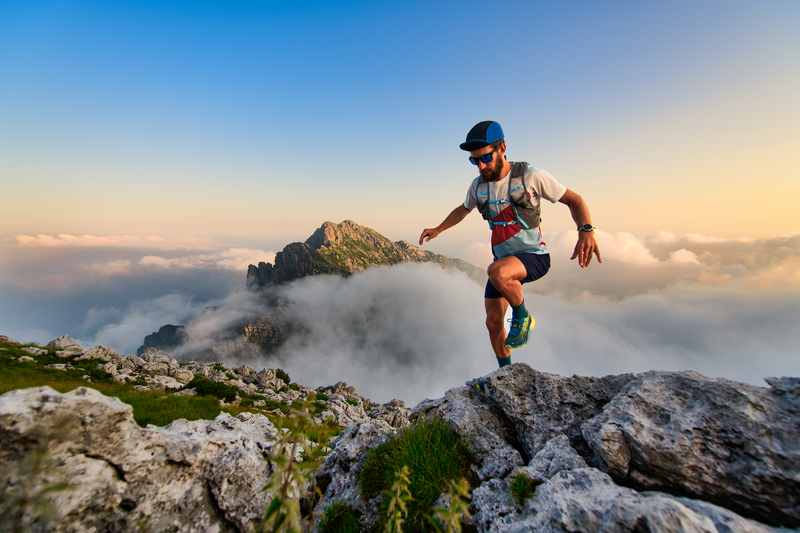
95% of researchers rate our articles as excellent or good
Learn more about the work of our research integrity team to safeguard the quality of each article we publish.
Find out more
ORIGINAL RESEARCH article
Front. Chem. , 22 April 2020
Sec. Chemical Biology
Volume 8 - 2020 | https://doi.org/10.3389/fchem.2020.00298
This article is part of the Research Topic Chemical Biology Editor’s Pick 2021 View all 9 articles
Cyclic depsipeptides constitute a fascinating class of natural products. Most of them are characterized by an ester formed between the β-hydroxy function of Ser/Thr -and related amino acids- and the carboxylic group of the C-terminal amino acid. Less frequent are those where the thiol of Cys is involved rendering a thioester (cyclo thiodepsipeptides) and even less common are the cyclo depsipeptides with a phenyl ester coming from the side-chain of Tyr. Herein, the preparation of the later through a cyclative cleavage using the Fmoc-MeDbz/MeNbz-resin is described. This resin has previously reported for the synthesis of cyclo thiodepsipeptides and homodetic peptides. The use of that resin for the preparation of all these peptides is also summarized.
Peptides are an important class of Active Pharmaceutical Ingredients (APIs) (Henninot et al., 2018; Lau and Dunn, 2018; Al Shaer et al., 2020; de la Torre and Albericio, 2020). They show excellent chemical and biological diversity, as well as high activity and specificity, low toxicity, low accumulation in tissues, and minimization of drug-drug interactions. Furthermore, the production of peptides ready for use in humans is relatively affordable thanks to the development of two complementary technologies, namely solid-phase peptide synthesis (SPPS) and reversed-phase high-performance liquid chromatography (RP-HPLC). The former was first described by Bruce Merrifield (Merrifield, 1963), and later refined by several groups, while the latter, founded on the pioneering work of Jim Waters (McDonald, 2006), allows the purification of complex synthetic crude peptides. Both technologies can be used to prepare peptides to fulfill research and industrial requirements (Zompra et al., 2009; Rasmussen, 2018).
Peptide synthesis can be briefly defined as the proper combination of protecting groups (Isidro-Llobet et al., 2009) and coupling reactions—the latter based exclusively on the activation of the carboxylic group (El-Faham and Albericio, 2011). Although there are a large number of coupling reagents that cleanly render the active species (El-Faham and Albericio, 2011), the development of the native chemical ligation (NCL) technique by Stephen Kent brought about a breakthrough in the field (Dawson et al., 1994). NCL involves the preparation of an unprotected C-thioester peptide that allows reaction with an unprotected N-terminal Cys to render first the thioester involving the thiol of the Cys, which rearranges to form the natural amide bond (Figure 1). This technique has allowed the synthesis of difficult, large peptides, and proteins (Camarero and Muir, 1999; Camarero et al., 2001; Miller et al., 2003; Avital-Shmilovici et al., 2013; Okamoto et al., 2014a,b; Boll et al., 2015; Pala-Pujadas et al., 2018; Snella et al., 2018; Spengler et al., 2018; Ashhurst et al., 2019; Sun and Brik, 2019; Jbara et al., 2020; Serra et al., 2020).
One of the keys to the success of the NCL approach is the preparation of unprotected C-thioester peptides. Although these compounds were first synthesized using the tert-butyloxycarbonyl (Boc)/benzyl (Bzl) strategy, several methods have been proposed for the same purpose using the friendlier fluorenylmethoxycarbonyl (Fmoc)/tert-butyl (tBu) approach. In this case, the main problem to overcome is the lack of stability of the thioester to the piperidine used in each step to remove the Fmoc group. In this regard, the thioester should be formed after elongation of the peptide. Although several linkers have been developed for this purpose (Camarero et al., 2004; Mulder et al., 2014; Kawakami et al., 2016; Morisaki et al., 2016; Olivier et al., 2017; Rao and Liu, 2017; Shelton et al., 2017; Flood et al., 2018), the 3-(Fmoc-amino)-4-(methylamino) benzoic acid (Fmoc-MeDbz, the acronym derives from N-methyl-diaminobenzoic acid) and/or its non-methylated analog developed by Blanco-Canosa and Dawson are optimal (Blanco-Canosa and Dawson, 2008; Blanco-Canosa et al., 2015) Fmoc-MeDbz is anchored to an amino resin. After removal of the Fmoc group, peptide elongation proceeds smoothly using normal coupling reagents (the electronic deactivation of the aromatic ring by the growing peptide chain and the presence of the Me in the second amino group impede the development of a second peptide chain on the N-Me-amino). Once the peptide has been elongated, the resin is reacted with p-nitrophenyl chloroformate, followed by cyclization in basic media [N,N-diisopropylethylamine (DIEA)] to render N-acyl-N'-methyl-benzimidazolinone (MeNbz)-resin. As an activated N-acylurea (N-acyl-N'-methylurea), this resin is susceptible to being a leaving group when under the attack of a nucleophile such as a thiol and therefore to rendering, in this case, the peptide thioester (Figure 2). This resin is considered a “safety catch,” in the terms described by Patek and Lebl, namely stable throughout peptide elongation and then activated to be labile to a given reagent (Patek and Lebl, 1999).
This MeNbz-resin has also been used by Stockdill's group to synthesize C-terminal-modified peptides (Arbour et al., 2017, 2018a,b). In this case, the linker is released through an intermolecular reaction by the action of nucleophiles such as alcohols, thiols, amines, and even amino acids.
Our group (Acosta et al., 2017) and Olsen's (Gless et al., 2017; Gless and Olsen, 2018) have used the same strategy but in an intramolecular mode for the preparation of cyclothiodepsipeptides. In this strategy, after the formation of the MeNbz-activated species, the protecting group of the side-chain of a Cys residue is removed and the free thiol attacks the peptidyl-MeNbz-resin to render the cyclic thioester, with concomitant release of the peptide from the resin (Figure 3). A further advantage of this “cyclative cleavage” approach is that only the cyclic peptide is released from the resin. In this regard, those lineal sequences that have not converted to the cyclic structure remain unaltered on the resin, thereby simplifying the crude product and thus the purification step.
Figure 3. Synthesis of head to side-chain cyclothiodepsipeptides (X = S) and cyclohomodeticpeptides (X = NH).
Our group has extended this “cyclative cleavage” to the preparation of homodetic cyclic peptides, where the nucleophile that causes the cleavage is an amino function, preferably from the side-chain of a peptide containing a basic amino acid (Abdel Monaim et al., 2018). In this case, the formation of the “head to side-chain” cyclic peptide is more favorable than the corresponding “head to tail” cyclic peptide, presumably due to the higher basicity of the ω-amino vs. the α-amino function (Figure 3).
Stockdill's group has recently reported an elegant strategy combining the two previous methods for the preparation of a “head to tail” cyclic peptide containing a Cys residue (Arbour et al., 2019). Thus, the formation of the cyclic thiodepsipeptide takes place by the attack of the thiol of a N-terminal Cys on the peptidyl-MeNbz-resin, after which the cyclic thiodepsipeptide rearranges to form the homodetic head to tail cyclic peptide.
An important class of cyclo heterodetic peptides are the head to side-chain cyclodepsipeptides, where a residue of Ser, Thr, or other β-hydroxy amino acid forms an ester bond with the carboxylic group of C-terminal amino acid (Pelay-Gimeno et al., 2013). In our hands, the application of cyclative cleavage on N-acyl-N'-MeDbz-resin for the preparation of Ser cyclodepsipeptides has proved unsuccessful, even when using strong bases such as NaH (results not shown).
Finally, less common but very intriguing peptides of the same family are those containing a phenyl ester coming from the phenol of a Tyr. These Tyr-cyclodepsipeptides are found in the fengycin family, which are lipodepsipeptides isolated from Bacillus strains (Sieber and Marahiel, 2003). Their syntheses are a challenge due to the inherent lability of the phenyl ester bond, which should be formed during the last steps of the synthetic strategy. Feliu and Planas' group developed an elegant strategy (Rosés et al., 2016; Roses et al., 2018), based on the following: incorporation of the first amino acid through the side-chain with the carboxylic acid protected in the form of the allyl ester; elongation of the peptide chain; formation of the phenyl ester with an N-Alloc-amino acid; treatment with Pd(0) to remove the allyl and the Alloc protecting groups; on-resin cyclization; and global deprotection and cleavage from the resin.
Here we report on a further application of the Fmoc-MeDbz/MeNbz-resin strategy for the synthesis of Tyr-cyclodepsipeptides.
As the aromatic ring of the Tyr side chain should confer more rigidity to the pending unit than the side chains of Cys or Lys/Orn/Dab/Dap studied in previous works (Acosta et al., 2017; Abdel Monaim et al., 2018), we first investigated the relevance of cyclodepsipeptide size with respect to facilitating the cyclization. Thus, model peptides with 6, 7, 8, and 9 amino acids were prepared, which correspond to cycles of 23, 26, 29, and 32-member rings, respectively (Figure 4). The sequence of these peptides was based on our previous works, related to the preparation of cyclo thiodepsipeptides and homodetic peptides as well (Acosta et al., 2017; Abdel Monaim et al., 2018). The peptide was built on a Fmoc-MeDbz-Gly-Rink-ChemMatrix resin with Fmoc/tBu amino acids using N,N'-diisopropylcarbodiimide (DIC) and OxymaPure in N,N-dimethylformamide (DMF) as coupling method (Subiros-Funosas et al., 2009). Tyr protected as Fmoc (α-amino) and 2-chlorotrityl (ClTrt) (phenol side-chain) was incorporated as the last amino acid. The Fmoc was removed with piperidine-DMF (2:8) and the amino function was acylated with hexanoic acid (Hx-OH). The Hx-Tyr(CTrt)-peptidyl-MeDbz-Gly-Rink-ChemMatrix resin was activated with p-nitrophenyl chloroformate in anhydrous DCM, followed by cyclization with 0.5 M DIEA in DMF for 45 min to render Hx-Tyr-peptidyl-MeNbz-resin with concomitant appearance of an intense yellow color due to the release of the p-nitrophenol. The peptide resin was then treated with a low concentration solution of trifluoroacetic acid (TFA) in DCM to remove the ClTrt protecting group of the phenol of Tyr. The peptide-resin was then washed thoroughly to ensure that no acid remained. The final cyclative cleavage occurred using 10% DIEA in DCM. The solutions, including the washings with DCM, were collected and concentrated. Cold diethyl ether (DEE) was added to facilitate the precipitation, and then centrifuged and decanted. The remaining solid was dissolved in CH3CN-H2O (1:1) and lyophilized.
As shown in Table 1, peptides 1 and 4, the smallest and the largest cycles, were not observed. In these cases, cyclization was attempted at 60°C with 10% DIEA in DMF, but no cyclic peptides were detected. However, peptides # 2 and # 3, containing 7 and 8 amino acids, respectively, showed a major peak in HPLC (Figures 5, 6) and the mass was corroborated by HPLC-MS (Supplementary Figures 3, 6).
Figure 5. HPLC analysis of crude peptide # 2 on a XBridge BEH130 C18 3.5 mm, 4.6 × 100 mm column. Eluents, A: H2O with 0.045% of TFA; B: ACN with 0.036% of TFA. Gradient: 5–100% B into A in 8 min, 1.0 mL/min, 220 nm 25°C.
Figure 6. HPLC analysis of crude peptide # 3 on XSelect C18 3.5 mm, 4.6 × 50 mm. Eluents, A: H2O with 0.1% of formic acid; B: ACN with 0.07% of formic acid. Gradient: 5–100% B into A in 8 min, 1.6 mL/min, 220 nm, 50°C.
It is difficult to generalize about peptide cyclization; however, peptides containing the same number of amino acids as peptide # 1, having Cys and Dab instead of Tyr, were able to cyclize (Acosta et al., 2017; Abdel Monaim et al., 2018). It is therefore possible to confirm that the phenol of the Tyr side chain imposes more restrictions than Cys or Dab residues. These limitations can be translated in either an inability to achieve the cyclative cleavage or/and the instability of the Tyr-cyclodepsipeptide once formed. As the linear peptides were not clearly detected, the most plausible explanation is the first one.
Next, the synthesis of the antifungal peptide BPC822 (Figure 7), first synthesized by Feliu and Planas' group, was attempted using the current methodology based on the use of the Fmoc-MeDbz/MeNbz-resin.
BPC822 contains eight amino acids similar to peptide # 3, and the phenyl ester is formed between the carboxylic group of the Ile and the phenol of the side-chain of Tyr. The involvement of the Ile, a β-substituted reside could add further difficulty to the formation of the ester. Thus, the Boc-Tyr(ClTrt)-Thr(tBu)-Glu(OtBu)-Val-Pro-Gln(Trt)-D-Tyr(tBu)-Ile-MeDbz-Gly-ChemMatrix-resin was constructed on a resin of reduced loading (0.33 mmol/g) to facilitate the cyclization. The resin was activated by treatment with p-nitrophenyl chloroformate in anhydrous DCM, followed by 0.5 M DIEA in DMF for 1 h with concomitant development of an intense yellow color due to the release of the p-nitrophenol to render the peptidyl-MeNbz-resin. The ClTrt, protecting group of the side-chain of the Tyr, was removed by short treatments with diluted solutions of TFA in DCM, as above for the model peptides.
The cyclative cleavage was first attempted with 10% DIEA at room temperature, but after the corresponding work-up (see below) no product was obtained. The same reaction was then repeated at 60°C for 30 min and under microwave-assisted heating (100 w) at 80°C for 15 min. In both cases, the DMF was evaporated and the remaining oil was treated with concentrated solutions of TFA-H2O-TIS (95:2.5:2.5) for 1 h to remove the remaining side-chain protecting groups. After partial evaporation of the TFA, the final product was then precipitated with cold DEE, centrifuged, decanted, and lyophilized. Although the two methods rendered the target peptide, the HPLC corresponding to the cyclative cleavage at 60°C (Figure 8) showed a much cleaner profile than that carried out under microwave conditions (Supplementary Figure 12). The final peptide was characterized by LC-MS and Maldi-TOF (Figure 9 and Supplementary Figures 9, 10, 11 and Table 1). In some cases, during the lyophilization and/or the posterior analysis, hydrolysis of the cyclic peptide was detected, thereby reinforcing the idea of the partial instability of head to side-chain Tyr-cyclodepsipeptides.
Figure 8. HPLC analysis of crude BPC822 peptide cyclized at 60°C on a XBridge BEH130 C18 3.5 mm, 4.6 × 100 mm column. Eluents, A: H2O with 0.045% of TFA; B: ACN with 0.036% of TFA. Gradient: 5–100% B into A in 8 min, 1.0 mL/min, 220 nm 25°C. The peak at 3.48 min could not be identified by MS.
Figure 9. Maldi-TOF of peak at 4.44 min of crude reaction peptide BPC822 obtained by heating at 60°C for 30 min. Calculated mass 993.48; mass found: [M + H]+ = 994.4986.
Here, the “safety catch” Fmoc-MeDbz/MeNbz-resin developed by Blanco-Canosa and Dawson for the preparation of linear peptide thioesters was investigated to synthesize “head to side-chain” cyclodepsipeptides through a cyclative cleavage. While this strategy proved unsuccessful for the preparation of those peptides involving Ser in the formation of the ester bond, even using strong bases, it allowed the preparation of Tyr-cyclodepsipeptides using DIEA as a base. In the case of unhindered C-terminal amino acids, the cyclative cleavage took place at room temperature. When the C-terminal amino acid was more hindered, the reaction occurred at 60°C. This technology represents a friendly method for the preparation of these fascinating peptides.
See Supplementary Information for a more detailed description of the full process. Herein, the key steps are described.
Although any amino resin can be used, aminomethyl ChemMatrix resin increases the success of the synthesis of these complex peptides (Garcia-Martin et al., 2006). To ensure quantitative incorporation of the Fmoc-MeDbz linker, a spacer of Gly is introduced to minimize steric hindrance caused by the polymeric matrix. The incorporation of the building blocks, linker and protected amino acids took place smoothly using 3 equiv. of each component, namely carboxylic acid, DIC, and OxymaPure in DMF. The incorporation first protected acid on the H-MeDbz-resin was carried out with HATU-DIEA due to the poor nucleophilicty of the benzyl amine present in the linker. Fmoc was removed with piperidine-DMF (2 × 5 min). Washings with DMF and DCM were intercalated during the treatments.
Acylation of the NMe aniline moiety was achieved by treatment with 4-nitrophenyl chloroformate (5 equiv.) in anhydrous DCM for 1 h. Two treatments were carried out to achieve quantitative yield. Although an acyl chloride was used, the reaction was done in the absence of base. After resin filtration and washing, 0.5 M of DIEA in DMF was added for 45 min to render the cyclic urea. The release of 4-nitrophenol is shown by a strong yellow color, which indicates the formation of the cyclic urea.
After the formation of the methylurea, the ClTrt of the phenol of Tyr was removed with TFA-triisopropylsilane (TIS)-DCM (2.5:2.5:95, 5 × 2 min washes). The resin was then washed thoroughly with DCM, DMF, and DCM, to ensure that no acid remained. The final cyclisation step occurred using 10% DIEA in DCM or DMF either at room temperature, at 60°C, or under microwave conditions, and the resin was further washed with DCM. The solution of 10% DIEA, including the DCM washings, was collected and evaporated to dryness. In the case of BCP822 peptide, the residue was treated for 1 h with TFA-TIS-DCM (95-2.5-2.5) to remove the remaining protecting groups and then evaporated to dryness.
In both cases, cold DEE was added in order to facilitate the precipitation of the peptide, which was then centrifuged and decanted. The remaining solid was dissolved in CH3CN-H2O (1:1) and lyophilized.
The datasets generated for this study are available on request to the corresponding author.
Experimental part was performed mainly by GA and LM under the supervision of the rest of co-authors. The manuscript was written with contributions from of all the authors. All authors have given approval to the final version of the manuscript.
This work was funded in part by the following: the National Research Foundation (NRF) and the University of KwaZulu-Natal (South Africa); MINECO, (RTI2018-093831-B-100), and the Generalitat de Catalunya (2017 SGR 1439) (Spain).
The authors declare that the research was conducted in the absence of any commercial or financial relationships that could be construed as a potential conflict of interest.
The authors thank University of KwaZulu Natal for encouraging this project.
The Supplementary Material for this article can be found online at: https://www.frontiersin.org/articles/10.3389/fchem.2020.00298/full#supplementary-material
Abdel Monaim, S. A. H., Acosta, G. A., Royo, M., El-Faham, A., de la Torre, B. G., and Albericio, F. (2018). Solid-phase synthesis of homodetic cyclic peptides from Fmoc-MeDbz-resin. Tetrahedron Lett. 59, 1779–1782. doi: 10.1016/j.tetlet.2018.03.084
Acosta, G. A., Royo, M., de la Torre, B. G., and Albericio, F. (2017). Facile solid-phase synthesis of head-side chain cyclothiodepsipeptides through a cyclative cleavage from MeDbz-resin. Tetrahedron Lett. 58, 2788–2791. doi: 10.1016/j.tetlet.2017.06.008
Al Shaer, D., Al Musaimi, O., Albericio, F., and de la Torre, B. G. (2020). 2019 FDA TIDES (Peptides and Oligonucleotides) harvest. Pharmaceuticals. 13:40. doi: 10.3390/ph13030040
Arbour, C. A., Belavek, K. J., Tariq, R., Mukherjee, S., Tom, J. K., Isidro-Llobet, A., et al. (2019). Bringing macrolactamization full circle: self-cleaving head-to-tail macrocyclization of unprotected peptides via mild N-acyl urea activation. J. Org. Chem. 84, 1035–1041. doi: 10.1021/acs.joc.8b02418
Arbour, C. A., Kondasinghe, T. D., Saraha, H. Y., Vorlicek, T. L., and Stockdill, J. L. (2018a). Epimerization-free access to C-terminal cysteine peptide acids, carboxamides, secondary amides, and esters via complimentary strategies. Chem. Sci. 9, 350–355. doi: 10.1039/C7SC03553E
Arbour, C. A., Saraha, H. Y., McMillan, T. F., and Stockdill, J. L. (2017). Exploiting the MeDbz linker to generate protected or unprotected C-terminally modified peptides. Chem. Eur. J. 23:, 12484–12488. doi: 10.1002/chem.201703380
Arbour, C. A., Stamatin, R. E., and Stockdill, J. L. (2018b). Sequence diversification by divergent C-Terminal elongation of peptides. J. Org. Chem. 83, 1797–1803. doi: 10.1021/acs.joc.7b02655
Ashhurst, A. S., McDonald, D. M., Hanna, C. C., Stanojevic, V. A., Britton, W. J., and Payne, R. J. (2019). Mucosal vaccination with a self-adjuvanted lipopeptide is immunogenic and protective against mycobacterium tuberculosis. J. Med. Chem. 62, 8080–8089. doi: 10.1021/acs.jmedchem.9b00832
Avital-Shmilovici, M., Mandal, K., Gates, Z. P., Phillips, N. B., Weiss, M. A., and Kent, S. B. H. (2013). Fully convergent chemical synthesis of ester insulin: determination of the high resolution X-ray structure by racemic protein crystallography. J. Am. Chem. Soc. 135, 3173–3185. doi: 10.1021/ja311408y
Blanco-Canosa, J. B., and Dawson, P. E. (2008). An efficient Fmoc-SPPS approach for the generation of thioester peptide precursors for use in native chemical ligation. Angew. Chem. Int. Ed. 47, 6851–6855. doi: 10.1002/anie.200705471
Blanco-Canosa, J. B., Nardone, B., Albericio, F., and Dawson, P. E. (2015). Chemical protein synthesis using a second-generation N-Acylurea linker for the preparation of peptide-thioester precursors. J. Am. Chem. Soc. 137, 7197–7209. doi: 10.1021/jacs.5b03504
Boll, E., Ebran, J.-P., Drobecq, H., El-Mahdi, O., Raibaut, L., Ollivier, N., et al. (2015). Access to large cyclic peptides by a one-pot two-peptide segment ligation/cyclization process. Org. Lett. 17, 130–133. doi: 10.1021/ol503359w
Camarero, J. A., Fushman, D., Cowburn, D., and Muir, T. W. (2001). Peptide chemical ligation inside living cells: in vivo generation of a circular protein domain. Bioorg. Med. Chem. 9, 2479–2484. doi: 10.1016/S0968-0896(01)00217-6
Camarero, J. A., Hackel, B. J., De Yoreo, J. J., and Mitchell, A. R. (2004). Fmoc-based synthesis of peptide α-thioesters using an aryl hydrazine support. J. Org. Chem. 69, 4145–4151. doi: 10.1021/jo040140h
Camarero, J. A., and Muir, T. W. (1999). Biosynthesis of a head-to-tail cyclized protein with improved biological activity. J. Am. Chem. Soc. 121, 5597–5598. doi: 10.1021/ja990929n
Dawson, P. E., Muir, T. W., Clark-Lewis, I., and Kent, S. B. (1994). Synthesis of proteins by native chemical ligation. Science 266:776. doi: 10.1126/science.7973629
de la Torre, B. G., and Albericio, F. (2020). The pharmaceutical industry in 2019. an analysis of FDA drug approvals from the perspective of molecules. Molecules 25:745. doi: 10.3390/molecules25030745
El-Faham, A., and Albericio, F. (2011). Peptide coupling reagents, more than a letter soup. Chem. Rev. 111, 6557–6602. doi: 10.1021/cr100048w
Flood, D. T., Hintzen, J. C. J., Bird, M. J., Cistrone, P. A., Chen, J. S., and Dawson, P. E. (2018). Leveraging the Knorr pyrazole synthesis for the facile generation of thioester surrogates for use in native chemical ligation. Angew. Chem. Int. Ed. 57, 11634–11639. doi: 10.1002/anie.201805191
Garcia-Martin, F., Quintanar-Audelo, M., Garcia-Ramos, Y., Cruz, L. J., Gravel, C., Furic, R., et al. (2006). ChemMatrix, a Poly(ethylene glycol)-based support for the solid-phase synthesis of complex peptides. J. Comb. Chem. 8, 213–220. doi: 10.1021/cc0600019
Gless, B. H., and Olsen, C. A. (2018). Direct peptide cyclization and one-pot modification using the MeDbz linker. J. Org. Chem. 83, 10525–10534. doi: 10.1021/acs.joc.8b01237
Gless, B. H., Peng, P., Pedersen, K. D., Gotfredsen, C. H., Ingmer, H., and Olsen, C. A. (2017). Structure–activity relationship study based on Autoinducing Peptide (AIP) from dog pathogen, S. schleiferi. Org. Lett. 19, 5276–5279. doi: 10.1021/acs.orglett.7b02550
Henninot, A., Collins, J. C., and Nuss, J. M. (2018). The current state of peptide drug discovery: back to the future? J. Med. Chem. 61, 1382–1414. doi: 10.1021/acs.jmedchem.7b00318
Isidro-Llobet, A., Alvarez, M., and Albericio, F. (2009). Amino acid-protecting groups. Chem. Rev. 109, 2455–2504. doi: 10.1021/cr800323s
Jbara, M., Maity, S. K., and Brik, A. (2020). Examining several strategies for the chemical synthesis of phosphorylated histone H3 reveals the effectiveness of the convergent approach. Eur. J. Org. Chem. doi: 10.1002/ejoc.201900257. [Epub ahead of print].
Kawakami, T., Mishima, Y., Kinoshita, M., Lee, Y.-H., and Suetake, I. (2016). A thiirane linker for isopeptide mimetics by peptide ligation. Tetrahedron Lett. 57, 2112–2115. doi: 10.1016/j.tetlet.2016.04.006
Lau, J. L., and Dunn, M. K. (2018). Therapeutic peptides: historical perspectives, current development trends, and future directions. Bioorg. Med. Chem. 26, 2700–2707. doi: 10.1016/j.bmc.2017.06.052
McDonald, P. D. (2006). “James waters and his liquid chromatography people: a personal perspective,” in The 350 Chromatographic Society Golden Jubilee Book: Recognising Half a Century of Supporting 351 Progress in Separation Science (ChromSoc, ILM).
Merrifield, R. B. (1963). Solid phase peptide synthesis. I the synthesis of a Tetrapeptide'. J. Am. Chem. Soc. 85, 2149–2154. doi: 10.1021/ja00897a025
Miller, J. S., Dudkin, V. Y., Lyon, G. J., Muir, T. W., and Danishefsky, S. J. (2003). Toward fully synthetic N-linked glycoproteins. Angew. Chem. Int. Ed. 42, 431–434. doi: 10.1002/anie.200390131
Morisaki, T., Denda, M., Yamamoto, J., Tsuji, D., Inokuma, T., Itoh, K., et al. (2016). An N-sulfanylethylanilide-based traceable linker for enrichment and selective labelling of target proteins. Chem. Commun. 52, 6911–6913. doi: 10.1039/C6CC01229A
Mulder, M. P. C., El Oualid, F., ter Beek, J., and Ovaa, H. (2014). A native chemical ligation handle that enables the synthesis of advanced activity-based probes: diubiquitin as a case study. ChemBioChem. 15, 946–949. doi: 10.1002/cbic.201402012
Okamoto, R., Mandal, K., Ling, M., Luster, A. D., Kajihara, Y., and Kent, S. B. H. (2014a). Total chemical synthesis and biological activities of glycosylated and non-glycosylated forms of the chemokines CCL1 and Ser-CCL1. Angew. Chem. Int. Ed. 53, 5188–5193. doi: 10.1002/anie.201310574
Okamoto, R., Mandal, K., Sawaya, M. R., Kajihara, Y., Yeates, T. O., and Kent, S. B. H. (2014b). (Quasi-)Racemic X-ray structures of glycosylated and non-glycosylated forms of the chemokine Ser-CCL1 prepared by total chemical synthesis. Angew. Chem. Int. Ed. 53, 5194–5198. doi: 10.1002/anie.201400679
Olivier, N., Desmet, R., Drobecq, H., Blanpain, A., Boll, E., Leclercq, B., et al. (2017). A simple and traceless solid phase method simplifies the assembly of large peptides and the access to challenging proteins. Chem. Sci. 8, 5362–5370. doi: 10.1039/C7SC01912B
Pala-Pujadas, J., Albericio, F., and Blanco-Canosa, J. B. (2018). Peptide ligations by using aryloxycarbonyl-o-methylaminoanilides: chemical synthesis of palmitoylated sonic hedgehog. Angew. Chem. Int. Ed. 57, 16120–16125. doi: 10.1002/anie.201810712
Patek, M., and Lebl, M. (1999). Safety-catch and multiply cleavable linkers in solid-phase synthesis. Biopolymers 47, 353–363. doi: 10.1002/(SICI)1097-0282(1998)47:5<353::AID-BIP3>3.0.CO;2-I
Pelay-Gimeno, M., Tulla-Puche, J., and Albericio, F. (2013). “Head-to-side-chain" cyclodepsipeptides of marine origin. Mar. Drugs 11, 1693–1717. doi: 10.3390/md11051693
Rao, C., and Liu, C.-F. (2017). Peptide Weinreb amide derivatives as thioester precursors for native chemical ligation. Org. Biomol. Chem. 15, 2491–2496. doi: 10.1039/C7OB00103G
Rasmussen, J. H. (2018). Synthetic peptide API manufacturing: a mini review of current perspectives for peptide manufacturing. Bioorg. Med. Chem. 26, 2914–2918. doi: 10.1016/j.bmc.2018.01.018
Roses, C., Camo, C., Oliveras, A., Moll, L., Lopez, N., Feliu, L., et al. (2018). Total solid-phase synthesis of dehydroxy fengycin derivatives. J. Org. Chem. 83, 15297–15311. doi: 10.1021/acs.joc.8b02553
Rosés, C., Camó, C., Vogels, K., Planas, M., and Feliu, L. (2016). Solid-phase synthesis of cyclic depsipeptides containing a tyrosine phenyl ester bond. Org. Lett. 18, 4140–4143. doi: 10.1021/acs.orglett.6b02281
Serra, G., Posada, L., and Hojo, H. (2020). On-resin synthesis of cyclic peptides via tandem N-to-S acyl migration and intramolecular thiol additive-free native chemical ligation. Chem. Commun. 56, 956–959. doi: 10.1039/C9CC07783A
Shelton, P. M. M., Weller, C. E., and Chatterjee, C. (2017). A facile N-mercaptoethoxyglycinamide (MEGA) linker approach to peptide thioesterification and cyclization. J. Am. Chem. Soc. 139, 3946–3949. doi: 10.1021/jacs.6b13271
Sieber, S. A., and Marahiel, M. A. (2003). Learning from Nature's drug factories: nonribosomal synthesis of macrocyclic peptides. J. Bacteriol. 185:7036. doi: 10.1128/JB.185.24.7036-7043.2003
Snella, B., Diemer, V., Drobecq, H., Agouridas, V., and Melnyk, O. (2018). Native chemical ligation at serine revisited. Org. Lett. 20, 7616–7619. doi: 10.1021/acs.orglett.8b03355
Spengler, J., Blanco-Canosa, J. B., Forni, L., and Albericio, F. (2018). One-pot peptide ligation-oxidative cyclization protocol for the preparation of short-/medium-size disulfide cyclopeptides. Org. Lett. 20, 4306–4309. doi: 10.1021/acs.orglett.8b01741
Subiros-Funosas, R., Prohens, R., Barbas, R., El-Faham, A., and Albericio, F. (2009). Oxyma: an efficient additive for peptide synthesis to replace the benzotriazole-based HOBt and HOAt with a lower risk of explosion. Chem. Eur. J. 15, 9394–9403. doi: 10.1002/chem.200900614
Sun, H., and Brik, A. (2019). The journey for the total chemical synthesis of a 53 kDa protein. Acc. Chem. Res. 52:3361–3371. doi: 10.1021/acs.accounts.9b00372
Keywords: cyclothiodepsipeptides, heterodetic cyclic peptides, homodetic cyclic peptides, native chemical ligation, solid-phase peptide synthesis
Citation: Acosta GA, Murray L, Royo M, de la Torre BG and Albericio F (2020) Solid-Phase Synthesis of Head to Side-Chain Tyr-Cyclodepsipeptides Through a Cyclative Cleavage From Fmoc-MeDbz/MeNbz-resins. Front. Chem. 8:298. doi: 10.3389/fchem.2020.00298
Received: 11 February 2020; Accepted: 25 March 2020;
Published: 22 April 2020.
Edited by:
Laszlo Otvos, Olpe LLC, United StatesReviewed by:
Mare Cudic, Florida Atlantic University, United StatesCopyright © 2020 Acosta, Murray, Royo, de la Torre and Albericio. This is an open-access article distributed under the terms of the Creative Commons Attribution License (CC BY). The use, distribution or reproduction in other forums is permitted, provided the original author(s) and the copyright owner(s) are credited and that the original publication in this journal is cited, in accordance with accepted academic practice. No use, distribution or reproduction is permitted which does not comply with these terms.
*Correspondence: Fernando Albericio, YWxiZXJpY2lvQHVrem4uYWMuemE=
Disclaimer: All claims expressed in this article are solely those of the authors and do not necessarily represent those of their affiliated organizations, or those of the publisher, the editors and the reviewers. Any product that may be evaluated in this article or claim that may be made by its manufacturer is not guaranteed or endorsed by the publisher.
Research integrity at Frontiers
Learn more about the work of our research integrity team to safeguard the quality of each article we publish.