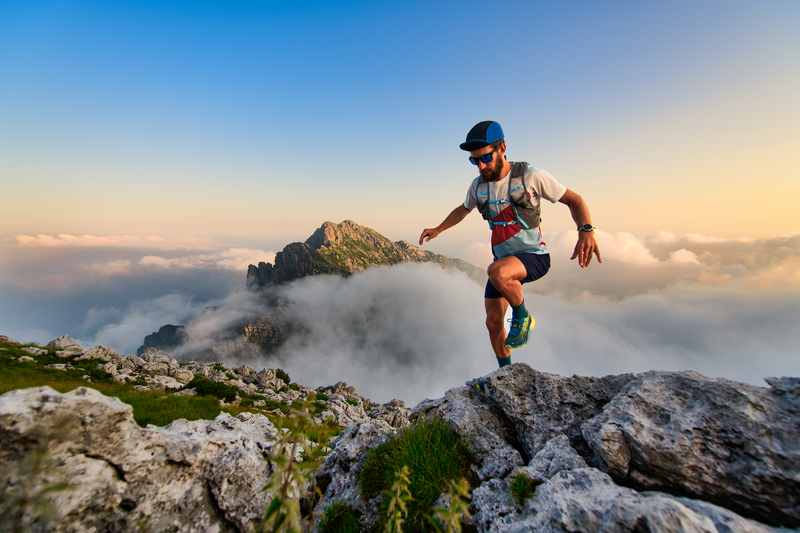
95% of researchers rate our articles as excellent or good
Learn more about the work of our research integrity team to safeguard the quality of each article we publish.
Find out more
REVIEW article
Front. Chem. , 03 April 2020
Sec. Nanoscience
Volume 8 - 2020 | https://doi.org/10.3389/fchem.2020.00219
This article is part of the Research Topic Nanozymes: From Rational Design to Biomedical Applications View all 14 articles
In recent years, the rapid development of nanoscience and technology has provided a new opportunity for the development and preparation of new inorganic enzymes. Nanozyme is a new generation of artificial mimetic enzyme, which like natural enzymes, can efficiently catalyze the substrate of enzyme under mild conditions, exhibiting catalytic efficiency, and enzymatic reaction kinetics similar to natural enzymes. However, nanozymes exist better stability than native enzymes, it can still maintain 85 % catalytic activity in strong acid and alkali (pH 2~10) or large temperature range (4~90°C). This provides conditions for designing complex catalytic systems. In this review, we discussed the enzymatic attributes and biomedical applications of gold nanoclusters, including peroxidase-like, catalase-like, detection of heavy metal ions, and therapy of brain and cancer etc. This review can help us understand the current research status nanozymes.
The natural enzyme is a kind of biocatalyst that is closely related to many life activities (Arnold et al., 2001). Similar to other catalysts, the presence of natural enzymes can greatly speed up the reaction and sometimes participate in the reaction, but the enzyme itself does not change before or after the reactions (Wilhelmová, 1996). In addition, it exhibits high catalytic efficiency, mild reaction conditions and high specificity (Sheldon, 2007). However, lots of natural enzymes are proteins, which are susceptible to high temperature, acidic or alkaline, causing loss of catalytic activity (Chang, 2013). In addition, natural enzymes presented in living organisms are difficult to purify, relatively expensive, and not easily transported and stored (Scopes, 2013). To overcome the limitations of instability and high cost for natural enzymes, researchers are looking for different ways to prepare mimetic enzymes to replace natural enzymes (Chen et al., 2010; Yang et al., 2016). Until now, different types of mimic enzymes have been designed and used in many biological fields, such as immunoassay (Lequin, 2005), glucose detection (Song et al., 2010), heavy metal ion detection (Kim et al., 2001), free radical protection (Barzegar and Moosavi-Movahedi, 2011), and tissue engineering (Griffith and Naughton, 2002). Host-guest chemistry (Wan et al., 2006) and supramolecular chemistry (Steed and Atwood, 2013) lay an important theoretical foundation for mimic enzyme. In essence, the basic meaning of host-guest chemistry comes from the interaction between enzyme and substrate, which is embodied in the complementary spatial and electronic arrangement of the binding site between the subject and the guest (Spichiger-Keller, 2008). This host-guest complementarity is similar to the combination of the nanozymes and the substrate. Based on non-covalent bond interactions, such as electrostatic interactions, hydrogen bonding and van der Waals forces (Pasternack et al., 1998; Wei and Wang, 2013), supramolecules are produced by the combination of substrate and receptor (Geim and Grigorieva, 2013). When receptors combine with complex ions or molecules, a supramolecule with stable structure and properties is formed, which has the functions of molecular recognition, catalytic and selective output. Host-guest chemistry and supramolecules chemistry are important theoretical weapons for the simulation of artificial enzymes.
At present, the ideal traditional enzyme systems include cyclodextrin (Del Valle, 2004), cyclophane (Gleiter and Hopf, 2006) and cyclic aromatic hydrocarbons (Tsipis and Tsipis, 2003). The chemical composition of traditional mimic peroxidase-like activation enzyme is non-protein, but these enzymes have similar catalytic performance to natural enzymes (Meeuwissen and Reek, 2010). These traditional mimetic enzymes are superior to natural enzymes in thermal stability and acid-base resistance, but their shortcomings, such as too-complex structure, difficulties in separation, single catalytic active sites, and low catalytic efficiency, still stunt their development progress (Liu Q. et al., 2017). With the development of nanomaterials, the researches on the catalysis of nanomaterials have also achieved many new results (Yin and Talapin, 2013). Nanozymes have the dual identities of enzymes and nanomaterials (Roduner, 2006). Unlike natural enzymes or traditional artificial enzymes, nanozymes (Wang et al., 2013; Li et al., 2015; Zhou et al., 2017; Fan et al., 2018; Qu et al., 2018; Chen et al., 2019; Huang et al., 2019a,b; Jiang et al., 2019; Liang and Yan, 2019; Liu et al., 2019; Mu et al., 2019a,b; Wu et al., 2019; Xu et al., 2019; Yan et al., 2019) have many physical and chemical properties, besides their catalytic functions (Senanayake et al., 2013). For example, Fe3O4 and CdS nanoparticles not only have the catalytic functions of peroxidase and superparamagnetic activities, but also have the property of luminescence (Liu et al., 2011). The enzymatic activity of nanozymes is not only related to its composition, crystal form and structure, but also to its surface properties (Lin et al., 2014a). Take gold nanoclusters (Au NCs) as an example, the combination of certain small molecules with Au NCs can change the surface microenvironment, resulting in changes in the catalytic activities of Au NCs (Jin, 2010). Wang et al. found that the surface modification of Au NCs can affect its catalytic activity (Yuwen et al., 2014). The Au NCs were modified with amino and citric acid respectively to make positive and negative charges on the surface, and then catalytically oxidize ABTS (Erel, 2004) (negatively charged) and TMB (Ding et al., 2018) (positively charged) to detect its catalytic activity. The amino and citric acid modified Au NCs were found to have high affinity to the substrates ABTS and TMB. Not only small molecules can be combined with Au NCs, inorganic nanoparticles, metal ions and biomacromolecules (such as DNA, RNA) can also be combined with it to change the surface microenvironment of Au NCs, thereby changing its catalytic activity. Compared with other nanomaterials with simulating peroxidase, gold nanoclusters have the advantages of small size, good stability, good biocompatibility, and are more prominent in the application of biological analysis. However, the potential of Au NCs as enzyme mimics is easily limited by the low catalytic activity at neutral environment. In addition, since the surface atom is the key catalytic sites for gold clusters, modification of nanozymes with various coating molecules may block their active sites, reducing or inhibiting their enzymatic activity. This review details the enzymatic properties of gold nanoclusters and their applications in biomedicine in recent years.
Peroxidase (Gao et al., 2007) is a kind of natural enzyme that have catalytic oxidation effects on hydrogen peroxide. The establishment of an analytical method involving H2O2 is of great significance in analytical chemistry and clinical medicine. In recent years, Au NCs have been reported to have peroxidase-like properties and are used in the fields of bionics, biosensing, and biomedicine (Feng et al., 2017; Liao et al., 2017). First, H2O2 can be adsorbed on the surface of gold nanomaterials, and the O–O bonds of H2O2 may be decomposed into dihydroxy radicals; at the same time, the generated hydroxyl radicals may be stabilized by gold nanomaterials through partial electron exchange interactions. This may contribute to their catalytic capabilities. Figure 1 shows the adsorption and decomposition of H2O2 on Au(111) under different pH conditions (Li et al., 2015). Under neutral conditions, H2O2 adsorbed on the surface of Au (111) can undergo acid decomposition and alkali decomposition. According to the principle of lowest energy, alkaline decomposition is more inclined under neutral conditions (Figure 1A) (He et al., 2012). It is worth noting that under these conditions, the adsorbed O* cannot generate O2 under the high energy barrier of 1.42 eV (Wu et al., 2019). Under acidic conditions, the decomposition pathway of H2O2 is similar to the decomposition of alkalis under neutral conditions. First, OH* is generated and then O* and H2O* are generated. The generated O* can extract H from the substrate. Therefore, under acidic and neutral conditions, Au (111) has peroxidase activity (Figure 1B). Ding et al. used 3, 3′, 5,5′-tetramethylbenzidine (TMB) as a substrate and found that histidine-modified gold clusters (His-Au NCs) have peroxidase-like activity. When H2O2 is present, peroxidase can catalyze the oxidation of TMB. Reaction, when His-Au NCs is mixed with H2O2 and TMB, the solution rapidly changes from colorless to blue, and the maximum absorption wavelength of the mixture is 652 nm. This is due to the TMB is oxidized to oxTMB and the solution is blue. These results indicated that His-Au NCs are capable of oxidizing TMB to develop color and have peroxidase-like properties (Liu Y. et al., 2017). Lin et al. compared the catalytic activity of unmodified Au NCs and studied the effect of amino-modified gold clusters (NH2-Au NCs) and citric acid-modified gold clusters (itrate-Au NCs) on the substrates ABTS and TMB. It was found that the surface unmodified Au NCs has the highest peroxidase activity. NH2-Au NCs have higher catalytic activities for ABTS than citrate-Au NCs, while NH2-Au NCs have lower catalytic activity for TMB than itrate-Au NCs. For the surface modified by Au NCs has different charges, it exhibits different variation, and the ability to adsorb the oxidized substrate is different, thus showing the difference in catalytic activity. The biocompatibility of Au NCs was adjusted by selecting different ligands (Liu et al., 2016). Wang's team prepared bovine serum albumin (BSA) modified Au NCs. In order to improve the peroxidase- like activity of Au NCs (Wang et al., 2011). Wen et al. used the horseradish peroxidase properties of gold nanoclusters to detect H2O2 (Wen et al., 2011). Jiang et al. discovered and applied precious metal nanoclusters. They report that gold chains of ferritin iron (Au-Ft) can produce a blue reaction by catalyzing the oxidation of TMB by H2O2. Compared to the native enzyme, Au-Ft exhibits higher activity and pH, temperature range, and the catalyzed reaction follows typical Mie kinetics. The lower Km value (0.097 μM) was exhibited by the Au-Ft kinetic parameters, and the specific activity for TMB oxidation exceeded HRP. According to these findings, Au-Ft was used as a peroxide mimic enzyme to perform glucose spectrometry. Photometric analysis the system exhibited acceptable repeatability and high specificity (Liu J. et al., 2017).
Figure 1. Calculated reaction spectrum (unit: eV) of H2O2 decomposition on Au surface: (A) neutral, (B) acidic, (C) basic [reproduced from Li et al. (2015) with permission from The Elsevier].
Catalase is an important enzyme that prevents oxidative damage of cells by reactive oxygen species (Glorieux and Calderon, 2017). For Au NCs, Au2+ is first reduced by a H2O2 to form Au+, which is accompanied by the production of protons and O2. After that, another H2O2 can be combined with oxygen vacancies to oxidize Au+ to Au2+ and release H2O. This completes the simulation of hydrogen peroxide. Most nanomaterial-based peroxidase mimetics typically exhibit enzymatic activity under alkaline conditions rather than under physiological conditions (Góth et al., 2004; Glorieux and Calderon, 2017). Under the basic conditions of OH pre-adsorption, H2O2 first transfers one H* to the pre-adsorbed OH to form HO and H2O*; then, HO gives one H to the other H2O2 and finally produces H2O * and O2 * (Figure 1C). Therefore, hydrogen peroxide-based activity can be observed under alkaline conditions (Li et al., 2015). He et al. (2013) demonstrated the intrinsic catalase activity of Au NCs using electron spin resonance spectroscopy combined with spin trapping and spin labeling. Under normal and basic conditions, Au NCs exhibit inherent catalase catalytic activity because Au NCs can convert H2O2 to H2O and O2. However, under acidic conditions, the catalase-like activity of Au NCs is significantly reduced, and once trapped in organelles, such as endosomes (pH≈5.5) and lysosomes (pH≈4.8), hydroxyl groups are produced. Free radicals (·OH) can easily induce apoptosis. Therefore, the catalase-like activity of Au NCs is limited (He et al., 2013). To change this situation, Liu et al. studied amine-terminated macromolecularly encapsulated gold nanoclusters (Au NCs-NH2). Au NCs-NH2 exhibits good catalase activity at physiologically acidic pH values (Liu C. P. et al., 2017). Figure 2A is a schematic diagram showing the enzymatic activity of Au NCs-NH2, which can catalyze the production of O2 by H2O2 by catalase activity. They changed the different groups of dendrimers, added Au NCs-OH and Au NCs-COOH, and studied the relative H2O2 consumption of Au NCs-NH2, Au NCs-OH and Au NCs-COOH under different solution pH values. A comparison of the amounts, they still have significant catalase-like activity at pH 4.8-7.4 (Figure 2B). By comparing the catalytic activities of Au NCs-NH2, Au NCs-OH and Au NCs-COOH at various concentrations of H2O2 at pH 4.8 and pH 7.4, the effect of Au NCs-NH2 was significantly better (Figure 2C). Fan et al. synthesized a derivative protein (apoFt) as a nanoreactor to obtain Au-apoFt with adjustable size and uniform dispersion. The catalytic activity of both was observed at pH and temperature compared to natural catalase. As the pH and temperature increase, the enzyme activity of Au-apoFt increases. The increase in pH may be due to the presence of OH- which promotes the dehydrogenation step of water in the catalytic reaction, showing an increase in enzyme activity. With rising of temperature, the increase in the enzyme activity of Au-apoFt may be due to an increase in the rate of molecular motion, which allows more H2O2 to adsorb to the surface of the Au nanoclusters, showing an increase in catalytic activity. Liu et al. found that bovine serum albumin-protected gold nanoclusters (BSA-Au NCs) can sensitively measure H2O2 concentration (Figure 2D). Red BSA-Au NCs have no catalytic activity for TMB in the presence of oxygen but no light, and have catalytic activity in the presence of oxygen and light (Figure 2E); blue BSA-Au NCs have a slight catalytic activity for TMB in the presence of oxygen but no light, and have strong catalytic activity when oxygen and light coexist (Figure 2F). The experiment shows that light can stimulate the catalytic activity of Au NCs (Wang et al., 2015).
Figure 2. Catalase-like of gold clusters (A) Au NCs-NH2 can catalyze H2O2 to produce H2O and O2 through catalase-like activity. (B) Consumption of H2O2 by Au NCs-NH2, Au NCs-OH and Au NCs-COOH at the same concentration (0.02 × 10−3 M). (C) The catalytic activities of different concentrations of Au NCs-NH2, Au NCs-OH and Au NCs-COOH on H2O2 (0.1 × 10−3 M) at pH 4.8 and 7.4 [reproduced from Liu C. P. et al. (2017) with permission from The Small]. (D) Effects of different concentrations of Ag+ on catalase activity of BSA-Au NCs. Red BSA-Au NCs [reproduced from Chang et al. (2016) with permission from The Elsevier]. (E) And blue BSA-Au NCs. (F) Catalyze TMB under different conditions: (a) under visible light, (b) in the presence of Au NCs, (c) in the presence of Au NCs under visible light [reproduced from Wang et al. (2015) with permission from The Elsevier].
Glucose oxidase (GOD) is widely distributed in animals and plants and microorganisms, and can specifically catalyze the production of glucose into gluconic acid and hydrogen peroxide under aerobic conditions (Gibson et al., 1964; Wilson and Turner, 1992; Bankar et al., 2009; Luo et al., 2010). At present, the application fields of GOD are constantly expanding, and the demand in domestic and foreign markets has increased dramatically. Low yield, low enzyme activity, and complex detection methods are the limiting factors for GOD industrialization. A lot of work has been done at home and abroad and significant progress has been made. Recent studies have proved that gold clusters have excellent GOD-like enzyme activity. Luo et al. reported an interesting autocatalytic, self-limiting system that controls the controlled growth of Au NCs with GOD-like (Luo et al., 2010). In this system, Au NCs can serve as both seeds and catalyst, that is, the Au NCs catalyzed glucose oxidation in situ produced H2O2, and induced the Au NCs seeds in the presence of gold chloride ions. More importantly, the growth of Au NCs is internally regulated by two negative feedback factors, the reduced size-dependent activity of Au NCs and the glucose-induced surface passivation of the products, leading to rapid self-limiting systems. Pandey et al. (2007) used chemical synthesis to covalently combine GOD with the surface of gold nanoclusters to form a GOD-Au NCs complex, which improves the catalytic activity of GOD, improves stability, and enhances the enzyme response temperature and pH durability (Xia et al., 2013). However, this method only improves the performance of the enzyme and does not fundamentally solve the problem. The activity of the enzyme is still restricted by a series of factors, and other substances are introduced into the reaction system, which makes the entire system more complicated. Rossi et al. found that in the presence of O2, glucose can be catalyzed by “bare” gold nanoclusters to produce gluconic acid and H2O2 (Pina et al., 2011). Based on the promotion of alkali and the production of H2O2, they proposed the mechanism of molecular activated gold catalysis (Figure 3A). The surface of the gold atom interacts with the hydrated glucose anion to form electron-rich gold, which effectively activates molecular oxygen through nucleophilic attack. O2 and gold intermediates Au2+ + -O or Au + -O can act as a bridge for the conversion of electrons from glucose to hydrogen peroxide. Thereby the final reaction product is formed. Lin et al. studied mesoporous silica-encapsulated gold nanoclusters (EMSN-Au NPs) with GOD properties through UV-Vis (Figures 3B,C) (Lin et al., 2014a). First, the GOD mimicking activity of EMSN-Au NPs in solution was evaluated. Glucose is catalyzed by them in the presence of O2 to produce gluconic acid. During the experiment, they used methyl red to detect the change in pH of the solution, as shown in Figure 3D. The results further confirmed that gluconic acid was indeed produced in the reaction catalyzed by EMSN-Au NPs. Superoxide dismutase (SOD) is a kind of antioxidant metal enzyme in vivo. It can catalyze superoxide anion free radical disproportionation to generate hydrogen peroxide and oxygen, which is very important in anti-oxidation. Gold nanoclusters decompose O2− into molecular oxygen (O2) and hydrogen peroxide (H2O2) through a cyclic redox electron transfer mechanism, thereby eliminating O activity. Weiwei et al. verified the SOD activity of Au NPs through ESR experiments (Figure 3E) (He et al., 2013).
Figure 3. Glucose oxidase-like and superoxide dismutase-like of gold clusters (A) Catalytic mechanism of GOD-like enzymes of Au nanoparticles [reproduced from Lin et al. (2014a) with permission from The Wiley-VCH]. (B) The oxidative decomposition of glucose into gluconic acid, absorbance at 505 nm. (1) Glucose only (2) EMSN-Au NPs alone (3) Glucose and EMSN-Au NPs (4) Glucose and EMSN-Au NPs. (C) In the presence of different concentrations of EMSN-Au NPs, glucose is oxidative decomposed into gluconic acid, the absorbance at 505 nm. (D) Typical photo of methyl red solution of glucose and EMSN-Au NPs [reproduced from Lin et al. (2014a) with permission from The Elsevier]. (E) Au NPs scavenge SOD-like activity of superoxide [reproduced from He et al. (2013) with permission from The Elsevier].
The enzymatic activities of nanomaterials are related to size (Mavrikakis et al., 2000; Lopez et al., 2004; Molina and Hammer, 2005; Miller et al., 2006; Zhou et al., 2010; Brodersen et al., 2011). It is possible to regulate the activity of nanomaterials by controlling their size, which has been confirmed in many studies. For gold nanomaterials as an example; the enzyme-like activity of gold nanoclusters is stronger than that of gold nanoparticles. Li et al. compared the enzyme-like activities of gold nanozymes with different structures and morphologies, including Au nanoclusters, Au nanoparticles, and Au nanotubes. At the same specific surface area, the Au nanoclusters had the strongest enzyme-like activity, while the Au nanotubes had the weakest enzyme-like activity. Through the study and analysis, they concluded that the differences in the enzyme-like activity of materials may be related to the differences in the crystal planes (Li et al., 2015). Corma et al. supported gold atoms on functionalized carbon nanotubes and explored their catalytic properties for phenol. They have catalytic activity equivalent to that of thiol oxidase. The catalytic activity also decreases with the increase of the size of the gold cluster, until it almost disappeared. According to theoretical calculations, smaller gold clusters can activate thiophenol and O2 and are therefore active, while larger nanoparticles are inactivated by alkoxides and lose their activity (Corma et al., 2013). Tamao et al. tested the catalytic performance of gold nanoparticles on CO under different loads (Figure 4A). Compared with ZrO2, TiO2 and CeO2, Al2O3 has a lower catalytic activity (Ishida et al., 2008). Oxygen vacancies can be formed at the peripheral interface of the Au particles. When gold nanoclusters are deposited on carbon materials and polymers, they lose their catalytic activity at temperatures above 120°C In the CO oxidation reaction, the catalytic activity of glucose oxidation observed on Au catalysts is more affected by Au particle size than by carrier properties, and is related to the turnover frequency (TOF) of Au atoms on the surface (Figure 4B). Rossi et al. studied the effect of particle size on the catalytic activity by using gold clusters (3–10nm) dispersed in water (Comotti et al., 2010). According to their research, the catalytic activity of glucose oxidase increases with decreasing particle size (Figure 4C). They first showed that gold nanoclusters (Au NCs) can catalyze the oxidation of glucose in the presence of O2 to produce gluconic acid and H2O2. In contrast, other metal nanomaterials tested, such as Cu, Ag, Pd, and Pt, did not show significant oxidase-like activity under similar conditions (Comotti et al., 2010; Quan et al., 2015). Lin et al. compared the CAT activity of several clusters experimentally, and the results showed that the CAT activity of Pt and Pd was better than that of Au and Ag. Otherwise, the SOD-like and CAT-like activity of Au and Pt nanozymes increased under alkaline conditions and decreased under acidic conditions (Lin et al., 2014b). Tsunoyama et al. prepared a group of monodisperse gold clusters (Au: PVP) through seed-mediated growth in the presence of polyvinylpyrrolidone (PVP) (Tsunoyama et al., 2006). The catalytic activity of Au: PVP clusters on hydroxybenzyl alcohol decreases with increasing core size (Figure 4D). Panigrahi et al. studied a core-shell Nano composite (R-Au) (Figure 4E) and derived the relationship between reduction rate and gold nanoclusters size (Panigrahi et al., 2007). The reaction rate decreases with increasing particle size. When the particle size is increased to 32 nm, as the particle size increases, the decline rate tends to be gentle. They believe that as the particle size increases, the decrease in catalytic performance is due to the increase in particle surface roughness. Therefore, the smaller the particle size, the higher the catalytic activity (Figure 4F).
Figure 4. Effect of support and particle size on gold cluster activity (A) Catalytic performance of different supported gold nanoclusters for CO. (B) Turnover frequency of glucose oxidation [reproduced from Ishida et al., 2008 with permission from The Wiley-VCH]. (C) Relationship between conversion rate and particle size [reproduced from Comotti et al. (2010) with permission from The Wiley-VCH]. (D) Relationship between the catalytic activities of Au: PVP clusters for the aerobic oxidation of hydroxybenzyl alcohol and the nuclear size [reproduced from Tsunoyama et al. (2006) with permission from The Elsevier]. (E) Graph of reduction rate as a function of R-Au particle size. (F) Relationship between different volumes and reaction rates [reproduced from Panigrahi et al. (2007) with permission from The American Chemical Society].
At present, heavy-metal contamination has caused great threats to the human health and our living environment. It is of great significance for the detection of heavy metals, with high selectivity of heavy metals (Bhan and Sarkar, 2005; Gallardo et al., 2014; Martin and Griswold, 2018). It is well-known that mercury is a toxic heavy metal and widely found in the environment (Ercal et al., 2001). Mercury ion (Hg2+) is the most common heavy metal ions. Even at very low concentrations, its destructive properties can affect the brain, god system and kidney (Bhan and Sarkar, 2005). Therefore, it is necessary to establish a fast, simple and sensitive method to detect Hg2+ in the environment. Zhu et al. found that Hg2+ has a selective inhibitory effect on the peroxidase activity of BSA-Au clusters (Zhu et al., 2013). The effect of common metal ions on the catalytic activity of BSA-Au was investigated using fluorescence quenching (Figure 5A). At the same concentration, Na+, Fe3+, Co2+, Ag+, Mg2+, Al3+, K+, Ca2+, Cr3+, Ni2+, Cu2+, Zn2+, Cd2+, Pb2+ to BSA-Au NCs have no effect on peroxidase-like activity. Hg2+ can inhibit the peroxidase-like activity of BSA-Au NCs and hardly catalyze the color reaction of TMB and H2O2 (Figure 5B). Similarly, the absorption spectrum of the reaction solution at 652 nm can also indicate that Hg2+ can inhibit the catalytic activity of BSA-Au NCs (Figure 5C, curve 2). Chelation of EDTA with Hg2+ can reduce the inhibitory effect of Hg2+ on the catalytic activity of BSA-Au NCs (Figure 5C, curve 3). Because mercury is easily complexed with sulfur, mercury ions (Hg2+) can combine with cysteine through Hg-S bond to form Hg-Cys complex. The affinity of cysteine to Hg2+ was significantly higher than that of other metal ions. Based on this mechanism, Ding et al. constructed fluorescence quenching of citrate-modified Au NCs to detect Hg2+ in tap water (Ding et al., 2012). Then, Qi et al. reported a probe that can paper-based visualization of Hg2+ based on conjugates of Tb3+/BSA-Au NCs. The probe can be highly complexed with Hg2+ via Hg-S bonds, and therefore has excellent selectivity. The method is simple and easy to operate, with only a ultraviolet lamp needed, which can be greatly promoted in practical applications (Qi et al., 2015). Using similar sensing mechanism, Lin et al. constructed a method to detect Hg2+ and methylmercury in seawater based on Lys protected Au NCs probe, and Xu et al. also used lysozyme-modified Au NCs to visually detect Hg2+ in water by visual and fluorescent colorimetry (Xu et al., 2015). The Zhu working group provided us with a colorimetric method that can detect Hg with high sensitivity and selectivity. They also detected of different working fluids and the influence of common metal ions on the catalytic activity. Finally, Hg2+ can specifically interact with Au+ to inhibit enzyme activity (Figure 5D) (Zhu et al., 2013). Liu et al. combined the peroxidase-like nanozymes activity of gold nanoclusters with the double-stranded nature of amino acids, and proposed a simple, sensitive and selective method for the detection of Cu2+ and histidine (His). The addition of different concentrations of Cu2+ can inhibit the peroxidation of histidine-gold nanoclusters (His-Au NCs) to varying degrees (Figure 5E). The absorbance of the solution at 652 nm gradually decreases with increasing Cu2+ concentration, so this method can be used to detect the concentration of Cu2+ in the solution. According to this calibration curve, Cu2+ can be measured with high sensitivity (Figure 5F) (Liu Y. et al., 2017). Although gold nanocluster probes with enzyme activity have been widely developed in recent years, many problems still need to be solved. First, most of the probes with enzyme activity controlled by metal ions are POD activity, while few probes are available for other types of enzyme activities. In addition, the sensor mechanism of the probes is relatively single, and more types of mechanisms need to be developed to design the probes. Finally, there is little research on the application in biological matrix or in vivo, which is very important for the biological application of enzyme activity.
Figure 5. Detection of heavy metal ions (A) Schematic diagram of Hg2+ detection principle. (B) The effect of different metal ions on the peroxidase like activity of BSA-Au NCs. (C) The inhibition of Hg2+ on the activity of BSA-Au NCS peroxidase under different conditions [reproduced from Zhu et al. (2013) with permission from The Elsevier]. (D) Hg2+ can specifically interact with Au+ and inhibit enzyme activity [reproduced from Chang et al. (2016) with permission from The Elsevier]. (E) Effect of different concentrations of copper ions on the absorption strength of solution. (F) A/A0 plot plotted at the concentration of Cu2+. A and A0 are absorbance at 652 nm with and without copper ions [reproduced from Liu Y. et al. (2017) with permission from The Elsevier].
Inorganic anions are widely present in ecosystems, but it should be noted that most inorganic anions have two-sided effects on the ecological environment and human health (Velizarov et al., 2004). Shojaeifard et al. tested environmental water and CN in human serum based on a combination of Au NCs and copper (II)-phthalocyanine complexes. Under the condition of CN−, the binding of Au NCs to the copper (II)-phthalocyanine complex is destroyed, so the fluorescence of the copper (II)- phthalocyanine complex quenched by Au NCs is restored, thus forming a stable [Au (CN)2]−. The method has good selectivity, high sensitivity and is suitable for popularization (Shojaeifard et al., 2016). Liu et al. also constructed a method based on Au NCs to detect CN− in environmental water samples, and successfully used to detect CN− in food sand samples and biological samples (Liu et al., 2010). Xiong et al. synthesized BSA-Au NCs and successfully detected Cl− in tap water with a detection limit of 0.50 μmol/L (Xiong et al., 2015). Wang et al. used glutathione-coated Au NCs as probes to construct a method for detecting I− in water with a detection limit of 400 nmol/L. This method can selectively identify I− from 12 common anions such as F−, Cl−, Br− (Yang et al., 2014). At the same time, Chang group used DNA as template to synthesize gold/silver nanozymes. Clusters were used to detect S2− in hot spring and seawater samples. This method specifically recognized S2− from Au3+, Ag+ and DNA in the presence of NaBH4. The quenching mechanism is that S can interact with gold and silver atoms, thus resulting in changes in the conformation of the template DNA (Chen et al., 2011). At present, gold clusters have been used to detect anions in vitro more thoroughly and sensitively, but few studies have been done in combination with organisms. The biological environment is more complex, so anion detection in vivo will be a challenge. In addition, the role of gold clusters in organisms should not only be a function of detection, but also require us to develop more properties of gold clusters for the diagnosis and treatment of organisms.
Nanoprobe with enzymatic properties have been attracting increasing attention in early screening and diagnosis of cancer (Schaller and Graf, 2004; Spichiger-Keller, 2008; He et al., 2010; Chi et al., 2012; Li and Xiaogang, 2015). In order to achieve the specificity and high accuracy of tumor detection, it was necessary to design and prepare an enzyme-simulated nanoprobe with tumor targeting, high enzyme activity and containing luminescent properties. Hu et al. described the folate receptor based gold nanoclusters (NCs-FA), which are novel luciferase mimic nanoprobes with high stability, low cytotoxicity and high enzyme activity. O-Phenylenediamine (OPD), 3-amino-9-ethylcarbazole (AEC), 5-aminosalicylic acid (5ASA) and 3,3,5,5-tetramethylbenzidine (TMB) can be used NCs-FA catalyzes to produce red, brown, brown, and blue. Experiments prove that NCs-FA nanoprobe has peroxidase activity (Figure 6A). To visualize the uptake of NCS-FA nanoprobes by cells, MCF-7 and HepG2 cells were stained with NCs-FA nanoprobes (NC) and observed under a focused laser microscope (Figure 6B) (Hu et al., 2014). The probes (NC) stained MCF-7 and HepG2 tumor tissues and observed them by fluorescence microscopy (Figure 6C). It confirmed that NCs-FA nanoprobes target tumor cells via FR. Au NPs have low anticancer activity and are widely used in drug carriers, biological imaging, and other fields. The new selenium-containing molecule (EGSE-TME) has low anticancer activity, but the combination of NCs-FA and EGSE-TME has produced a system with good anticancer activity. Li et al. synthesized Au NP/Se. In order to explore its cytotoxicity, tumor mice were injected with PBS, Au NP/citrate, EGSe-tMe, and Au NP/Se on day 0 and 8, respectively. Compared with the tumor volume of the control group, mice treated with Au NP/ citrate, EGSe-tMe had less obvious tumor growth inhibition effect, and however, mice treated with Au NP/Se showed tumor growth strong inhibitory effect (Figure 6D). There was no significant cha of mice treated with Au NP/Se (Figure 6E). And photographs of mice after administration of different drugs on day 10 were observed, which showed that the systemic toxicity of Au NP/Se was low (Li et al., 2016). However, there are still some problems in the development of nanoprobe for tumor microenvironment. Further binding to ligands may help to reduce toxicity and guide targeting, but can affect the catalytic activity and subsequent metabolism of the nanozymes, as well as the microenvironment of the organisms. Although nanoprobes have achieved good results in animal experiments, the differences between animals and humans are huge, and their clinical applications need to be further developed and utilized.
Figure 6. Gold clusters for tumor treatment. (A) NCs-FA and HRP catalyze the color contrast of OPD, AEC, 5ASA, DAB, and TMB. (B) MCF-7 cells and HepG2 cells were stained with NCs-FA under a confocal laser microscope [reproduced from Hu et al. (2014) with permission from The Ivy Publisher]. (C) Fluorescence imaging of NCs-FA stained MCF-7 and HepG2 tumor tissues. (D) Relative tumor volume of mice after different drug treatments. (E) Body weight changes in mice after different drug treatments [reproduced from Li et al. (2016) with permission from The American Chemical Society].
The toxicity of nanozymes has caused widespread concern (Zhang et al., 2018). Liu et al. found that amino-terminated gold nanoclusters (Au NCs-NH2) have low cytotoxicity and can protect primary neurons from oxidative damage (Liu et al., 2016). The peroxidase-like activity of Au NCs-NH2 is inhibited by the polymerized 3-amines, and thus exhibits catalase-like activity, which decomposes H2O2, thereby providing neurons with protection against oxidative damage (Figure 7A) (Mukherjee et al., 2010). Compared to the control, H2O2 excitation resulted in significant cell death and less red fluorescence. Neuronal cells were pre-treated with Au NCs-NH2 and showed red fluorescence similar to that of the control group after challenge with H2O2. The same is true for neurons treated with Au NCs-NH2 alone, further demonstrating the low cytotoxicity of Au NCs-NH2 (Figure 7B) (Wang et al., 2013). Cell viability in different treatment groups was determined by MTT assay and treatment with H2O2 alone (100 × 10−6 or 200 × 10−6 M) resulted in a significant decrease in neuronal cell viability. Pretreatment with Au NCs-NH2 followed by challenge with H2O2, the cells remained viable and the results were similar to the untreated controls. This indicates that pretreatment of primary neuronal cells with Au NCs-NH2 have resistance to H2O2 induced toxicity (Figure 7C) (Huang et al., 2004). The catalase-like activities of Au NCs-NH2 and Au NCs-OH prepared from different end groups of dendrimers were not affected at different pH, but the catalytic performance of Au NCs-NH2 for H2O2 was significantly better than that of Au NCs-OH (Figure 7D) (Jao et al., 2010). The level of H2O2 in cells after H2DCFDA staining was quantified by flow cytometry, and the protective effect of NCs-NH2 in H2O2 treated neurons was obtained (Figures 7E,F). The fluorescence intensity of neurons pretreated with Au NCs-NH2 was significantly increased compared to the control (Graf et al., 2004). Although the catalytic activity of nanozymes is closely related to their surface properties, the mechanism of enzymelike activity of its surface coating is poorly understood. Once the nano-enzyme loses its surface accessibility, its catalytic performance will be inhibited, thus affecting its application in brain therapy and other aspects. Currently, biomedical research on gold nanoclusters without surface coating is limited due to concerns about their toxicity and stability.
Figure 7. Gold clusters for brain disease treatment. (A) Au NCs coated with dendrimers have the effect of detoxifying H2O2 to protect neurons from oxidative damage. (B) The H2O2 challenge resulted in significant cell death, with less red fluorescence than the control. (C) Cell viability of neurons pretreated with AuNCs-NH2 under H2O2. (D) The catalytic activities of G4NH2, Au NCs-OH and Au NCs-NH2 on H2O2 were compared at pH 4.8 and 7.4, respectively. (E) Changes in DCF fluorescence intensity in neurons pretreated with Au NCs-NH2. (F) Confocal fluorescence images of neuronal cells treated with Au NCs-NH2 for 24 h [reproduced from Liu et al. (2016) with permission from The Small].
In this article, we attempt to give a comprehensive overview based on gold nanoclusters. We have summarized the characteristics of enzymes such as glucose oxidase, peroxidase, catalase, superoxide dismutase, etc. The applications of gold nanoclusters in ion detection, tumor treatment and brain treatment were analyzed. This enzymatic property of gold clusters is derived from the functional groups present on the gold itself or on the surrounding monolayer. Although the development of gold clusters catalysts has made encouraging progress, the overall performance of these artificial catalytic systems is often not comparable to natural catalysts. To this end, the following aspects are awaiting implementation:
1. In recent years, with the development of nanotechnology, we can control the size and shape of nanoparticles by including hydrogen reduction, self-assembly, porous support matrix and surfactant assisted methods to adjust their catalytic activity. Because of the size dependence of gold nanomaterials, this allows us to conduct directional manipulation of surface properties. Because the surface charge and other parameters have great influence on the cell adhesion behavior, the preparation of gold nanoclusters enzyme with atomic precision is a promising method to affect the biological activity. In addition, catalysts with atomic precision are more conducive to revealing the relationship between electronic structure and properties. At the same time, the sources of catalytic activity of functional groups, and the future work may continue to focus on changes in functional groups present at catalytic sites, resulting in highly active catalysts. More importantly, we hope to open up new strategies to significantly improve catalytic performance and biosafety through the regulation of three changes in atomic accuracy, catalytic sites and functional groups.
2. Although rapid progress has been made in the development of nanomaterials with enzyme mimicking activity, it is unclear how widely these materials are used in vivo and clinically. So far, it is uncertain whether gold nanoclusters with enzyme-like activity can significantly replace many naturally occurring enzymes. All endogenous enzymes in our bodies are as a whole system and work interdependently. Any artificial substitutes, including nanoclusters, may not be suitable for the system and may cause serious side effects. During the expression of enzyme-like functions, nanoclusters can generate free radicals, causing toxicological effects. Therefore, it is worthy of further study to make full use of the beneficial effect of the enzyme-like activity of the nanoclusters and explore their application in vivo.
3. Although many researchers have demonstrated the therapeutic effects of nanozymes systems through cell and animal experiments, it is still difficult to explain the mechanism of nanozymes systems in vivo through these biological experiments. For example, quantifying reaction kinetics in vivo is not an easy task, although the available evidence supports the ultimate therapeutic outcome of these nanozymes. We can only know the results, but not the processes in the body. In recent years, multifunctional optical probes have been widely developed to detect the concentration and distribution of specific biochemical substances in the biological environment. Therefore, we may need to develop multifunctional nanometer enzyme probes, such as using the luminescence of gold clusters for imaging or sensing (Such as detecting markers of neurological disease), and then combining its enzyme-like properties for treatment, which can achieve the purpose of combining diagnosis with treatment, providing powerful help for the study of the distribution and mechanism of action of nanomaterials in living organisms. These diagnostic reagents are expected to help accurately characterize the catalytic process in vivo, and ultimately comprehensively clarify the relationship between the composition, structure, and in vivo properties of nanozymes drugs.
SL wrote the first draft. HL and X-DZ modified the manuscript content and format. YZ and WL modified the syntax.
This work was financially supported by the National Natural Science Foundation of China (Grant No. 91859101, 81971744, U1932107, 81673106, and 81471786), CAMS Innovation Fund for Medical Science (2017-12M-1-012) and National Natural Science Foundation of Tianjin (No. 19JCZDJC34000), the Innovation Foundation of Tianjin University.
The authors declare that the research was conducted in the absence of any commercial or financial relationships that could be construed as a potential conflict of interest.
Arnold, F. H., Wintrode, P. L., Miyazaki, K., and Gershenson, A. (2001). How enzymes adapt: lessons from directed evolution. Trends Biochem. Sci. 26, 100–106. doi: 10.1016/S0968-0004(00)01755-2
Bankar, S. B., Bule, M. V., Singhal, R. S., and Ananthanarayan, L. (2009). Glucose oxidase–an overview. Biotechnol. Adv. 27, 489–501. doi: 10.1016/j.biotechadv.2009.04.003
Barzegar, A., and Moosavi-Movahedi, A. A. (2011). Intracellular ROS protection efficiency and free radical-scavenging activity of curcumin. PLoS ONE 6:26012. doi: 10.1371/journal.pone.0026012
Bhan, A., and Sarkar, N. (2005). Mercury in the environment: effect on health and reproduction. Rev. Environ. Health. 20, 39–56. doi: 10.1515/REVEH.2005.20.1.39
Brodersen, S. H., Grønbjerg, U., Hvolbæk, B., and Schiøtz, J. (2011). Understanding the catalytic activity of gold nanoparticles through multi-scale simulations. J. Catal. 284, 34–41. doi: 10.1016/j.jcat.2011.08.016
Chang, H. T., Chen, P. C., Periasamy, A. P., Harroun, S. G., and Wu, W. P. (2016). Photoluminescence sensing systems based on copper, gold and silver nanomaterials. Coordin. Chem. Rev. 320, 129–138. doi: 10.1016/j.ccr.2015.12.002
Chang, T. M. S. (2013). Biomedical Applications of Immobilized Enzymes and Proteins. Quebec City, QC: Springer.
Chen, J., Huang, L., Wang, Q., Wu, W., Zhang, H., Fang, Y., et al. (2019). Bio-inspired nanozyme: a hydratase mimic in a zeolitic imidazolate framework. Nanoscale. 11, 5960–5966. doi: 10.1039/C9NR01093A
Chen, W.-Y., Lan, G.-Y., and Chang, H.-T. (2011). Use of fluorescent DNA-templated gold/silver nanoclusters for the detection of sulfide ions. Anal. chem. 83, 9450–9455. doi: 10.1021/ac202162u
Chen, Z., Xu, L., Liang, Y., and Zhao, M. (2010). pH-sensitive water-soluble nanospheric imprinted hydrogels prepared as horseradish peroxidase mimetic enzymes. Adv. Mater. 22, 1488–1492. doi: 10.1002/adma.200903122
Chi, X., Huang, D., Zhao, Z., Zhou, Z., Yin, Z., and Gao, J. (2012). Nanoprobes for in vitro diagnostics of cancer and infectious diseases. Biomaterials 33, 189–206. doi: 10.1016/j.biomaterials.2011.09.032
Comotti, M., Pina, C. D., Matarrese, R., and Rossi, M. (2010). The catalytic activity of “naked” gold particles. Angew. Chem. Int. Edit. 116, 5936–5939. doi: 10.1002/ange.200460446
Corma, A., Concepción, P., Boronat, M., Sabater, M. J., Navas, J., and Yacaman, MJ. (2013). Exceptional oxidation activity with size-controlled supported gold clusters of low atomicity. Nat. Chem. 5, 775–81. doi: 10.1038/nchem.1721
Del Valle, E. M. (2004). Cyclodextrins and their uses: a review. Process Biochem. 39, 1033–1046. doi: 10.1016/S0032-9592(03)00258-9
Ding, N., Zhao, H., Peng, W., He, Y., Zhou, Y., Yuan, L., et al. (2012). A simple colorimetric sensor based on anti-aggregation of gold nanoparticles for Hg2+ detection. Colloid. Surface. A. 395, 161–167. doi: 10.1016/j.colsurfa.2011.12.024
Ding, Y., Zhao, J., Li, B., Zhao, X., Wang, C., Guo, M., et al. (2018). The CoOOH-TMB oxidative system for use in colorimetric and test strip based determination of ascorbic acid. Microchim. Acta. 185:131. doi: 10.1007/s00604-018-2675-z
Ercal, N., Gurer-Orhan, H., and Aykin-Burns, N. (2001). Toxic metals and oxidative stress part I: mechanisms involved in metal-induced oxidative damage. Curr. Top. Med. Chem. 1, 529–539. doi: 10.2174/1568026013394831
Erel, O. (2004). A novel automated direct measurement method for total antioxidant capacity using a new generation, more stable ABTS radical cation. Clin. Biochem. 37, 277–285. doi: 10.1016/j.clinbiochem.2003.11.015
Fan, K., Xi, J., Fan, L., Wang, P., Zhu, C., Tang, Y., et al. (2018). In vivo guiding nitrogen-doped carbon nanozyme for tumor catalytic therapy. Nat. Commun. 9:1440. doi: 10.1038/s41467-018-03903-8
Feng, J., Huang, P., Shi, S., Deng, K.-Y., and Wu, F.-Y. (2017). Colorimetric detection of glutathione in cells based on peroxidase-like activity of gold nanoclusters: a promising powerful tool for identifying cancer cells. Anal. Chim. Acta. 967, 64–69. doi: 10.1016/j.aca.2017.02.025
Gallardo, C., Monrás, J., Plaza, D., Collao, B., Saona, L., Durán-Toro, V., et al. (2014). Low-temperature biosynthesis of fluorescent semiconductor nanoparticles (CdS) by oxidative stress resistant Antarctic bacteria. J. Biotechnol. 187, 108–115. doi: 10.1016/j.jbiotec.2014.07.017
Gao, L., Zhuang, J., Nie, L., Zhang, J., Zhang, Y., Gu, N., et al. (2007). Intrinsic peroxidase-like activity of ferromagnetic nanoparticles. Nat. Nanotechnol. 2, 577–83. doi: 10.1038/nnano.2007.260
Geim, A. K., and Grigorieva, I. V. (2013). Van der Waals heterostructures. Nature 499, 419–425. doi: 10.1038/nature12385
Gibson, Q. H., Swoboda, B. E., and Massey, V. (1964). Kinetics and mechanism of action of glucose oxidase. J. Biol. Chem. 239, 3927–3934.
Gleiter, R., and Hopf, H. (2006). Modern Cyclophane Chemistry. Heidelberg; Braunschweig: John Wiley Sons.
Glorieux, C., and Calderon, P. B. (2017). Catalase, a remarkable enzyme: targeting the oldest antioxidant enzyme to find a new cancer treatment approach. Biol. Chem. 398, 1095–1108. doi: 10.1515/hsz-2017-0131
Góth, L., Rass, P., and Páy, A. (2004). Catalase enzyme mutations and their association with diseases. Mol. Diagn. 8, 141–149. doi: 10.1007/BF03260057
Graf, E. R., Zhang, X. Z., Jin, S. X., Linhoff, M. W., and Craig, A. M. (2004). Neurexins induce differentiation of GABA and glutamate postsynaptic specializations via neuroligins. Cell, 119, 1013–1026. doi: 10.1016/j.cell.2004.11.035
Griffith, L. G., and Naughton, G. (2002). Tissue engineering–current challenges and expanding opportunities. Science 295, 1009–1014. doi: 10.1126/science.1069210
He, W., Wamer, W. G., Hu, X., and Wu, X. (2013). Intrinsic catalytic activity of Au nanoparticles with respect to hydrogen peroxide decomposition and superoxide scavenging. Biomaterials 34, 765–773. doi: 10.1016/j.biomaterials.2012.10.010
He, W., Zhou, Y.-T., Wamer, W. G., Boudreau, M. D., and Yin, J.-J. (2012). Mechanisms of the pH dependent generation of hydroxyl radicals and oxygen induced by Ag nanoparticles. Biomaterials 33, 7547–7555. doi: 10.1016/j.biomaterials.2012.06.076
He, X., Gao, J., Gambhir, S. S., and Cheng, Z. (2010). Near-infrared fluorescent nanoprobes for cancer molecular imaging: status and challenges. Trends Mol. Med. 16, 574–583. doi: 10.1016/j.molmed.2010.08.006
Hu, D., Sheng, Z., Fang, S., Wang, Y., Gao, D., Zhang, P., et al. (2014). Folate receptor-targeting gold nanoclusters as fluorescence enzyme mimetic nanoprobes for tumor molecular colocalization diagnosis. Theranostics 4, 142–153. doi: 10.7150/thno.7266
Huang, H., Starodub, O., McIntosh, A., Atshaves, B. P., Woldegiorgis, G., Kier, A. B., et al. (2004). Liver fatty acid-binding protein colocalizes with peroxisome proliferator activated receptor α and enhances ligand distribution to nuclei of living cells. Biochemistry 43, 2484–2500. doi: 10.1021/bi0352318
Huang, L., Chen, J., Gan, L., Wang, J., and Dong, S. (2019a). Single-atom nanozymes. Sci. Adv. 5:eaa5490. doi: 10.1126/sciadv.aav5490
Huang, Y., Ren, J., and Qu, X. (2019b). Nanozymes: classification, catalytic mechanisms, activity regulation, and, applications. Chem. Rev. 119, 4357–4412. doi: 10.1021/acs.chemrev.8b00672
Ishida, T., Kinoshita, N., Okatsu, H., Akita, T., Takei, T., and Haruta, M. (2008). Influence of the support and the size of gold clusters on catalytic activity for glucose oxidation. Angew. Chem. 47, 9265–9268. doi: 10.1002/anie.200802845
Jao, Y.-C., Chen, M.-K., and Lin, S.-Y. (2010). Enhanced quantum yield of dendrimer-entrapped gold nanodots by a specific ion-pair association and microwave irradiation for bioimaging. Chem. Commun. 46, 2626–2628. doi: 10.1039/b926364k
Jiang, D., Ni, D., Rosenkrans, Z. T., Huang, P., Yan, X., and Cai, W. (2019). Nanozyme: new horizons for responsive biomedical applications. Chem. Soc. Rev. 48, 3683–3704. doi: 10.1039/C8CS00718G
Jin, R. (2010). Quantum sized, thiolate-protected gold nanoclusters. Nanoscale 2, 343–362. doi: 10.1039/B9NR00160C
Kim, Y., Johnson, R. C., and Hupp, J. T. (2001). Gold nanoparticle-based sensing of “spectroscopically silent” heavy metal ions. Nano Lett. 1, 165–167. doi: 10.1021/nl0100116
Lequin, R. M. (2005). Enzyme immunoassay (EIA)/enzyme-linked immunosorbent assay (ELISA). Clin. Chem. 51, 2415–2418. doi: 10.1373/clinchem.2005.051532
Li, J., Liu, W., Wu, X., and Gao, X. (2015). Mechanism of pH-switchable peroxidase and catalase-like activities of gold, silver, platinum and palladium. Biomaterials 48, 37–44. doi: 10.1016/j.biomaterials.2015.01.012
Li, T., Li, F., Xiang, W., Yi, Y., Chen, Y., Cheng, L., et al. (2016). Selenium-containing amphiphiles reduced and stabilized gold nanoparticles: kill cancer cells via reactive oxygen species. ACS Appl. Mater. Inter. 8, 22106–22112. doi: 10.1021/acsami.6b08282
Li, W., and Xiaogang, Q. (2015). Cancer biomarker detection: recent achievements and challenges. Chem. Soc. Rev. 44, 2963–2997. doi: 10.1039/C4CS00370E
Liang, M., and Yan, X. (2019). Nanozymes: from new concepts, mechanisms, and standards to applications. Acc. Chem. Res. 52, 2190–2200. doi: 10.1021/acs.accounts.9b00140
Liao, H., Liu, G., Liu, Y., Li, R., Fu, W., and Hu, L. (2017). Aggregation-induced accelerating peroxidase-like activity of gold nanoclusters and their applications for colorimetric Pb2+ detection. Chem. Commun. 53, 10160–10163. doi: 10.1039/C7CC05409B
Lin, Y., Ren, J., and Qu, X. (2014a). Nano-gold as artificial enzymes: hidden talents. Adv. Mater. 26, 4200–4217. doi: 10.1002/adma.201400238
Lin, Y., Ren, J., and Qu, X. (2014b). Catalytically active nanomaterials: a promising candidate for artificial enzymes. Accounts Chem. Res. 47, 1097–1105. doi: 10.1021/ar400250z
Liu, C. P., Wu, T. H., Lin, Y. L., Liu, C. Y., Wang, S., and Lin, S. Y. (2016). Tailoring enzyme-like activities of gold nanoclusters by polymeric tertiary amines for protecting neurons against oxidative stress. Small 12, 4127–4135. doi: 10.1002/smll.201503919
Liu, C. P., Wu, T. H., Liu, C. Y., Chen, K. C., Chen, Y. X., Chen, G. S., et al. (2017). Self-supplying O2 through the catalase-like activity of gold nanoclusters for photodynamic therapy against hypoxic cancer cells. Small 13:1700278. doi: 10.1002/smll.201700278
Liu, H., Hong, G., Luo, Z., Chen, J., Chang, J., Gong, M., et al. (2019). Atomic-precision gold clusters for NIR-II imaging. Adv. Mater. 31:1901015. doi: 10.1002/adma.201901015
Liu, J., Wang, Z., Yan, X., and Jian, P. (2017). Metallic cobalt nanoparticles imbedded into ordered mesoporous carbon: a non-precious metal catalyst with excellent hydrogenation performance. J. Colloid Interf. Sci. 505, 789–795. doi: 10.1016/j.jcis.2017.06.081
Liu, Q., Yang, Y., Lv, X., Ding, Y., Zhang, Y., Jing, J., et al. (2017). One-step synthesis of uniform nanoparticles of porphyrin functionalized ceria with promising peroxidase mimetics for H2O2 and glucose colorimetric detection. Sensor. Actuat B-Chem. 240, 726–734. doi: 10.1016/j.snb.2016.09.049
Liu, S., Lu, F., Xing, R., and Zhu, J. J. (2011). Structural effects of Fe3O4 nanocrystals on peroxidase-like activity. Chem. Eur. J. 17, 620–625. doi: 10.1002/chem.201001789
Liu, Y., Ai, K., Cheng, X., Huo, L., and Lu, L. (2010). Gold-nanocluster-based fluorescent sensors for highly sensitive and selective detection of cyanide in water. Adv. Funct. Mater. 20, 951–956. doi: 10.1002/adfm.200902062
Liu, Y., Ding, D., Zhen, Y., and Guo, R. (2017). Amino acid-mediated ‘turn-off/turn-on' nanozyme activity of gold nanoclusters for sensitive and selective detection of copper ions and histidine. Biosens. Bioelectron. 92, 140–146. doi: 10.1016/j.bios.2017.01.036
Lopez, N., Janssens, T., Clausen, B., Xu, Y., Mavrikakis, M., Bligaard, T., et al. (2004). On the origin of the catalytic activity of gold nanoparticles for low-temperature CO oxidation. J. Catal. 223, 232–235. doi: 10.1016/j.jcat.2004.01.001
Luo, W., Zhu, C., Su, S., Li, D., He, Y., Huang, Q., et al. (2010). Self-catalyzed, self-limiting growth of glucose oxidase-mimicking gold nanoparticles. ACS Nano. 4, 7451–7458. doi: 10.1021/nn102592h
Martin, S., and Griswold, W. (2018). Human health effects of heavy metals. Internation. J. 15, 1–6. doi: 10.14445/23939133/IJAC-V5I1P102
Mavrikakis, M., Stoltze, P., and Nørskov, J. K. (2000). Making gold less noble. Catal. Lett. 64, 101–106. doi: 10.1023/A:1019028229377
Meeuwissen, J., and Reek, J. N. (2010). Supramolecular catalysis beyond enzyme mimics. Nat. Chem. 2, 615–21. doi: 10.1038/nchem.744
Miller, J., Kropf, A., Zha, Y., Regalbuto, J., Delannoy, L., Louis, C., et al. (2006). The effect of gold particle size on AuAu bond length and reactivity toward oxygen in supported catalysts. J. Catal. 240, 222–234. doi: 10.1016/j.jcat.2006.04.004
Molina, L., and Hammer, B. (2005). Some recent theoretical advances in the understanding of the catalytic activity of Au. Appl. Catal. A-Gen. 291, 21–31. doi: 10.1016/j.apcata.2005.01.050
Mu, X., He, H., Wang, J., Long, W., Li, Q., Liu, H., et al. (2019a). Carbogenic nanozyme with ultrahigh reactive nitrogen species selectivity for traumatic brain injury. Nano Lett. 19, 4527–4534. doi: 10.1021/acs.nanolett.9b01333
Mu, X., Wang, J., Li, Y., Xu, F., and Long, W. (2019b). Redox trimetallic nanozyme with neutral environment preference for brain injury. ACS Nano. 13, 1870–1884. doi: 10.1021/acsnano.8b08045
Mukherjee, S. P., Davoren, M., and Byrne, H. J. (2010). In vitro mammalian cytotoxicological study of PAMAM dendrimers–towards quantitative structure activity relationships. Toxicol. in vitro 24, 169–177. doi: 10.1016/j.tiv.2009.09.014
Pandey, P., Singh, S. P., Arya, S. K., Gupta, V., Datta, M., Singh, S., et al. (2007). Application of thiolated gold nanoparticles for the enhancement of glucose oxidase activity. Langmuir. 23, 3333–3337. doi: 10.1021/la062901c
Panigrahi, S., Basu, S., Praharaj, S., Pande, S., Jana, S., Pal, A., et al. (2007). Synthesis and size-selective catalysis by supported gold nanoparticles: study on heterogeneous and homogeneous catalytic process. J. Phys. Chem. C. 111, 4596–4605. doi: 10.1021/jp067554u
Pasternack, R. F., Gibbs, E. J., Collings, P. J., dePaula, J. C., Turzo, L. C., and Terracina, A. (1998). A non-conventional approach to supramolecular formation dynamics. J. Am. Chem. Soc. 120, 5873–5878. doi: 10.1021/ja980186q
Pina, C. D., Falletta, E., and Rossi, M. (2011). Update on selective oxidation using gold. Chem. Soc. Rev. 41, 350–369. doi: 10.1039/C1CS15089H
Qi, Y.-X., Zhang, M., Zhu, A., and Shi, G. (2015). Terbium (iii)/gold nanocluster conjugates: the development of a novel ratiometric fluorescent probe for mercury (ii) and a paper-based visual sensor. Analyst 140, 5656–5661. doi: 10.1039/C5AN00802F
Qu, X., Sun, H., Zhou, Y., and Ren, J. (2018). Carbon nanozymes: enzymatic properties, catalytic mechanism and applications. Angew. Chem. 57, 9224–9237. doi: 10.1002/anie.201712469
Quan, H., Zuo, C., Li, T., Liu, Y., Li, M., Zhong, M., et al. (2015). Electrochemical detection of carcinoembryonic antigen based on silver nanocluster/horseradish peroxidase nanocomposite as signal probe. Electrochim. Acta. 176, 893–897. doi: 10.1016/j.electacta.2015.07.086
Roduner, E. (2006). Size matters: why nanomaterials are different. Chem. Soc. Rev. 35, 583–592. doi: 10.1039/b502142c
Schaller, B., and Graf, R. (2004). Cerebral is chemia and reperfusion: the pathophysiologic concept as a basis for clinical therapy. J. Cerebr. Blood. F. Met. 24, 351–371. doi: 10.1097/00004647-200404000-00001
Scopes, R. K. (2013). Protein Purification: Principles and Practice. LaTrobe, VIC: Spring Science Business Media.
Senanayake, S. D., Stacchiola, D., and Rodriguez, J. A. (2013). Unique properties of ceria nanoparticles supported on metals: novel inverse ceria/copper catalysts for CO oxidation and the water-gas shift reaction. Accounts. Chem. Res. 46, 1702–1711. doi: 10.1021/ar300231p
Sheldon, R. A. (2007). Enzyme immobilization: the quest for optimum performance. Adv. Synth. Catal. 349, 1289–1307. doi: 10.1002/adsc.200700082
Shojaeifard, Z., Hemmateenejad, B., and Shamsipur, M. (2016). Efficient on–off ratiometric fluorescence probe for cyanide ion based on perturbation of the interaction between gold nanoclusters and a copper (II)-phthalocyanine complex. ACS Appl. Mater. Inter. 8, 15177–15186. doi: 10.1021/acsami.6b01566
Song, Y., Qu, K., Zhao, C., Ren, J., and Qu, X. (2010). Graphene oxide: intrinsic peroxidase catalytic activity and its application to glucose detection. Adv. Mater. 22, 2206–2210. doi: 10.1002/adma.200903783
Spichiger-Keller, U. E. (2008). Chemical Sensors and Biosensors for Medical and Biological Applications. New York, NY: John Wiley and Sons.
Tsipis, A. C., and Tsipis, C. A. (2003). Hydrometal analogues of aromatic hydrocarbons: a new class of cyclic hydrocoppers (I). J. Am. Chem. Soc. 125, 1136–1137. doi: 10.1021/ja028786j
Tsunoyama, H., Sakurai, H., and Tsukuda, T. (2006). Size effect on the catalysis of gold clusters dispersed in water for aerobic oxidation of alcohol. Chem. Phys. Lett. 429, 528–532. doi: 10.1016/j.cplett.2006.08.066
Velizarov, S., Crespo, J. G., and Reis, M. A. (2004). Removal of inorganic anions from drinking water supplies by membrane bio/processes. Rev. Environ. Sci. Bio. 3, 361–380. doi: 10.1007/s11157-004-4627-9
Wan, Y., Yang, H., and Zhao, D. (2006). “Host– Guest” chemistry in the synthesis of ordered non-siliceous mesoporous materials. Accounts Chem. Rev. 39, 423–432. doi: 10.1021/ar050091a
Wang, G.-L., Jin, L.-Y., Dong, Y.-M., Wu, X.-M., and Li, Z.-J. (2015). Intrinsic enzyme mimicking activity of gold nanoclusters upon visible light triggering and its application for colorimetric trypsin detection. Biosens. Bioelectron. 64, 523–529. doi: 10.1016/j.bios.2014.09.071
Wang, X., Wu, P., Lv, Y., and Hou, X. (2011). Ultrasensitive fluorescence detection of glutaraldehyde in water samples with bovine serum albumin-Au nanoclusters. Microchem. J. 99, 327–331. doi: 10.1016/j.microc.2011.06.004
Wang, X., Zhang, Y., Li, T., Tian, W., Zhang, Q., and Cheng, Y. (2013). Generation 9 polyamidoamine dendrimer encapsulated platinum nanoparticle mimics catalase size, shape, and catalytic activity. Langmuir 29, 5262–5270. doi: 10.1021/la3046077
Wei, H., and Wang, E. (2013). Nanomaterials with enzyme-like characteristics (nanozymes): next-generation artificial enzymes. Chem. Soc. Rev. 42, 6060–6093. doi: 10.1039/c3cs35486e
Wen, F., Dong, Y., Feng, L., Wang, S., and Zhang, S. (2011). Horseradish peroxidase functionalized fluorescent gold nanoclusters for hydrogen peroxide sensing. Anal. Chem. 83, 1193–1196. doi: 10.1021/ac1031447
Wilhelmová, N. (1996). Cornish-bowden, a.: fundamentals of enzyme kinetics. Biol. Planrraum. 38, 430–430. doi: 10.1007/BF02896674
Wilson, R., and Turner, A.P.F. (1992). Glucose oxidase: an ideal enzyme. Biosens. Bioelectron. 7, 165–185. doi: 10.1016/0956-5663(92)87013-F
Wu, J., Wang, X., Wang, Q., Lou, Z., Li, S., Zhu, Y., et al. (2019). Nanomaterials with enzyme-like characteristics (nanozymes): next-generation artificial enzymes (II). Chem. Soc. Rev. 48, 1004–1076. doi: 10.1039/C8CS00457A
Xia, X., Long, Y., and Wang, J. (2013). Glucose oxidase-functionalized fluorescent gold nanoclusters as probes for glucose. Anal. Chim. Acta. 772, 81–86. doi: 10.1016/j.aca.2013.02.025
Xiong, X., Tang, Y., Zhang, L., and Zhao, S. (2015). A label-free fluorescent assay for free chlorine in drinking water based on protein-stabilized gold nanoclusters. Talanta 132, 790–795. doi: 10.1016/j.talanta.2014.10.022
Xu, B., Wang, H., Wang, W., Gao, L., Li, S., Pan, X., et al. (2019). A single-atom nanozyme for wound disinfection applications. Angew. Chem. Int. Edit. 58, 4911–4916. doi: 10.1002/anie.201813994
Xu, X., Li, Y.-F., Zhao, J., Li, Y., Lin, J., Li, B., et al. (2015). Nanomaterial-based approaches for the detection and speciation of mercury. Analyst. 140, 7841–7853. doi: 10.1039/C5AN01519G
Yan, R., Sun, S., Yang, J., Long, W., Wang, J., Mu, X., et al. (2019). A nanozyme-based bandage with single-atom catalysis for brain trauma. ACS Nano. 13, 11552–11560. doi: 10.1021/acsnano.9b05075
Yang, B., Li, J., Deng, H., and Zhang, L. (2016). Progress of mimetic enzymes and their applications in chemical sensors. Crit. Rev. Anal. Chem. 46, 469–481. doi: 10.1080/10408347.2016.1151767
Yang, X., Wang, J., Su, D., Xia, Q., Chai, F., Wang, C., et al. (2014). Fluorescent detection of TNT and 4-nitrophenol by BSA Au nanoclusters. Dalton T 43, 10057–10063. doi: 10.1039/C4DT00490F
Yin, Y., and Talapin, D. (2013). The chemistry of functional nanomaterials. Chem. Soc. Rev. 42, 2484–2487. doi: 10.1039/c3cs90011h
Yuwen, L., Xu, F., Xue, B., Luo, Z., Zhang, Q., Bao, B., et al. (2014). General synthesis of noble metal (Au, Ag, Pd, Pt) nanocrystal modified MoS2 nanosheets and the enhanced catalytic activity of Pd–MoS2 for methanol oxidation. Nanoscale 6, 5762–5769. doi: 10.1039/C3NR06084E
Zhang, Y., Wang, F., Liu, C., Wang, Z., Kang, L., Huang, Y., et al. (2018). Nanozyme decorated metal–organic frameworks for enhanced photodynamic therapy. ACS Nano. 12, 651–661. doi: 10.1021/acsnano.7b07746
Zhou, X., Xu, W., Liu, G., Panda, D., and Chen, P. (2010). Size-dependent catalytic activity and dynamics of gold nanoparticles at the single-molecule level. J. Am. Chem. Soc. 132, 138–146. doi: 10.1021/ja904307n
Zhou, Y., Liu, B., Yang, R., and Liu, J. (2017). Filling in the gaps between nanozymes and enzymes: challenges and opportunities. Bioconjugate Chem. 28, 2903–2909. doi: 10.1021/acs.bioconjchem.7b00673
Keywords: gold nanoclusters, nanozyme, enzyme-like properties, cancer therapy, bio-detection
Citation: Zhang Y, Li S, Liu H, Long W and Zhang X-D (2020) Enzyme-Like Properties of Gold Clusters for Biomedical Application. Front. Chem. 8:219. doi: 10.3389/fchem.2020.00219
Received: 03 January 2020; Accepted: 09 March 2020;
Published: 03 April 2020.
Edited by:
Kelong Fan, Institute of Biophysics (CAS), ChinaReviewed by:
Rong Yang, National Center for Nanoscience and Technology (CAS), ChinaCopyright © 2020 Zhang, Li, Liu, Long and Zhang. This is an open-access article distributed under the terms of the Creative Commons Attribution License (CC BY). The use, distribution or reproduction in other forums is permitted, provided the original author(s) and the copyright owner(s) are credited and that the original publication in this journal is cited, in accordance with accepted academic practice. No use, distribution or reproduction is permitted which does not comply with these terms.
*Correspondence: Wei Long, bG9uZ3dheUBpcm0tY2Ftcy5hYy5jbg==; Xiao-Dong Zhang, eGlhb2Rvbmd6aGFuZ0B0anUuZWR1LmNu
Disclaimer: All claims expressed in this article are solely those of the authors and do not necessarily represent those of their affiliated organizations, or those of the publisher, the editors and the reviewers. Any product that may be evaluated in this article or claim that may be made by its manufacturer is not guaranteed or endorsed by the publisher.
Research integrity at Frontiers
Learn more about the work of our research integrity team to safeguard the quality of each article we publish.