that the coulombic efficiencies of LCl and LCl-10 were in the vicinity of 100%, revealing that the cells had almost no self-discharge. The charge-discharge curves for LCl and LCl-10 after various cycles are shown in Figures 5C,D, respectively. The relatively wider and more stable voltage plateau for LCl-10 demonstrated good electrochemical performance and stability, unveiling the remarkable cycling properties of LCl-10.
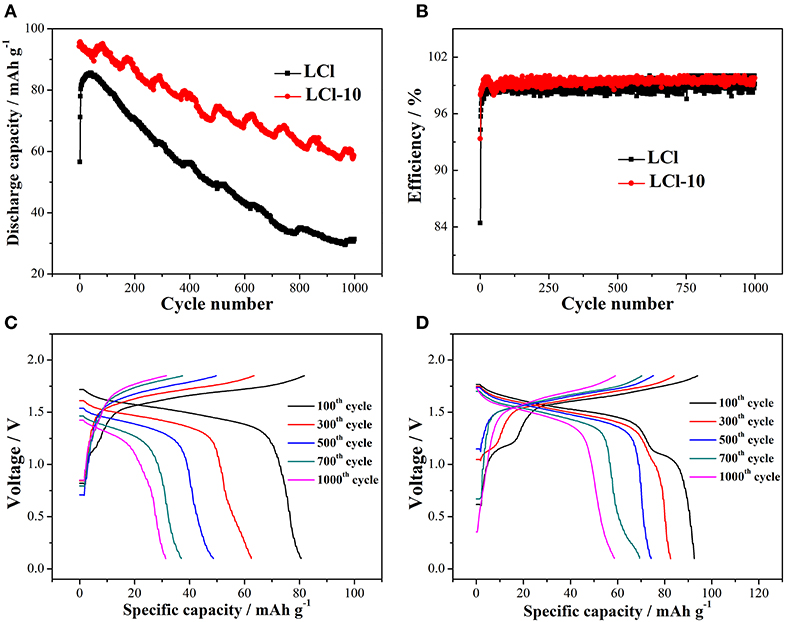
Figure 5. Cycling performances (A) and coulombic efficiency (B) for LCl and LCl-10 at 5°C for 1,000 cycles, charge-discharge curves for LCl (C) and LCl-10 (D) after different cycles.
In order to study the structural stability of the electrode after multiple cycles, the electrode morphology was analyzed by SEM. The results for LCl-10 electrodes after 100, 500, and 1,000 cycles are shown in Figure 6. As can be seen, the LCl-10 electrode surface was smooth after 100 cycles, and the morphology remained stable even after 1,000 cycles, without apparent dissolution. This demonstrated that LCl-10 had excellent structural stability after a long period of charging and discharging.
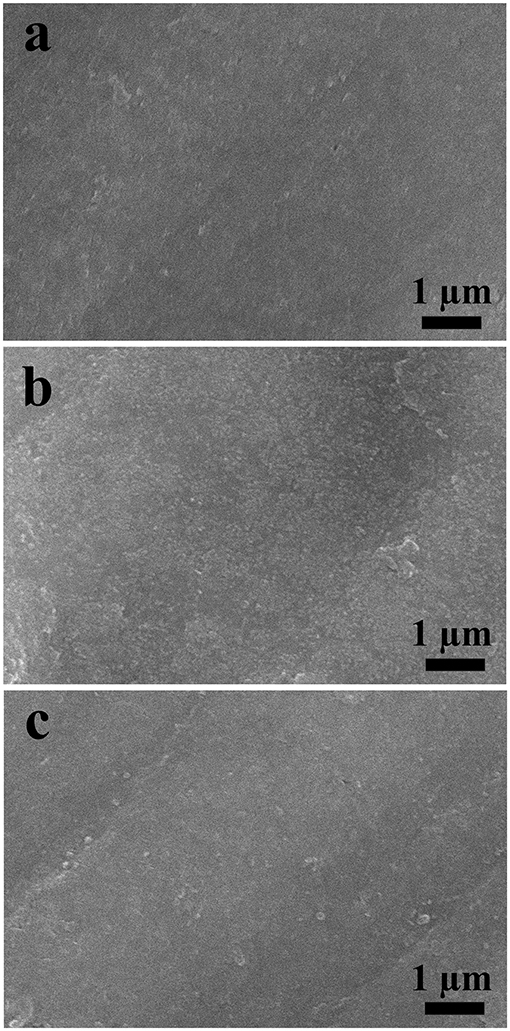
Figure 6. SEM images of LCl-10 anodes after 100 (a), 500 (b), and 1,000 (c) cycles at a rate of 5°C.
Cyclic voltammetry tests of the cells for LCl and LCl-10 were carried out to study the electrochemical kinetics, and the results are shown in Figure 7. As can be observed, both LCl and LCl-10 had two obvious current peaks. Further, the current density of LCl-10 was higher than the corresponding current density of LCl. The higher current density and narrower peak potential difference of LCl-10 illustrated the excellent electrochemical property of LCl-10. The anodic and cathodic peak current density of LCl-10 at 1.6 V reached 0.38 and 0.38 A g−1, respectively, with an obvious improvement compared to LCl (0.30 and 0.30 A g−1). The peak potential difference of LCl-10 with a value of 0.12 V was smaller than that of LCl (0.15 V), indicating better electrochemical reversibility of the modified electrode.
An electrochemical impedance spectroscopy test was implemented to investigate the effect of Cl doping on the electrochemical kinetics. Nyquist plots and fitting results for LCl and LCl-10 are shown in Figure 8. The Nyquist plots were characterized by an intercept, a semicircle, and an inclined line at high, middle, and low frequencies, parallel to ohmic resistance (Rs) including the electrolyte and electrode, charge transfer resistance (Rct) linked with the electrochemical reaction, and Warburg impedance (Zw) related to the migration of Li ions, respectively. As can be seen, the electronic conductivity of LCl-10 did not change significantly compared to that of LCl. This indicated that Cl doping caused no obvious change in the electronic conductivity of the material. This was due to the improvement in electronic conductivity from the introduction of carbon. LCl-10 delivered an Rct value of 30.0 Ω, which was less than that of LCl (43.9 Ω), illustrating that the Cl doping had a positive effect on the electrochemical kinetics.
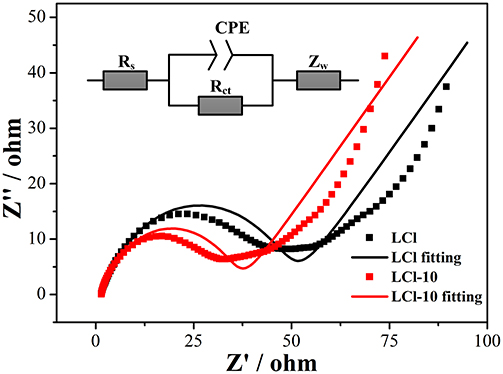
Figure 8. Electrochemical impedance spectra and fitting results of the assembled cell for LCl and LCl-10.
Conclusions
LiTi2(PO4)3/C composites doped with chlorine on phosphate spots were successfully synthesized with a sol-gel technique. Chlorine doping did not significantly impact the main structure and morphology of LiTi2(PO4)3/C. Of all the samples, LCL-10 composites showed the optimum rate performance, and their advantages were more obvious at a higher rate. LCl-10 had a discharge capacity of 108.5 and 85.5 mAh g−1 at 0.5 and 15°C, respectively, which was 13.1 and 44.3 mAh g−1 higher than those of LCl. Moreover, the discharge capacity of LCl-10 was maintained at 61.3% after 1,000 cycles at 5°C, which was 25.1% higher than that of LCl (36.2%). In a word, LiTi2(PO4)3/C doped with an appropriate amount of chlorine is a potential anode for high-performance ARLBs.
Data Availability Statement
All datasets generated for this study are included in the article/supplementary material.
Author Contributions
HL: carried out experiments and wrote the manuscript. YT: carried out experiments. ZX: performed analyzed experimental results. PW: designed experiments. ZL: revised the manuscript.
Funding
This research was funded by the National Natural Science Foundation of China (NNSFC), grant numbers 11704223 and 11803015, by the Natural Science Foundation of Fujian Province, grant number 2018J05008 and JZ160459, by the Ph.D. Research Startup Foundation of Quanzhou Normal University (G16057), and by the Distinguished Young Scholars Program of Fujian Province (C18032).
Conflict of Interest
The authors declare that the research was conducted in the absence of any commercial or financial relationships that could be construed as a potential conflict of interest.
References
Fang, Y. Z., Hu, R., Liu, B. Y., Zhang, Y. Y., Zhu, K., Yan, J., et al. (2019). MXene-derived TiO2/reduced graphene oxide composite with an enhanced capacitive capacity for Li-ion and K-ion batteries. J. Mater. Chem. A 7, 5363–5372. doi: 10.1039/C8TA12069B
He, D., Huang, X., and Li, M. (2019). Hierarchical CP(=O)(O)n (n ≤ 2)-linked nano-Si/N-doped C/graphene porous foam as anodes for high-performance lithium ion batteries. Carbon 141, 531–541. doi: 10.1016/j.carbon.2018.10.007
He, P., Liu, J. L., Cui, W. J., Luo, J. Y., and Xia, Y. Y. (2011). Investigation on capacity fading of LiFePO4 in aqueous electrolyte. Electrochim Acta 56, 2351–2357. doi: 10.1016/j.electacta.2010.11.027
Hua, K., Li, X., Fang, D., Bao, R., Yi, J., Luo, Z., et al. (2018). Vanadium trioxide nanowire arrays as a cathode material for lithium-ion battery. Ceram Int. 44, 11307–11313. doi: 10.1016/j.ceramint.2018.03.178
Huan, H., Jile, H., Tang, Y. J., Li, X., Yi, Z., Gao, X., et al. (2020). Fabrication of ZnO@Ag@Ag3PO4 ternary heterojunction: superhydrophilic properties, antireflection and photocatalytic properties. Micromachines 11:309. doi: 10.3390/mi11030309
Huang, Z., Liu, L., Zhou, Q., Tan, J., Yan, Z., Xia, D., et al. (2015). Carbon-coated lithium titanium phosphate nanoporous microplates with superior electrochemical performance. J. Power Sources 294, 650–657. doi: 10.1016/j.jpowsour.2015.06.143
Jiang, S. L., Huang, R. M., Zhu, W. C., Li, X. Y., Zhao, Y., Gao, Z. X., et al. (2019). Free-Standing SnO2@rGO anode via the anti-solvent-assisted precipitation for superior lithium storage performance. Front. Chem. 7:878. doi: 10.3389/fchem.2019.00878
Lakhnot, A. S., Gupta, T., Singh, Y., Hundekar, P., Jain, R., Han, F., et al. (2019). Aqueous lithium-ion batteries with niobium tungsten oxide anodes for superior volumetric and rate capability. Energy Storage Mater 27, 506–513. doi: 10.1016/j.ensm.2019.12.012
Li, J. K., Chen, X. F., Yi, Z., Yang, H., Tang, Y. J., Yi, Y., et al. (2020a). Broadband solar energy absorber based on monolayer molybdenum disulfide using tungsten elliptical arrays. Mater. Today Energy 16:100390. doi: 10.1016/j.mtener.2020.100390
Li, J. K., Chen, Z. Q., Yang, H., Yi, Z., Chen, X. F., Yao, W. T., et al. (2020b). Tunable broadband solar energy absorber based on monolayer transition metal dichalcogenides materials using au nanocubes. Nanomaterials 10:257. doi: 10.3390/nano10020257
Li, W., McKinnon, W. R., and Dahn, J. R. (1994). Lithium intercalation from aqueous solutions. J. Electrochem. Soc. 141, 2310–2316. doi: 10.1149/1.2055118
Liang, Y., Peng, C., Kamiike, Y., Kuroda, K., and Okido, M. (2019). Gallium doped NASICON type LiTi2(PO4)3 thin-film grown on graphite anode as solid electrolyte for all solid state lithium batteries. J. Alloy Compd. 775, 1147–1155. doi: 10.1016/j.jallcom.2018.10.226
Lu, M., Zhang, X., Ji, J., Xu, X., and Zhang, Y. (2020). Research progress on power battery cooling technology for electric vehicles. J. Energy Storage 27:101155. doi: 10.1016/j.est.2019.101155
Luo, J. Y., and Xia, Y.-Y. (2009). Electrochemical profile of an asymmetric supercapacitor using carbon-coated LiTi2(PO4)3 and active carbon electrodes. J. Power Sources 186, 224–227. doi: 10.1016/j.jpowsour.2008.09.063
Manjunatha, H., Mahesh, K. C., Suresh, G. S., and Venkatesha, T. V. (2011). The study of lithium ion de-insertion/insertion in LiMn2O4 and determination of kinetic parameters in aqueous Li2SO4 solution using electrochemical impedance spectroscopy. Electrochim Acta 56, 1439–1446. doi: 10.1016/j.electacta.2010.08.107
Mao, Q., Zhang, C., Yang, W., Yang, J., Sun, L., Hao, Y., et al. (2019). Mitigating the voltage fading and lattice cell variations of O3-NaNi0.2Fe0.35Mn0.45O2 for high performance Na-ion battery cathode by Zn doping. J. Alloy Compd. 794, 509–517. doi: 10.1016/j.jallcom.2019.04.271
Qi, Y., Mu, L., Zhao, J., Hu, Y.-,S., Liu, H., and Dai, S. (2015). Superior Na-storage performance of low-temperature-synthesized Na3(VO1−xPO4)2F1+2x (0 ≤ x ≤ 1) Nanoparticles for Na-ion batteries. Angew. Chem. Int. Ed. 54, 9911–9916. doi: 10.1002/anie.201503188
Ruffo, R., La Mantia, F., Wessells, C., Huggins, R. A., and Cui, Y. (2011). Electrochemical characterization of LiCoO2 as rechargeable electrode in aqueous LiNO3 electrolyte. Solid State Ionics 192, 289–292. doi: 10.1016/j.ssi.2010.05.043
Sun, D., Xue, X., Tang, Y., Jing, Y., Huang, B., Ren, Y., et al. (2015). High-rate LiTi2(PO4)3@N-C composite via bi-nitrogen sources doping. ACS Appl. Mater. Inter. 7, 28337–28345. doi: 10.1021/acsami.5b08697
Vidal-Abarca, C., Lavela, P., Aragon, M. J., Plylahan, N., and Tirado, J. L. (2012). The influence of iron substitution on the electrochemical properties of Li1+xTi2−xFex(PO4)3/C composites as electrodes for lithium batteries. J. Mater. Chem. 22, 21602–21607. doi: 10.1039/c2jm34227h
Wang, H. Q., Zhang, H. Z., Cheng, Y., Feng, K., Li, X. F., and Zhang, H. M. (2017). Rational design and synthesis of LiTi2(PO4)3−xFx anode materials for high-performance aqueous lithium ion batteries. J. Mater. Chem. A 5, 593–599. doi: 10.1039/C6TA08257B
Wang, Y., Yi, J., and Xia, Y. (2012). Recent progress in aqueous lithium-ion batteries. Adv. Energy Mater. 2, 830–840. doi: 10.1002/aenm.201200065
Wessells, C., Huggins, R. A., and Cui, Y. (2011). Recent results on aqueous electrolyte cells. J. Power Sources 196, 2884–2888. doi: 10.1016/j.jpowsour.2010.10.098
Wu, X., Xiang, Y., Peng, Q., Wu, X., Li, Y., Tang, F., et al. (2017). Green-low-cost rechargeable aqueous zinc-ion batteries using hollow porous spinel ZnMn2O4 as the cathode material. J. Mater. Chem. A 5, 17990–17997. doi: 10.1039/C7TA00100B
Yang, S. N., Zhang, M. S., Wu, X. W., Wu, X. S., Zeng, F. H., Li, Y. T., et al. (2019). The excellent electrochemical performances of ZnMn2O4/Mn2O3: The composite cathode material for potential aqueous zinc ion batteries. J. Electroanal Chem. 832, 69–74. doi: 10.1016/j.jelechem.2018.10.051
Yue, P., Wang, Z., Li, X., Xiong, X., Wang, J., Wu, X., et al. (2013). The enhanced electrochemical performance of LiNi0.6Co0.2Mn0.2O2 cathode materials by low temperature fluorine substitution. Electrochim Acta 95, 112–118. doi: 10.1016/j.electacta.2013.02.037
Zhang, C., Zheng, B., Song, Z., Shi, S., and Mao, H. (2020). Microwave-assisted synthesis of a novel CuC2O4·xH2O/Graphene composite as anode material for lithium ion batteries. Ceram Int. 46, 1018–1025. doi: 10.1016/j.ceramint.2019.09.066
Zhao, M., Zheng, Q., Wang, F., Dai, W., and Song, X. (2011). Electrochemical performance of high specific capacity of lithium-ion cell LiV3O8//LiMn2O4 with LiNO3 aqueous solution electrolyte. Electrochim Acta 56, 3781–3784. doi: 10.1016/j.electacta.2011.02.057
Zhao, R., Liu, J., and Gu, J. (2016). Simulation and experimental study on lithium ion battery short circuit. Appl. Energy 173, 29–39. doi: 10.1016/j.apenergy.2016.04.016
Zhou, D., Liu, S., Wang, H., and Yan, G. (2013). Na2V6O16•0.14H2O nanowires as a novel anode material for aqueous rechargeable lithium battery with good cycling performance. J. Power Sources 227, 111–117. doi: 10.1016/j.jpowsour.2012.11.022
Zhou, P., Zhang, M. Y., Wang, L. P., Huang, Q. Z., Su, Z. A., Li, L. W., et al. (2019). Synthesis and electrochemical performance of ZnSe electrospinning nanofibers as an anode material for lithium ion and sodium ion batteries. Front. Chem. 7:569. doi: 10.3389/fchem.2019.00569
Keywords: aqueous rechargeable lithium batteries, anode, anion doping, lithium titanium phosphate, electrochemical performance
Citation: Luo H, Tang Y, Xiang Z, Wu P and Li Z (2020) Cl− Doping Strategy to Boost the Lithium Storage Performance of Lithium Titanium Phosphate. Front. Chem. 8:349. doi: 10.3389/fchem.2020.00349
Received: 02 February 2020; Accepted: 03 April 2020;
Published: 12 May 2020.
Edited by:
Xianwen Wu, Jishou University, ChinaReviewed by:
Zhouguang Lu, Southern University of Science and Technology, ChinaKai Zhu, Harbin Engineering University, China
Copyright © 2020 Luo, Tang, Xiang, Wu and Li. This is an open-access article distributed under the terms of the Creative Commons Attribution License (CC BY). The use, distribution or reproduction in other forums is permitted, provided the original author(s) and the copyright owner(s) are credited and that the original publication in this journal is cited, in accordance with accepted academic practice. No use, distribution or reproduction is permitted which does not comply with these terms.
*Correspondence: Pinghui Wu, cGh3dSYjeDAwMDQwO3pqdS5lZHUuY24=
†These authors have contributed equally to this work