- 1Department of Chemistry, Zhejiang University, Hangzhou, China
- 2College of Materials & Environmental Engineering, Hangzhou Dianzi University, Hangzhou, China
Selective oxidation of methane to methanol has been often considered as a “holy grail” reaction in catalysis. Herein, we systematically investigate the effect of solution pH and Pd-to-Au ratio of AuPdx colloid on the catalytic performance of methane oxidation. It is revealed that these two parameters can determine the amount of H2O2 participated in the reaction, which is linearly related to the productivity of oxygenates. A high catalytic performance in methane activation requires a high utilization of H2O2 to generate more ·OH. The optimal pH is 3.0 and the optimal Pd-to-Au ratio is between 0.1 to 0.7.
Introduction
Natural gas, the cleanest and cheapest fossil fuel, is widely accepted as a promising alternative resource to crude oil. According to BP statistical review of world energy, the proven natural gas reserve is more than 190 trillion cubic meters in 2018. Its global consumption and production have increased by over 5%, which recorded the largest annual growth for over 30 years (Dudley, 2019). Unfortunately, natural gas reserve is generally located in remote areas far away from its markets, which leads to high transportation cost (Jones et al., 1987). In order to economically utilize the resource, it is necessary to convert methane, the major constituent of natural gas, into liquid fuels for easier transportation. Currently, the industrial transformation of methane proceeds via an indirect route in which methane is first converted to syngas and then the syngas is converted to methanol and higher hydrocarbons (Vernon et al., 1990; Cheng et al., 2016, 2017; Jiao et al., 2016; Alvarez-Galvan et al., 2019). The high energy consumption and capital input, however, limit the wide application of this process, especially in the utilization of remote located natural gas fields. To address this problem, increasing effort has been devoted to the direct conversion of methane, a more economical and environmentally friendly route to utilize methane (Tang et al., 2014; Schwach et al., 2017). Among them, the direct oxidation of methane to methanol has been one of the major challenges for many decades (Palkovits et al., 2009; Grundner et al., 2015; Shan et al., 2017; Sushkevich et al., 2017; Cui et al., 2018; Park et al., 2019; Jin et al., 2020).
In practice, the oxidation of methane to methanol can be performed in the liquid or gas phase (Palkovits et al., 2009; Sushkevich et al., 2017; Cui et al., 2018; Park et al., 2019). However, the gas-phase and high temperature processes generally have limited methanol yield due to the thermodynamically favored over-oxidation of methanol to COx. During the last few years, increasing attention has been devoted to the low-temperature liquid-phase oxidation of methane to methanol using N2O, O2, and H2O2 as oxidants (Agarwal et al., 2017; Williams et al., 2018; McVicker et al., 2020). Recently, Hutchings et al. reported a low-temperature (50°C) route in aqueous hydrogen peroxide (H2O2) for oxidizing methane to methanol in high yield (92%). By using unsupported colloidal Au-Pd nanoparticles as a catalyst, they successfully incorporated molecular oxygen into the liquid oxidation products (Agarwal et al., 2017). It is important to highlight that the activation of methane to methyl radical by H2O2 is a key step for the incorporation of molecular oxygen. However, no quantitative relationship between the utilization of H2O2 and methane activation activity is obtained yet. Besides, due to the self-decomposition of H2O2 in the reaction conditions, only part of H2O2 can participate in the activation of methane. Considering the high price of hydrogen peroxide (0.67 $ kg−1 of 100% H2O2) (Thomas et al., 2000), it is also economically important to maximize the utilization efficiency of H2O2. To address these problems, we tightly control the solution pH and Pd-to-Au ratio of AuPd colloid nanoparticles to manage the utilization efficiency of H2O2. It turns out that the productivity of oxygenates is linearly related to the amount of H2O2 that participated in the activation of methane.
Experimental
Chemicals and Materials
Sodium borohydride (NaBH4, 96%), palladium chloride (PdCl2, 98.5%), hydrogen tetrachloroaurate trihydrate (HAuCl4·3H2O, 99.9%) were obtained from Sinopharm Chemical Reagent Co., Ltd., China. Polyvinyl pyrrolidone (PVP, 360,000 Da) was from Shanghai Macklin Biochemical Co., Ltd., China. Methane (CH4, 99.99%) and oxygen (O2, 99.999%) was from Hangzhou Jingong Gas, China. Deionized water was used throughout the experiments.
Catalyst Preparation
The colloidal AuPdx nanoparticles (x is the Pd-to-Au molar ratio) were synthesized by a deposition (Lopez-Sanchez et al., 2008; Agarwal et al., 2017) method. Firstly, Au and Pd precursor were prepared in deionized water with polyvinyl pyrrolidone (PVP, 360,000 Da) added as a stabilizer. After 10 min of stirring, the precursors were reduced with freshly prepared 0.1 M NaBH4 solution. The red or brown colloid was left stirring for at least 30 min to ensure all the metal precursor salts reduced to metallic nanoparticles and NaBH4 decomposed.
Characterizations
Transmission electron microscopy (TEM) images were taken using a Hitachi HT7700 microscope operated at 120 kV by drop casting the nanocrystal dispersions onto carbon-coated Cu grids and drying under ambient conditions. Powder X-ray diffraction (XRD) patterns were recorded on a Rigaku Ultima IV diffractometer with Cu Kα radiation. UV-vis absorption spectra were taken using a UNIC UV-2802 spectrophotometer.
Catalytic Measurement
The oxidation of methane was conducted using a stirred autoclave reactor (30 mL, DHA-M630). Typically, the vessel was charged with 10 mL of catalyst (Au-Pd colloid, 6.6 μmol) and fresh added H2O2. The pH of the solution was adjusted by HCl and NaOH. Subsequently, the autoclave was sealed and purged 3 times with methane before being pressurized with methane (3.0 MPa) and oxygen (0.5 MPa). The autoclave was heated to 50°C within 20 min and stirred at 700 rpm for another 30 min. Once the reaction was completed, the autoclave was put into an ice bath. The concentration of H2O2 was quantified by UV-vis spectroscopy with acidified K2TiO(C2O4)2 solution as chromogenic agent (Meng et al., 2018; Wang et al., 2019). The gas phase product of the reactions was quantified by GC with TCD detectors. 1H-NMR studies were carried out to quantify the amounts of liquid phase products using a Bruker 600 MHz NMR equipped with a solvent suppression system (Shan et al., 2017). An internal standard containing 1% TMS in CDCl3 (99.9% D) was placed in a sealed tube and used to quantify the amount of product. It is important to highlight that the presence of PVP is very important to the stability of AuPd colloid catalysts. TEM images of spent catalysts under different pH suggest that the particle sizes of the spent catalysts are close to each other and are only slightly larger than those of fresh catalysts, indicating the colloidal catalysts are stable in the pH range from 1 to 8. In contrast, colloidal catalysts without PVP coagulate after 3 h reaction at pH 6–8 or 0.5 h reaction at pH 1–5.
Results
The methane oxidation reaction was carried out in a stirred autoclave reactor using O2 and H2O2 as oxidants to gain methyl hydroperoxide and methanol as primary products in aqueous solution at 50°C. Other products included formic acid and COx was scarcely detected. AuPdx nanoparticles (x is the Pd-to-Au molar ratio) synthesized by a typical colloidal method were utilized as catalysts (Lopez-Sanchez et al., 2008; Agarwal et al., 2017). Two key parameters, the solution pH and the Pd-to-Au ratio, were investigated to reveal the quantitative relationship between H2O2 utilization and the activity of methane activation. The concentration of H2O2 after reaction was determined by a spectrophotometry method with Ti reagent (Wang et al., 2019). It is important to note that due to the self-decomposition of H2O2, only part of the H2O2 are involved in the activation of methane. The self-decomposition of H2O2 was determined by control experiments that conducted under the same reaction conditions without methane. The amount of H2O2 participated in methane activation (denoted as reactive H2O2) is calculated by the difference between the amount of total consumed H2O2 and the self-decomposed H2O2.
The Effect of pH on CH4 Oxidation
Solution pH is a key parameter that affects the lifetime of H2O2 (Samanta, 2008; Jung et al., 2009). In this work, the initial pH of reaction solution was adjusted from 1.0 to 8.0. Table 1 shows the H2O2 consumption under different reaction conditions. Notably, the H2O2 decomposition in the absence of AuPd colloid is very similar to that with AuPd colloid, indicating the self-decomposition of H2O2 (unselective H2O2 decomposition) is mainly determined by the reaction condition (solution pH) though the presence of AuPd colloid also increases the self-decomposition of H2O2. Figure 1A plots the amount of total consumed H2O2 and reactive H2O2 as functions of solution pH. Interestingly, these two plots are very different from each other. The total consumption of H2O2 increases rapidly as the pH. At pH = 1, only trace of H2O2 is consumed while at pH higher than 6, all H2O2 is consumed. On the contrary, the reactive H2O2 exhibits a volcano plot with pH. The maximum amount of reactive H2O2 is achieved at pH = 3. These results suggest that a proper pH is crucial to transform H2O2 for methane activation. At low pH, the H2O2 is too stable to active methane. At high pH, the H2O2 decompose too fast so that its participation in methane activation is also limited.
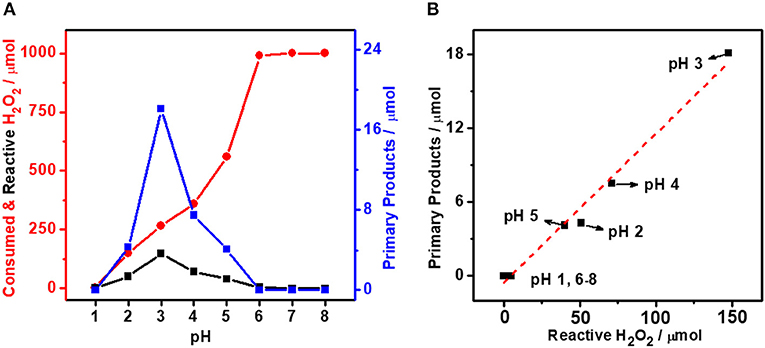
Figure 1. (A) Methane oxidation reaction performance carried out with AuPd0.1 colloid with various pH. (B) Primary products as a function of reactive H2O2 with various pH. Reaction conditions: 3.0 MPa methane, 0.5 MPa O2, 1,000 μmol H2O2, 50°C, 0.5 h, 6.6 μmol metal per reaction.
Furthermore, we also plot the amount of oxygenates as a function of pH. Notably, it shows a similar volcano trend with the reactive H2O2. The maximum productivity (22.9 μmol of total products and 18.1 μmol of primary products) is also achieved at pH = 3. To quantitatively analyze the relationship between methane activation activity and the utilization of H2O2, we further plot the amount of oxygenates as a function of the amount of reactive H2O2. As shown in Figure 1B, a linear relationship is obtained, which clearly reveal the decisive role of reactive H2O2 in the activation of methane. It is indicated that a proper acidity of reaction environment is crucial to maximize the utilization of H2O2 for more efficient oxidation of methane to methanol.
The Effect of Au-Pd Ratio on CH4 Oxidation
It is generally accepted that Au is less active than Pd toward H2O2 decomposition (Choudhary et al., 2006; Li et al., 2011). Our previous study (Yan et al., 2020) suggests that the Au can alter the electronic structure of Pd in bimetallic catalysts to influence their catalytic performance. The tuning of Pd-to-Au ratio in AuPdx is therefore expected to regulate the self-decomposition and thus the utilization efficiency of H2O2. Figures 2a–d show the TEM images of colloidal AuPdx nanoparticles. As can be seen from these figures, AuPdx with different Pd-to-Au molar ratios share the similar spherical morphology and particle size (~3 nm). According to the literatures (Pritchard et al., 2013; Agarwal et al., 2017), AuPd nanoparticles synthesized by colloidal method are bimetallic alloys. The surface electronic structure of AuPd colloid was investigated by XPS in these literatures (Agarwal et al., 2017; McVicker et al., 2020). It turns out that Au and Pd are mainly in their metallic states. Electrons are transferred from Pd to Au, consistent with their electronegativities (Au, 2.54; Pd, 2.20). The charge interaction increases the Au s-state occupancy and indicates the bimetallic alloy formation (Chen et al., 2018; Yuan et al., 2018). In order to verify the formation of AuPd alloy, we herein collect XRD patterns for colloidal AuPdx nanoparticles. As shown in Figure 2e, all AuPdx nanoparticles exhibit symmetrical peak between Au (111) and Pd (111). Besides, as the Pd-to-Au ratio increases, the diffraction peak of AuPdx nanoparticles shifts continuously to a higher angle toward the Pd (111) peak. These results clearly confirm that all AuPdx nanoparticles are single-phase AuPd alloy (Qiao et al., 2014). In addition, the characteristic Au plasmon resonance band (~ 520 nm) decreases with the increasing of palladium content and finally disappears, suggesting the changes in band structure and the alloying of Au and Pd (Figure 2f) (Deki et al., 1999).
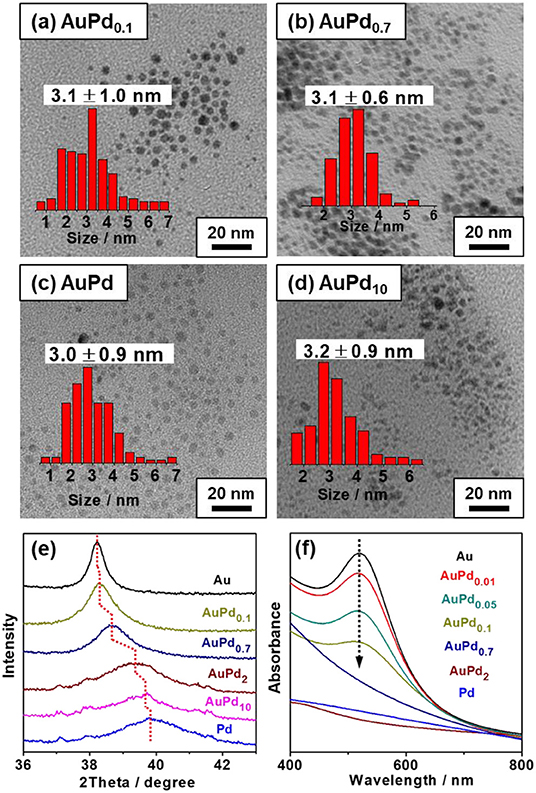
Figure 2. TEM images and particle size distributions of colloidal AuPdx nanoparticles. (a) AuPd0.1, (b) AuPd0.7, (c) AuPd1.0, (d) AuPd10, (e) XRD, and (f) UV-vis spectrum of colloidal AuPdx catalysts.
Figure 3A plots the amount of total consumed H2O2 and reactive H2O2 as functions of Pd-to-Au ratio. A distinct increase in H2O2 total consumption is observed from 115 to 781 μmol with the increasing of palladium content (x value of AuPdx) of the colloid catalyst, confirming Pd is more active than Au for H2O2 decomposition. In terms of reactive H2O2, a volcano curve is observed. The platform of high reactive H2O2 amount is achieved when the x of AuPdx is between 0.1 and 0.7. Further increasing Pd-to-Au ratio would significantly increase the self-decomposition of H2O2 and therefore decrease the amount of reactive H2O2. It is important to note that the amount of primary oxygenates produced on AuPdx also follows the similar trend as reactive H2O2, with AuPd0.1 to AuPd0.7 showing much higher activity than other catalysts. To quantitatively analyze the relationship between methane activation activity and the utilization of H2O2, we further plot the amount of primary oxygenates as a function of the amount of reactive H2O2 over various AuPdx catalysts. The quasi-linear relationship between reactive H2O2 and primary products (Figure 3B) again reveals the decisive role of reactive H2O2 in the activation of methane. It is interesting to note that the slope in Figure 3B (0.11) is almost identical to that in Figure 1B (0.12), suggesting that both pH and the Au-Pd ratio affect the H2O2 utilization and methane oxidation in a similar way. A proper Au-Pd ratio in catalyst is crucial to transform H2O2 for methane activation. Specifically, Au is less active to transform H2O2 while Pd is too active for the decomposition of H2O2. Medium Pd-to-Au ratios, i.e., 0.1–0.7, can successfully balance the activation and self-decomposition of H2O2. They transform most H2O2 to active methane, which leads to maximum productivity of oxygenates.
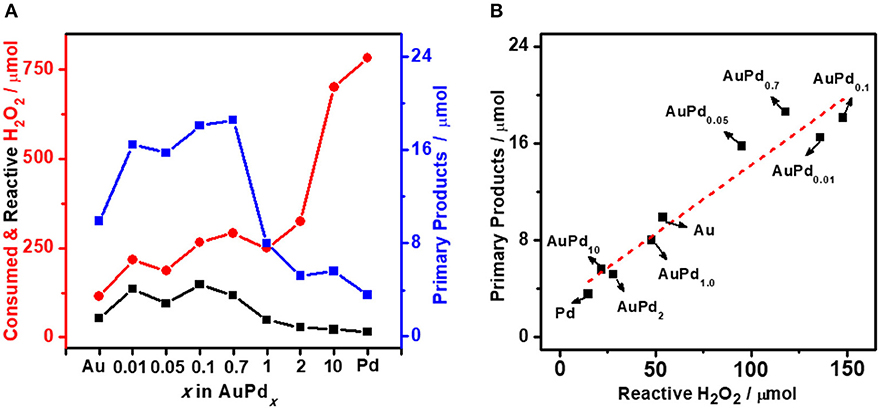
Figure 3. (A) Methane oxidation reaction performance carried out with colloidal AuPdx nanoparticles. (B) Primary products as a function of reactive H2O2 with various x (Au-to-Pd ratio). Reaction conditions: 3.0 MPa methane, 0.5 MPa O2, 1,000 μmol H2O2, 50°C, 0.5 h, initial pH = 3.0, 6.6 μmol metal per reaction.
Discussion
Previous study by Hutchings et al. (Agarwal et al., 2017) suggested that the activation of CH4 by H2O2 is the rate-determining step for the mild oxidation of methane of methanol. It proceeds through a radical mechanism with ·OH radicals generated from H2O2 serving as the radical initial agent to activate CH4 to CH3·. Once CH3· radicals were formed, they readily react with O2 to form CH3OH. It is important to note that the generation of ·OH from H2O2 is dependent on the solution pH (Maezono et al., 2011). At acidic solution (pH <4), H2O2 is relative stable and trends to generate ·OH whereas at basic solution, H2O2 decomposes very fast to generate O2 and H2O (Barreiro et al., 2007). In this study, the optimal pH for methane activation is 3.0, the same as that for the generation of ·OH (Maezono et al., 2011). Taken together, the linear relationship between reactive H2O2 and oxygenates productivity could be linked by the generation of ·OH. The more easily ·OH radicals are generated, the more amount of oxygenates are produced. In terms of Pd-to-Au ratios, the addition of Au into Pd can dilute the surface to reduce the number of sites for O-O scission. Besides, the electron transfer between Au and Pd also alter the adsorption strength of H2O2 with metal surface (Kanungo et al., 2019). A relative mild decomposition of H2O2 to ·OH radicals can therefore be achieved by tuning the Pd-to-Au ratio. Similar results have been reported in the direct synthesis of H2O2 from H2 and O2 (Kanungo et al., 2019). According to the literature, a high concentration of ·OH radicals facilitates the disproportionation to H2O and O2 via a hydrogen transfer mechanism (Plauck et al., 2016). To this end, the regulation of Pd-to-Au ratio can affect the generation and transformation of ·OH, which in return quasi-linearly affects the productivity of oxygenates. It is important to note that at the reaction condition we utilized in this study, the gain factor, defined as mol of oxygenate produced/mol of reactive H2O2, is <1. This result indicates that only part of ·OH radicals participates in the activation of methane. The rest of them likely disproportionate to form H2O and O2 via a hydrogen transfer mechanism (Plauck et al., 2016). Hutchings et al. suggested that reducing the addition of H2O2 could significantly increase the gain factor to 1.2 (Agarwal et al., 2017). It is most likely because decreasing the addition of H2O2 can significantly decrease the amount and therefore the disproportionation of ·OH. To this end, an ideal way to utilize H2O2 for methane activation is to in-situ generate H2O2. Interestingly, this idea is recently realized by Jin et al. (2020). By utilizing a hydrophobically coated zeolite as the catalyst, they successfully generated peroxide from H2 and O2 and keep it close to the AuPd active site, where incoming methane is selectively oxidized to methanol.
Conclusion
We systematically investigated the influence of solution pH and Pd-to-Au ratio of AuPd colloid nanoparticles on the catalytic performance in methane oxidation to methanol. Linear relationships were obtained between the reactive H2O2 and the productivity of oxygenates, demonstrating improved H2O2 utilization efficiency is critical for methane activation. Developing catalysts that can in situ generate H2O2 (or ·OH) and active methane is a promising direction to produce methanol from methane.
Data Availability Statement
All datasets generated for this study are included in the article/supplementary material.
Author Contributions
JF and SZ designed the study. YY and CC performed most of the experiments. YY, CC, SZ, and JF wrote the paper. JL and LX performed some of the experiments and revised the paper.
Funding
This work was supported by National Natural Science Foundation of China (91545113, 91845203, 21703050, and 21802122), China Post-doctoral Science Foundation (2019M662020), Shell Global Solutions International B. V. (PT71423, PT74557).
Conflict of Interest
The authors declare that the research was conducted in the absence of any commercial or financial relationships that could be construed as a potential conflict of interest.
References
Agarwal, N., Freakley, S. J., McVicker, R. U., Althahban, S. M., Dimitratos, N., He, Q., et al. (2017). Aqueous Au-Pd colloids catalyze selective CH4 oxidation to CH3OH with O2 under mild conditions. Science 358, 223–227. doi: 10.1126/science.aan6515
Alvarez-Galvan, C., Melian, M., Ruiz-Matas, L., Eslava, J. L., Navarro, R. M., Ahmadi, M., et al. (2019). Partial oxidation of methane to syngas over nickel-based catalysts: influence of support type, addition of rhodium, and preparation method. Front. Chem. 7:104. doi: 10.3389/fchem.2019.00104
Barreiro, J. C., Capelato, M. D., Martin-Neto, L., and Hansen, H. C. B. (2007). Oxidative decomposition of atrazine by a Fenton-like reaction in a H2O2/ferrihydrite system. Water Res. 41, 55–62. doi: 10.1016/j.watres.2006.09.016
Chen, S.-S., Yang, Z.-Z., Wang, A.-J., Fang, K.-M., and Feng, J.-J. (2018). Facile synthesis of bimetallic gold-palladium nanocrystals as effective and durable advanced catalysts for improved electrocatalytic performances of ethylene glycol and glycerol oxidation. J. Colloid Interface Sci. 509, 10–17. doi: 10.1016/j.jcis.2017.08.063
Cheng, K., Gu, B., Liu, X., Kang, J., Zhang, Q., and Wang, Y. (2016). Direct and highly selective conversion of synthesis gas into lower olefins: design of a bifunctional catalyst combining methanol synthesis and carbon–carbon coupling. Angew. Chem. Int. Ed. 55, 4725–4728. doi: 10.1002/anie.201601208
Cheng, K., Zhou, W., Kang, J., He, S., Shi, S., Zhang, Q., et al. (2017). Bifunctional catalysts for one-step conversion of syngas into aromatics with excellent selectivity and stability. Chem 3, 334–347. doi: 10.1016/j.chempr.2017.05.007
Choudhary, V. R., Samanta, C., and Choudhary, T. (2006). Factors influencing decomposition of H2O2 over supported Pd catalyst in aqueous medium. J. Mol. Catal. A Chem. 260, 115–120. doi: 10.1016/j.molcata.2006.07.009
Cui, X., Li, H., Wang, Y., Hu, Y., Hua, L., Li, H., et al. (2018). Room-temperature methane conversion by graphene-confined single iron atoms. Chem 4, 1902–1910. doi: 10.1016/j.chempr.2018.05.006
Deki, S., Akamatsu, K., Hatakenaka, Y., Mizuhata, M., and Kajinami, A. (1999). Synthesis and characterization of nano-sized gold-palladium bimetallic particles dispersed in polymer thin film matrix. Nanostruct. Mater. 11, 59–65. doi: 10.1016/S0965-9773(99)00019-7
Grundner, S., Markovits, M. A., Li, G., Tromp, M., Pidko, E. A., Hensen, E. J., et al. (2015). Single-site trinuclear copper oxygen clusters in mordenite for selective conversion of methane to methanol. Nat. Commun. 6:7546. doi: 10.1038/ncomms8546
Jiao, F., Li, J., Pan, X., Xiao, J., Li, H., Ma, H., et al. (2016). Selective conversion of syngas to light olefins. Science 351, 1065–1068. doi: 10.1126/science.aaf1835
Jin, Z., Wang, L., Zuidema, E., Mondal, K., Zhang, M., Zhang, J., et al. (2020). Hydrophobic zeolite modification for in situ peroxide formation in methane oxidation to methanol. Science 367, 193–197. doi: 10.1126/science.aaw1108
Jones, C. A., Leonard, J. J., and Sofranko, J. A. (1987). Fuels for the future: remote gas conversion. Energy Fuels 1, 12–16. doi: 10.1021/ef00001a002
Jung, Y. S., Lim, W. T., Park, J. Y., and Kim, Y. H. (2009). Effect of pH on Fenton and Fenton-like oxidation. Environ. Technol. 30, 183–190. doi: 10.1080/09593330802468848
Kanungo, S., van Haandel, L., Hensen, E. J., Schouten, J. C., and d'Angelo, M. F. N. (2019). Direct synthesis of H2O2 in AuPd coated micro channels: an in-situ X-Ray absorption spectroscopic study. J. Catal. 370, 200–209. doi: 10.1016/j.jcat.2018.12.017
Li, J., Staykov, A., Ishihara, T., and Yoshizawa, K. (2011). Theoretical study of the decomposition and hydrogenation of H2O2 on Pd and Au@Pd surfaces: understanding toward high selectivity of H2O2 synthesis. J. Phys. Chem. C 115, 7392–7398. doi: 10.1021/jp1070456
Lopez-Sanchez, J. A., Dimitratos, N., Miedziak, P., Ntainjua, E., Edwards, J. K., Morgan, D., et al. (2008). Au–Pd supported nanocrystals prepared by a sol immobilisation technique as catalysts for selective chemical synthesis. Phys. Chem. Chem. Phys. 10, 1921–1930. doi: 10.1039/b719345a
Maezono, T., Tokumura, M., Sekine, M., and Kawase, Y. (2011). Hydroxyl radical concentration profile in photo-Fenton oxidation process: generation and consumption of hydroxyl radicals during the discoloration of azo-dye Orange II. Chemosphere 82, 1422–1430. doi: 10.1016/j.chemosphere.2010.11.052
McVicker, R., Agarwal, N., Freakley, S. J., He, Q., Althahban, S., Taylor, S. H., et al. (2020). Low temperature selective oxidation of methane using gold-palladium colloids. Catal. Today 342, 32–38. doi: 10.1016/j.cattod.2018.12.017
Meng, Y., Zou, S., Zhou, Y., Yi, W., Yan, Y., Ye, B., et al. (2018). Activating molecular oxygen by Au/ZnO to selectively oxidize glycerol to dihydroxyacetone. Catal. Sci. Technol. 8, 2524–2528. doi: 10.1039/C8CY00319J
Palkovits, R., Antonietti, M., Kuhn, P., Thomas, A., and Schüth, F. (2009). Solid catalysts for the selective low-temperature oxidation of methane to methanol. Angew. Chem. Int. Ed. 48, 6909–6912. doi: 10.1002/anie.200902009
Park, M. B., Park, E. D., and Ahn, W.-S. (2019). Recent progress in direct conversion of methane to methanol over copper-exchanged zeolites. Front. Chem. 7:514. doi: 10.3389/fchem.2019.00514
Plauck, A., Stangland, E. E., Dumesic, J. A., and Mavrikakis, M. (2016). Active sites and mechanisms for H2O2 decomposition over Pd catalysts. Proc. Natl. Acad. Sci. U.S.A. 113, E1973–E1982. doi: 10.1073/pnas.1602172113
Pritchard, J., Piccinini, M., Tiruvalam, R., He, Q., Dimitratos, N., Lopez-Sanchez, J. A., et al. (2013). Effect of heat treatment on Au–Pd catalysts synthesized by sol immobilisation for the direct synthesis of hydrogen peroxide and benzyl alcohol oxidation. Catal. Sci. Technol. 3, 308–317. doi: 10.1039/C2CY20234D
Qiao, P., Zou, S., Xu, S., Liu, J., Li, Y., Ma, G., et al. (2014). A general synthesis strategy of multi-metallic nanoparticles within mesoporous titania via in situ photo-deposition. J. Mater. Chem. A 2, 17321–17328. doi: 10.1039/C4TA02970D
Samanta, C. (2008). Direct synthesis of hydrogen peroxide from hydrogen and oxygen: an overview of recent developments in the process. Appl. Catal. A Gen. 350, 133–149. doi: 10.1016/j.apcata.2008.07.043
Schwach, P., Pan, X., and Bao, X. (2017). Direct conversion of methane to value-added chemicals over heterogeneous catalysts: challenges and prospects. Chem. Rev. 117, 8497–8520. doi: 10.1021/acs.chemrev.6b00715
Shan, J., Li, M., Allard, L. F., Lee, S., and Flytzani-Stephanopoulos, M. (2017). Mild oxidation of methane to methanol or acetic acid on supported isolated rhodium catalysts. Nature 551:605. doi: 10.1038/nature24640
Sushkevich, V. L., Palagin, D., Ranocchiari, M., and van Bokhoven, J. A. (2017). Selective anaerobic oxidation of methane enables direct synthesis of methanol. Science 356, 523–527. doi: 10.1126/science.aam9035
Tang, P., Zhu, Q., Wu, Z., and Ma, D. (2014). Methane activation: the past and future. Energy Environ. Sci. 7, 2580–2591. doi: 10.1039/C4EE00604F
Thomas, C., James, B. D., Lomax Jr, F. D., and Kuhn Jr, I. F. (2000). Fuel options for the fuel cell vehicle: hydrogen, methanol or gasoline? Int. J. Hydrogen Energy 25, 551–567. doi: 10.1016/S0360-3199(99)00064-6
Vernon, P. D., Green, M. L., Cheetham, A. K., and Ashcroft, A. T. (1990). Partial oxidation of methane to synthesis gas. Catal. Lett. 6, 181–186. doi: 10.1007/BF00774718
Wang, Y., Yi, M., Wang, K., and Song, S. (2019). Enhanced electrocatalytic activity for H2O2 production by the oxygen reduction reaction: rational control of the structure and composition of multi-walled carbon nanotubes. Chin. J. Catal. 40, 523–533. doi: 10.1016/S1872-2067(19)63314-0
Williams, C., Carter, J. H., Dummer, N. F., Chow, Y. K., Morgan, D. J., Yacob, S., et al. (2018). Selective oxidation of methane to methanol using supported AuPd catalysts prepared by stabilizer-free sol-immobilization. ACS Catal. 8, 2567–2576. doi: 10.1021/acscatal.7b04417
Yan, Y., Ye, B., Chen, M., Lu, L., Yu, J., Zhou, Y., et al. (2020). Site-specific deposition creates electron-rich Pd atoms for unprecedented C-H activation in aerobic alcohol oxidation. Chin. J. Catal. 41, 1240–1247. doi: 10.1016/S1872-2067(20)63535-5
Yuan, T., Chen, H.-Y., Ma, X., Feng, J.-J., Yuan, P.-X., and Wang, A.-J. (2018). Simple synthesis of self-supported hierarchical AuPd alloyed nanowire networks for boosting electrocatalytic activity toward formic acid oxidation. J. Colloid Interface Sci. 513, 324–330. doi: 10.1016/j.jcis.2017.11.012
Keywords: methane oxidation, AuPd colloid, H2O2 utilization, pH, Au-to-Pd ratio, low temperature
Citation: Yan Y, Chen C, Zou S, Liu J, Xiao L and Fan J (2020) High H2O2 Utilization Promotes Selective Oxidation of Methane to Methanol at Low Temperature. Front. Chem. 8:252. doi: 10.3389/fchem.2020.00252
Received: 16 February 2020; Accepted: 17 March 2020;
Published: 07 April 2020.
Edited by:
Young-Seok Shon, California State University, Long Beach, United StatesReviewed by:
Simon Freakley, University of Bath, United KingdomJiu-Ju Feng, Zhejiang Normal University, China
Copyright © 2020 Yan, Chen, Zou, Liu, Xiao and Fan. This is an open-access article distributed under the terms of the Creative Commons Attribution License (CC BY). The use, distribution or reproduction in other forums is permitted, provided the original author(s) and the copyright owner(s) are credited and that the original publication in this journal is cited, in accordance with accepted academic practice. No use, distribution or reproduction is permitted which does not comply with these terms.
*Correspondence: Shihui Zou, eHVlc2hhbjE5OSYjeDAwMDQwOzE2My5jb20=; Jie Fan, amZhbiYjeDAwMDQwO3pqdS5lZHUuY24=
†These authors have contributed equally to this work