- 1Department of Chemistry, Wichita State University, Wichita, KS, United States
- 2Department of Chemistry, Iowa State University, Ames, IA, United States
- 3Ames Laboratory, U.S. Department of Energy, Ames, IA, United States
Flux crystal growth has been widely applied to explore new phases and grow crystals of emerging materials. To accommodate the needs of high-quality single crystals, the flux crystal growth should be reliable, controllable, and predictable. The selections of suitable flux and growth conditions remain empirical due to the lack of systematic investigation especially for reactions, which involve highly volatile components, such as P and As. Considering the flux elements, often the system in question is a quaternary or a higher multinary system, which drastically increases complexity. In this manuscript, on the examples of flux growth of phosphides and arsenides, guidelines of flux selections, existing challenges, and future directions are discussed. We expect that the field will be further developed by applying in situ techniques and computational modeling of the nucleation and growth kinetics. Additionally, leveraging variables other than temperature, such as applied pressure, will make flux growth a more powerful tool in the future.
Introduction
The fundamental research goals of solid state chemistry, materials science, and condensed matter physics are to establish correlations between crystal structure and physical properties (Pamplin, 1980; Kanatzidis et al., 2005; The National Academies of Sciences Engineering Medicine, 2009). The single crystalline solids provide a suitable and simple platform due to the absence of grain boundaries and suppression and/or control of defects. Large, mm-sized or even bigger single crystals are indispensable for the characterization of anisotropic physical properties, such as magnetic, heat and charge transport, or optical properties (Phelan et al., 2012; Babu et al., 2018; Liu et al., 2018; Canfield, 2020). The scientific community has raised high standards for single crystal growth. Growth of single crystals should be fast, controllable, and capable of handling complex systems (Pamplin, 1980; Kanatzidis et al., 2005; The National Academies of Sciences Engineering Medicine, 2009; Canfield, 2020). One of the options is to grow crystals from a high-temperature molten media, flux, which is widely used for growth of different intermetallic, semiconducting, and insulating compounds of diverse chemical nature ranging from oxides and halides to metal alloys (Pamplin, 1980; Canfield and Fisk, 1991; Kanatzidis et al., 2005; Bugaris and zur Loye, 2012; Phelan et al., 2012; Juillerat et al., 2019; Canfield, 2020). The art of flux crystal growth is a combination of science and technology. The science part requires comprehensive knowledge of chemical bonding and reactivity of components and flux, combined with knowledge of the thermodynamics and kinetics of the growth processes. The technology aspect of the crystal growth is in its “trial and error” nature, which requires multiple attempts sometimes guided by the grower's intuition. In situ studies of the flux growth have provided insights regarding the structure of the liquid phase and cascades of solid–solid transformation occurring during heating and cooling (Shoemaker et al., 2014; Woo et al., 2018). Several general aspects of flux selection, together with examples and challenges, are discussed in this paper with focus on ternary and multinary phosphides and arsenides. The chemistry of phosphides and arsenides at elevated temperature is complicated because of the high vapor pressure of P or As. The volatility of P and As has resulted in poor exploration of many metal–phosphorus (arsenic) phase diagrams in the areas of high pnictogen content. Nevertheless, compounds with substantial P(As) content often exhibit interesting chemistry and useful practical properties, with potential applications as heat conductors, thermoelectrics, catalysts, and non-linear optical materials (Soheilnia et al., 2007; Lindsay et al., 2013; Dolyniuk et al., 2017; Nuss et al., 2017; Pöhls et al., 2017; Li et al., 2018; Owens-Baird et al., 2018, 2019; Woo et al., 2018; Coleman et al., 2019; Mark et al., 2019; Yu et al., 2019).
Flux: General Considerations
Various options exist for flux growth of inorganic crystals, such as metal flux, salt flux, or self-flux. Basic considerations for flux choice involve three aspects: solubility of reactants in flux, reaction path, and nucleation and growth of the target crystals (Figure 1, bottom). The presumptions about solubility of reactants in the flux are mainly drawn from the binary phase diagrams (Figure 1G). The flux crystal growth process is usually accompanied by chemical reaction, distinguishing itself from the growth of molecular compounds where recrystallization techniques prevail. In most cases, the flux plays a dual role in the crystal growth process: facilitating chemical reactions and aiding crystal growth (Figures 1H,I). In addition to the chemical and physical properties of the reactants and the products, interactions between the reactants and the flux should also be considered. The controllable nucleation and growth for a new system are challenging to achieve, due to the lack of systematic investigations. To compensate, a series of experiments are performed screening several flux/reagent ratios and heating/cooling rates. Owing to the limited size of this minireview, the comprehensive discussions of thermodynamics and kinetics are not covered here.
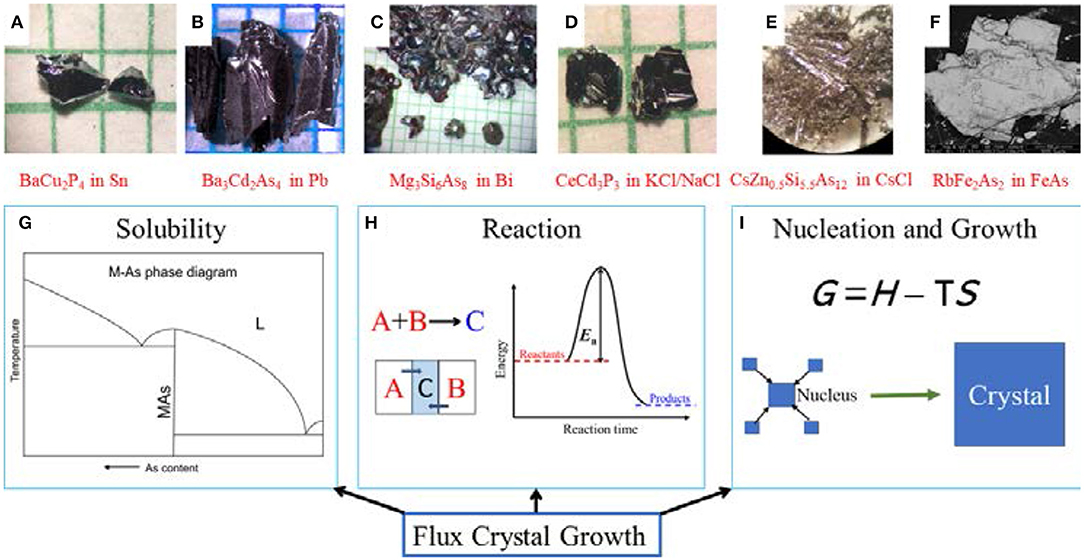
Figure 1. Top: Examples of phosphide and arsenide crystals grown in different fluxes (Wang et al., 2013; Dolyniuk et al., 2015; Woo et al., 2018, 2019a). (D,F) show unpublished crystals synthesized in Kovnir group. Bottom: main aspects of flux crystal growth. (A) BaCu2P4 in Sn, (B) Ba3Cd2As4 in Pb, (C) Mg3Si6As8 in Bi, (D) CeCd3P3 inKCl/NaCl, (E) CsZn0.5Si5.5As12 in CsCl, (F) RbFe2As2 in FeAs, (G) M-As phase diagram, (H) Solid-state reaction, (I) Nucleation and growth.
For exploring a new system, selecting a suitable flux is crucial. The following considerations of the flux properties are important:
Solubility
Moderate solubility of the target phase in the flux and a low-temperature dependence of the solubility are required. Low solubility of the reactants and the target phase will prevent chemical reactions and crystal growth. However, very high solubility will result in unsaturated solutions inhibiting the crystallization of the target phase. The temperature dependence of a target phase's solubility plays a critical role in the crystal growth process because it determines the cooling rate. For a new system or a new compound, solubility and its temperature dependence are often unknown and not easy to determine, but a few hints may be obtained by running several model experiments. Cooling rates as low as 0.4 K/h have been used, requiring weeks to complete the growth.
Inertness
The inertness of flux is required to avoid formation of stable phases between the flux and the reactants. Forming flux-containing compounds will shift the stoichiometry and disturb the nucleation process. Another aspect of flux inertness is undesirable doping of the target phase with flux components.
Melting Temperature
Large temperature ranges are ideal for crystal growth. Favorable fluxes have low melting temperatures coupled with high boiling points.
Toxicity
Non-toxic flux with low vapor pressure is safer and easier to handle for the environment and researchers. For example, Hg is rarely used as flux.
Container
To avoid incorporation of the container elements into the system and/or the target crystals, chemical reactions between flux and container are highly undesirable. If the flux has low solubility of volatile reactants (P, As) or the flux itself has a high vapor pressure, the container volume is another parameter that must be considered.
Removal
Flux should be removed after the growth is complete. Several methods including mechanical separation and polishing of crystals, high-temperature centrifugation of liquid flux, and dissolving flux at or near room temperature are frequently used. In the latter case the flux nature defines whether simple solvents (water, ethanol) or acid (HCl, H2O2/acetic acid) should be used. Dissolution requires the target phase to be stable in that media, limiting the choice of fluxes. Thus, KCl/NaCl is preferred over Sn flux for acid-sensitive materials due to the solubility of chlorides in water. Bi and Pb fluxes are not soluble in non-oxidizing acids and require the presence of hydrogen peroxide in the dissolving media.
Price
Flux is used in high excess in comparison to amounts of target materials or reactants. Thus, very expensive fluxes are always not the first choice especially with “trial and error” approaches. For example, Au/Si eutectic (Tmelting = 636 K) might be a good flux, but it is rarely used due to the high price of gold.
Viscosity, Density, and Polarity
The high viscosity of flux may result in non-stationary mass transport, which brings no benefits to crystal growth. The densities of reactants and the flux should be comparable. Analogous to mixing water and benzene in a beaker, a phase separation may occur during flux crystal growth. In water/benzene combination not only density but also polarity of the liquids plays an important role. For flux synthesis, the rule of thumb is that metallic phases grow better from metallic fluxes (Sn, Pb, Bi), while semiconducting crystals are easier to grow from semiconducting fluxes (salts). This is not a strict rule, and exceptions are known.
The bottom line is that no single flux can satisfy all the criteria. However, these guiding principles can help to make rational flux choice for synthesis.
Metal Flux Examples
Phosphorus and arsenic can form binary pnictides with almost all metals (excluding Hg and Bi) (Shatruk, 2019). Mercury has high vapor pressure and is toxic, thus bismuth should be the first choice for flux to grow phosphides and arsenides based on the inertness of flux criteria. Bi works well for arsenides due to the relatively high solubility of As in Bi. However, the solubility of P in Bi is quite low, and Sn flux is preferred for phosphides. Despite the existence of a number of binary Sn–P phases, multiple complex phosphides have been grown from Sn flux (Kanatzidis et al., 2005). Tin phosphides can be washed away with acid and have moderate melting points below 850 K. Binary tin arsenides have similar melting points to tin phosphides of ~870 K; however, Sn has somewhat limited success as a flux to grow arsenides; see example of RCo2Pn2 phases below. A common side-product of arsenide growth attempts in tin flux is SnAs binary with NaCl structure, which is different from SnP. The latter is a metastable phase with a unique crystal structure (Gullman, 1990). More systematic studies are required to figure out whether SnAs is an important intermediate, which affects crystallization processes or just a common side-product.
Pb was recently reported to form binary PbP7 (Schäfer et al., 2014), which demonstrates higher affinity of Pb to P compared to Bi, despite the exact phase diagram for the Pb–P system was not reported. Svilen Bobev et al. reported multiple successful crystal growths of both phosphides and arsenides from lead flux (Bobev et al., 2009; Saparov and Bobev, 2010; He et al., 2016). Finally, other metallic fluxes, such as Zn, Al, Ga, and In have been used for selected cases of phosphide and arsenide crystal growth.
BaCu2P4 in Sn Flux
Synthesis of twisted clathrate BaCu2P4 (Dolyniuk et al., 2015) is hampered by the existence of another clathrate with close composition, BaCu2P3.75. Thermal analysis confirmed that BaCu2P4 converts into BaCu2P3.75 upon heating prior to melting, which explains the experimental observations that high-temperature syntheses of BaCu2P4 always resulted in the formation of BaCu2P3.75. In turn, Ba and Cu starting materials are not active at low temperatures (<1,000 K) due to their high melting points, 1,003 and 1,353 K, respectively. To resolve this issue, flux growth was applied. P has high solubility in Sn (Zavrazhnov et al., 2018), which can be removed by centrifugation. The mm-sized BaCu2P4 crystals were successfully grown by Sn flux at 1,073 K (Figure 1A).
Mg3Si6As8 in Bi Flux
The Mg–Si–As ternary system has been overlooked for many years with only one theoretically predicted compound MgSiAs2 (Woo et al., 2018). Our synthetic efforts discovered three new ternary compounds, MgSiAs2, Mg3Si6As8, and Mg3Si3As8 (Woo et al., 2018; Wang et al., 2019). MgSiAs2 was confirmed to have chalcopyrite structure type, while the other two compounds crystallize in new structure types. The crystal growth of the Mg–Si–As system is challenging due to the combination of high vapor pressure of Mg and As and the inertness of Si at reaction temperatures. As discussed above, Bi is preferred over Sn flux for arsenides. Indeed, mm-sized red crystals of Mg3Si6As8 were obtained in Bi flux (Figure 1C).
Ba3Cd2As4 in Pb Flux
When exploring the synthesis of Ba3Cd2As4 (Wang et al., 2013), Cd and Pb flux were applied to grow crystals. Cd has a moderate range of operation between melting and boiling points, 594 and 1,038 K, respectively. Using Cd as a self-flux, the chances of incorporation of foreign elements were minimized. A disadvantage of Cd flux was the formation of binary admixture Cd3As2. Small crystals of Ba3Cd2As4 were grown from Cd flux with low yield. Larger crystals of Ba3Cd2As4 were grown from Pb flux. Pb has a comparable melting point and much higher boiling point than Cd, 603 and 2,023 K, respectively. The Ba, Cd, and As reactants have reasonable solubility in Pb at elevated temperatures. Mm-sized crystals of Ba3Cd2As4 were grown in Pb flux (Figure 1B).
RCo2Pn2 (R = Rare-Earth Metal; Pn = P and As) in Sn and Bi Fluxes
In the three aforementioned examples, direct reactions of elements produced the polycrystalline samples of the target phases. Often this is not the case, and flux is used to overcome high reaction barriers. For example, reactions of neat La, Co, and P resulted in a mixture of stable binary phosphides, LaP, and CoP/Co2P, which would not react with each other preventing formation of La–Co–P ternaries. Sn flux resolves these issues resulting in mm-sized crystals of RCo2P2, which allows for establishing of the structure–magnetic property relationships (Kovnir et al., 2010, 2011a,b; Kovnir et al., 2013; Thompson et al., 2014a; Tan et al., 2017). When switching to isostructural arsenides, tin flux failed to produce any RCo2As2 phases. Instead Bi flux was successfully applied (Thompson et al., 2011, 2014b; Tan et al., 2016a, 2018). Detailed structural characterizations revealed that Bi is capable of partially replacing R3+ cations, probably due to its trivalent nature (Thompson et al., 2011). No evidences of Sn incorporation into crystals of RCo2P2 were found. However, divalent Sn was reported to partially replace Ba2+ in isostructural AFe2As2 superconductors (A = Ba, K) (Mathieu and Latturner, 2009).
GeP in Sn and Bi Fluxes
Incorporation of the metal flux components into the crystal structure is one of the reasons for discarding certain fluxes. Elemental Ge and P will not react with each other at the temperatures at which binary GeP is stable, thus calling for flux application. The growth of GeP van-der-Waals semiconductor crystals were achieved from Sn flux (Lee et al., 2015). The detailed structural characterizations show that a significant degree of disorder in both Ge and P sites is introduced by partial replacement of Ge with larger Sn atoms. Sn doping resulted in drastic decrease in melting/decomposition temperature by 228 K compared to the pristine GeP. Similarly, over an order of magnitude increase in electrical resistivity was detected for Sn-doped GeP. Undoped GeP was successfully synthesized using Bi flux instead of Sn. No incorporation of Bi was detected by SEM/EDS and crystallographic investigations (Lee et al., 2015).
Salt Flux Crystal Growth Examples
Many inorganic pnictide crystals can be grown in salt fluxes. Salt fluxes are high-temperature ionic liquids (Table 1). The salt flux can be inert (NaCl/KCl) or reactive (CsCl, AuCl, ZnCl2). A huge advantage of salt flux is the simplicity of removal by solvent wash, especially by water. Owing to the lack of knowledge about elemental solubility in salts, the selecting of salt flux is an empirical process, until appropriate phase diagrams are made. An example of crystals of CeCd3P3 grown in NaCl/KCl inert salt flux is shown in Figure 1D. Reactive fluxes often can be applied to overcome inertness of a transition metal. Thus, BaT2P4 and BaT2P3.75 (T = Cu, Ni, Au) can be produced starting from TCl or TCl2 precursors and excess of Ba (Kovnir et al., 2011; Fulmer et al., 2013a,b; Dolyniuk et al., 2015). Metal chlorides serve as source of T element due to Ba + 2TCl → BaCl2 + 2T reactions. Simultaneously, the mixture of barium and transition metal chlorides serves as a flux media.
Finally, salt flux may be used to reduce the reactivity of a reactant. Metallic Cs is extremely reactive, challenging to handle, and requires a glovebox due to its pyrophoric nature. Instead, the CsCl + Mg combination can be safely handled in air. CsCl is used as a flux and a source of Cs while producing a mixture of Mg and Cs chlorides that are removed by water washing after synthesis (Figure 1E) (Woo et al., 2019a).
La2Mg3SiP6 in MgI2 Flux
As summarized in Table 1, salt flux was useful to grow compounds in La–M–P systems (M = Zn, Cd, Cu, Mg, Si). The crystal growth of La2Mg3SiP6 was impeded by the combination of inertness of La and Si and high reactivity and vapor pressures of Mg and P (Wang et al., 2017). Attempts to grow La2Mg3SiP6 using Sn or Zn metal flux were unsuccessful. Changing to KCl/NaCl flux resulted only in a mixture of Zn3P2 and SiP2. Finally, MgI2 was proven to be proper flux for crystal growth of La2Mg3SiP6. Partial decomposition of MgI2 at reaction temperatures and release of iodine, which may serve as a transport agent might play a role in promoting reactivity of binary phosphides.
Self-Flux
The crystal growth by self-flux (flux composed solely of elements present in the target crystals) has the lowest chances of incorporating foreign elements. The self-flux is usually pre-synthesized, examples are provided in Table 1. When exploring the synthesis of a ternary system ABC, all possible binary compounds, AB, AC, BC can be considered as self-flux if they meet the following criteria: facile synthesis, low melting and high boiling points, easy removal. For example, CaAFe4As4 (A = K, Rb, Cs) superconductors were initially discovered as polycrystalline samples, which only exist in a narrow temperature window (Iyo et al., 2016). Afterward, large crystals were grown from FeAs self-flux (Meier et al., 2016). A special crucible design, such as the Canfield crucibles, is very useful for separation of large crystals from flux (Canfield, 2020).
Transport Growth in Flux
Transport reactions in a liquid flux have a characteristic temperature gradient. At the hot end, reactants dissolve or form mobile species that migrate toward the cold end and crystallize in the target phase. This stands for endothermic equilibrium reaction, while for exothermic reaction, the transport happens from cold to hot end. Provided that the temperature gradient is consistent and includes the crystallization temperature of the target phase, crystal growth occurs on the cold end while simultaneously consuming reactants on the hot end, similar to the vapor transport reactions. Transport reactions do not require full solubility of reactants in the flux, allowing for a wider choice of fluxes and larger mass loadings of the starting materials, resulting in larger quantities of produced crystals. Transport reactions allow certain flexibility when selecting a flux because the solubility of reactants may not be as crucial. In turn, the viscosity and associated mass transport apply stricter constraints to the flux selection. This method is relatively new but has been demonstrated for crystal growth of antimonides, halides, chalcogenides, and transition metal chalcophosphates with MPQ3 (Q = S, Se) compositions (Yan et al., 2017). The development of transport reactions for crystal growth of phosphides and arsenides is currently underway. For liquid transport, presumably all of the fluxes previously discussed could allow crystal growth, which indicates that temperature gradients can be a useful variable when applied correctly. In a more in-depth review, Yan et al. (2017) demonstrated several examples of self-flux and salt flux used for transport growth.
Discussion and Perspective
As discussed above, a researcher has several flux-based methods to grow single crystals of desired phosphides and arsenides. There is no universal, one-size-fits-all, flux method and no guarantee that for any given phosphide or arsenide the flux growth of cm-sized crystals can be developed. More systematic studies of flux growth mechanisms are required to make the latter statement false. Since the reaction and crystal growth are performed within a sealed environment to contain vapor pressure of pnictogens and prevent oxidation by air, the observation of reaction and crystal growth processes are limited. Many assumptions based on simple binary phase diagrams have to be made, which are not always correct, and not all binary phase diagrams are fully developed. Computational modeling of liquid-to-solid transformations in selected areas of complex phase diagrams may help reduce the number of trials for crystal growth. Recent developments of in situ techniques, TEM, and elastic scattering at synchrotron and neutron sources, revealed a lot of hidden processes occurring during flux synthesis, thus allowing for more rational design of flux reactions and crystal growth (Shoemaker et al., 2014; Woo et al., 2018, 2019b; Vasquez et al., 2019). Finally, computationally cheap methods, such as machine learning (Yao et al., 2019), can be applied to build bridges between “trial and error” and fully rational approaches. Results of unsuccessful trials, which are documented only in lab notebooks are required to train machine learning models and reveal hidden relationships.
Another challenge to be addressed is an adaptive control of crystal growth. Currently, most flux crystal growth relies upon spontaneous crystallization processes by manipulating temperature, initial reactants/flux ratios, and cooling rates. A design of a new type of flux crystal growth furnace, which can balance the inert atmosphere requirement and controlled growth with adaptive feedback loop, may provide larger and better quality crystals. The last to be mentioned, flux crystal growth under extreme conditions such as high temperature–high pressure remains essentially unexplored. Sparked by the recent discovery of almost room temperature superconductivity in LaH10 superhydride, there are rich opportunities to explore new phases and reaction mechanisms under applied pressure (Geballe et al., 2018). However, the challenges due to the presence of extreme conditions and necessity to work in limited sample environments are substantial. So far, crystals of superhydrides have not been produced and studied. Maybe high-temperature and high-pressure flux can help to grow crystals of superhydrides?
Author Contributions
The manuscript was written through contributions of all authors. All authors have given approval to the final version of the manuscript.
Funding
This work was supported by the Laboratory Research and Development Program of the Ames Laboratory under the U.S. Department of Energy Contract No. DE-AC02–07CH11358.
Conflict of Interest
The authors declare that the research was conducted in the absence of any commercial or financial relationships that could be construed as a potential conflict of interest.
Acknowledgments
JW was thankful for the support from Wichita State University start-up funds. PY was thankful to Chateaubriand Fellowship Program.
References
Babu, R., Giribabu, L., and Singh, S. P. (2018). Recent advances in halide-based perovskite crystals and their optoelectronic applications. Cryst. Growth Des. 18, 2645–2664. doi: 10.1021/acs.cgd.7b01767
Bao, J.-K., Liu, J.-Y., Ma, C.-W., Meng, Z.-H., Tang, Z.-T., Sun, Y.-L., et al. (2015). Superconductivity in Quasi-One-Dimensional K2Cr3As3 with significant electron correlations. Phys. Rev. X. 5:011013. doi: 10.1103/PhysRevX.5.011013
Bobev, S., Xia, S.-Q., Bauer, E. D., Ronning, F., Thompson, J. D., and Sarrao, J. L. (2009). Nickel deficiency in RENi2−xP2 (RE = La, Ce, Pr). Combined crystallographic and physical property studies. J. Solid State Chem. 182, 1473–1480. doi: 10.1016/j.jssc.2009.03.014
Bugaris, D. E., and zur Loye, H.-C. (2012). Materials discovery by flux crystal growth: quaternary and higher order oxides. Angew. Chem. Int. Ed. 51, 3780–3811. doi: 10.1002/anie.201102676
Canfield, P. C. (2020). New materials physics. Rep. Progr. Phys. 83:016501. doi: 10.1088/1361-6633/ab514b
Canfield, P. C., and Fisk, Z. (1991). Growth of single crystals from metallic fluxes. Philos. Mag. B. 65, 1117–1123. doi: 10.1080/13642819208215073
Cheng, B., Hu, B. F., Yuan, R. H., Dong, T., Fang, A. F., Chen, Z. G., et al. (2012). Field-induced spin-flop transitions in single-crystalline CaCo2As2. Phys. Rev. B 85:144426. doi: 10.1103/PhysRevB.85.144426
Coleman, N. Jr., Lovander, M. D., Leddy, J., and Gillan, E. G. (2019). Phosphorus-rich metal phosphides: direct and tin flux-assisted synthesis and evaluation as hydrogen evolution electrocatalysts. Inorg. Chem. 58, 5013–5024. doi: 10.1021/acs.inorgchem.9b00032
Dolyniuk, J., Wang, J., Lee, K., and Kovnir, K. (2015). Twisted Kelvin cells and truncated octahedral cages in the crystal structures of unconventional clathrates, AM2P4 (A = Sr, Ba, M = Cu, Ni). Chem. Mater. 27, 4476–4484. doi: 10.1021/acs.chemmater.5b01592
Dolyniuk, J., Zaikina, J. V., Kaseman, D. C., Sen, S., and Kovnir, K. (2017). Breaking the Tetra-Coordinated Framework Rule: New Clathrate Ba8M24P28+δ (M = Cu/Zn). Angew. Chem. Int. Ed. 56, 2418–2422. doi: 10.1002/anie.201611510
Fulmer, J., Kaseman, D., Dolyniuk, J., Lee, K., Sen, S., and Kovnir, K. (2013a). BaAu2P4: Layered Zintl Polyphosphide with Infinite (P−) Chains. Inorg. Chem. 52, 7061–7067. doi: 10.1021/ic400584w
Fulmer, J., Lebedev, O. I., Roddatis, V. V., Kaseman, D., Sen, S., Dolyniuk, J., et al. (2013b). Clathrate Ba8Au16P30: The “Gold Standard” for lattice thermal conductivity. J. Amer. Chem. Soc. 135, 12313–12323. doi: 10.1021/ja4052679
Geballe, Z. M., Liu, H., Mishra, A. K., Ahart, M., Somayazulu, M., Meng, Y., et al. (2018). Synthesis and stability of lanthanum superhydrides. Angew. Chem. Int. Ed. 57, 688–692. doi: 10.1002/anie.201709970
Gullman, J. (1990). The crystal structure of SnP. J. Solid State Chem. 87, 202–207. doi: 10.1016/0022-4596(90)90083-A
He, H., Stoyko, S., and Bobev, S. (2016). New insights into the application of the valence rules in Zintl phases - Crystal and electronic structures of Ba7Ga4P9, Ba7Ga4As9, Ba7Al4Sb9, Ba6CaAl4Sb9, and Ba6CaGa4Sb9. J. Solid State Chem. 236, 116–122. doi: 10.1016/j.jssc.2015.07.015
Iyo, A., Kawashima, K., Kinjo, T., Nishio, T., Ishida, S., Fujihisa, H., et al. (2016). New-Structure-Type Fe-Based Superconductors: CaAFe4As4 (A = K, Rb, Cs) and SrAFe4As4 (A = Rb, Cs). J. Am. Chem. Soc. 138:3410. doi: 10.1021/jacs.5b12571
Jiang, J., Olmstead, M. M., Kauzlarich, S. M., Lee, H.-O., Klavins, P., and Fisk, Z. (2005). Negative magnetoresistance in a magnetic semiconducting Zintl phase: Eu3In2P4. Inorg. Chem. 44, 5322–5327. doi: 10.1021/ic0504036
Juillerat, C. A., Klepov, V. V., Morrison, G., Pace, K. A., and zur Loye, H.-C. (2019). Flux crystal growth: a versatile technique to reveal the crystal chemistry of complex uranium oxides. Dalton Trans. 48, 3162–3181. doi: 10.1039/C8DT04675A
Kanatzidis, M. G., Pöttgen, R., and Jeitschko, W. (2005). The Metal Flux: a preparative tool for the exploration of intermetallic compounds. Angew. Chem. Int. Ed. 44, 6996–7023. doi: 10.1002/anie.200462170
Kovnir, K., Garlea, V. O., Thompson, C. M., Zhou, H. D., Reiff, W. M., Ozarowski, A., et al. (2011b). Spin-glass behavior in LaFexCo2−xP2 solid solutions: interplay between magnetic properties and crystal and electronic structures. Inorg. Chem. 50, 10274–10283. doi: 10.1021/ic201328y
Kovnir, K., Reiff, W. M., Menushenkov, A. P., Yaroslavtsev, A. A., Chernikov, R. V., and Shatruk, M. (2011a). Chemical metamagnetism": from antiferromagnetic PrCo2P2 to ferromagnetic Pr0.8Eu0.2Co2P2 via chemical compression. Chem. Mater. 23, 3021–3024. doi: 10.1021/cm200782z
Kovnir, K., Stockert, U., Budnyk, S., Prots, Y., Baitinger, M., Paschen, S., et al. (2011). Introducing a magnetic guest to a tetrel-free clathrate: synthesis, structure, and properties of EuxBa8−xCu16P30 (0 ≤ x ≤ 1.5). Inorg. Chem. 50, 10387–10396. doi: 10.1021/ic201474h
Kovnir, K., Thompson, C. M., Garlea, V. O., Haskel, D., Polyanskii, A. A., Zhou, H., et al. (2013). Modification of magnetic anisotropy through 3d-4f coupling in La0.75Pr0.25Co2P2. Phys. Rev. B. 88:104429. doi: 10.1103/PhysRevB.88.104429
Kovnir, K., Thompson, C. M., Zhou, H. D., Wiebe, C. R., and Shatruk, M. (2010). Tuning ferro- and metamagnetic transitions in rare-earth cobalt phosphides La1−xPrxCo2P2. Chem. Mater. 22, 1704–1713. doi: 10.1021/cm903497h
Lee, K., Synnestvedt, S., Bellard, M., and Kovnir, K. (2015). GeP and (Ge1−xSnx)(P1−yGey) (x = 0.12, y = 0.05): Synthesis, structure, and properties of two-dimensional layered tetrel phosphides. J. Solid State Chem. 224, 62–70. doi: 10.1016/j.jssc.2014.04.021
Li, S., Zheng, Q., Lv, Y., Liu, X., Wang, X., Huang, P. Y., et al. (2018). High thermal conductivity in cubic boron arsenide crystals. Science 361, 579–581. doi: 10.1126/science.aat8982
Lindsay, L., Broido, D. A., and Reinecke, T. L. (2013). First-principles determination of ultrahigh thermal conductivity of boron arsenide: a competitor for diamond? Phys. Rev. Lett. 111:025901. doi: 10.1103/PhysRevLett.111.025901
Liu, S., Joe, G., Zhao, H., Zhou, Y., Orvis, T., Huyan, H., et al. (2018). Giant optical anisotropy in a quasi-one-dimensional crystal. Nat. Photon. 12, 392–396. doi: 10.1038/s41566-018-0189-1
Mark, J., Wang, J., Wu, K., Lo, J. G., Lee, S., and Kovnir, K. (2019). Ba2Si3P6: 1D nonlinear optical material with thermal barrier chains. J. Amer. Chem. Soc. 141, 11976–11983. doi: 10.1021/jacs.9b04653
Mathieu, J. L., and Latturner, S. E. (2009). Zintl phase as dopant source in the flux synthesis of Ba1−xKxFe2As2 type superconductors. Chem. Commun. 33, 4965–4967. doi: 10.1039/b910749e
Meier, W. R., Kong, T., Kaluarachchi, U. S., Taufour, V., Jo, N. H., Drachuck, G., et al. (2016). Anisotropic thermodynamic and transport properties of single crystalline CaKFe4As4. Phys. Rev. B 94:064501. doi: 10.1103/PhysRevB.94.064501
Nuss, J., Wedig, U., Xie, W., Yordanov, P., Bruin, J., Hübner, R., et al. (2017). Phosphide–tetrahedrite Ag6Ge10P12: thermoelectric performance of a long-forgotten silver-cluster compound. Chem. Mater. 29, 6956–6965. doi: 10.1021/acs.chemmater.7b02474
Owens-Baird, B., Kolen'ko, Y. V., and Kovnir, K. (2018). Structure-activity relationships for Pt-free metal phosphide hydrogen evolution electrocatalysts. Chem. Europ. J. 24, 7298–7311. doi: 10.1002/chem.201705322
Owens-Baird, B., Xu, J., Petrovykh, D. Y., Bondarchuk, O., Ziouani, Y., Gonzalez-Ballesteros, N., et al. (2019). NiP2: a story of two divergent polymorphic multifunctional materials. Chem. Mater. 31, 3407–3418. doi: 10.1021/acs.chemmater.9b00565
Ozawa, T. C., and Kauzlarich, S. M. (2003). (NaCl)/(KCl) flux single crystal growth and crystal structure of the new quaternary mixed-metal pnictide: BaCuZn3As3. Inorg. Chem. 42, 3183–3186. doi: 10.1021/ic034061k
Phelan, W. A., Menard, M. C., Kangas, M. J., McCandless, G. T., Drake, B. L., and Chan, J. Y. (2012). Adventures in crystal growth: synthesis and characterization of single crystals of complex intermetallic compounds. Chem. Mater. 24, 409–420. doi: 10.1021/cm2019873
Pöhls, J.-H., Faghaninia, A., Petretto, G., Aydemir, U., Ricci, F., Li, G., et al. (2017). Metal phosphides as potential thermoelectric materials. J. Mater. Chem. C 5, 12441–12456. doi: 10.1039/C7TC03948D
Saparov, B., and Bobev, S. (2010). Isolated infinite chains in the Zintl phases Ba2ZnPn2(Pn = As, Sb, Bi) - Synthesis, structure, and bonding. Inorg. Chem. 49, 5173–5179. doi: 10.1021/ic100296x
Schäfer, K., Benndorf, C., Eckert, H., and Pöttgen, R. (2014). PbP7 – a polyphosphide with a three-dimensional [P7]2−− network of condensed and P-bridged P6 hexagons. Dalton Trans. 43, 12706–12710. doi: 10.1039/C4DT01539H
Shatruk, M. (2019). “Synthesis of phosphides,” in Fundamentals and Applications of Phosphorous Nanomaterials, ACS Symposium Series, ed H. Ji (Washington, DC: American Chemical Society), 103–134.
Shoemaker, D. P., Hu, Y.-J., Chung, D. Y., Halder, G. J., Chupas, P. J., Soderholm, L., et al. (2014). In situ studies of a platform for metastable inorganic crystal growth and materials discovery. Proc. Natl. Acad. Sci. U.S.A. 111, 10922–10927. doi: 10.1073/pnas.1406211111
Soheilnia, N., Xu, H., Zhang, H., Tritt, T. M., Swainson, I., and Kleinke, H. (2007). Thermoelectric properties of Re3Ge0.6As6.4 and Re3GeAs6 in comparison to Mo3Sb5.4Te1.6. Chem. Mater. 19, 4063–4068. doi: 10.1021/cm0708517
Tabassum, D., Lin, X., and Mar, A. (2015). Rare-earth manganese arsenides RE4Mn2As5 (RE = La - Pr). J. Alloys Compd. 636, 187–190. doi: 10.1016/j.jallcom.2015.02.145
Tan, X., Fabbris, G., Haskel, D., Yaroslavtsev, A. A., Cao, H., Thompson, C. M., et al. (2016a). A transition from localized to strongly correlated electron behavior and mixed valence driven by physical or chemical pressure in ACo2As2 (A = Eu, Ca). J. Amer. Chem. Soc. 138, 2724–2731. doi: 10.1021/jacs.5b12659
Tan, X., Garlea, V. O., Chai, P., Geondzhian, A. Y., Yaroslavtsev, A. A., Xin, Y., et al. (2016b). Synthesis, crystal structure, and magnetism of A2Co12As7 (A=Ca, Y, Ce–Yb). J. Solid State Chem. 236, 147–158. doi: 10.1016/j.jssc.2015.08.038
Tan, X., Garlea, V. O., Kovnir, K., Thompson, C. M., Xu, T., Cao, H., et al. (2017). Complex magnetic phase diagram with multistep spin-flop transitions in La0.25Pr0.75Co2P2. Phys. Rev. B, 95:024428. doi: 10.1103/PhysRevB.95.024428
Tan, X., Tener, Z. P., and Shatruk, M. (2018). Correlating itinerant magnetism in RCo2Pn2 pnictides (R = La, Ce, Pr, Nd, Ca, Pn = P, As) to their crystal and electronic structures. Acc. Chem. Res. 51, 230–239. doi: 10.1021/acs.accounts.7b00533
The National Academies of Sciences Engineering Medicine (2009). Frontiers in Crystalline Matter from Discovery to Technology. Washington, DC: The National Academies Press.
Thompson, C. M., Kovnir, K., Eveland, S., Herring, M. J., and Shatruk, M. (2011). Synthesis of ThCr2Si2-type arsenides from Bi flux. Chem. Commun. 47, 5563–5565. doi: 10.1039/c1cc10578g
Thompson, C. M., Kovnir, K., Garlea, V. O., Choi, E. S., Zhou, H. D., and Shatruk, M. (2014a). Unconventional magnetism in ThCr2Si2-type phosphides, La1−xNdxCo2P2. J. Mater. Chem. C. 2, 756–7569. doi: 10.1039/C4TC00564C
Thompson, C. M., Tan, X., Kovnir, K., Garlea, V. O., Gippius, A. A., Yaroslavtsev, A. A., et al. (2014b). Synthesis, structures, and magnetic properties of rare-earth cobalt arsenides, RCo2As2 (R = La, Ce, Pr, Nd). Chem. Mater. 26, 3825–3837. doi: 10.1021/cm501522v
Vasquez, G., Huq, A., and Latturner, S. E. (2019). In situ neutron diffraction studies of the metal flux growth of Ba/Yb/Mg/Si intermetallics. Inorg. Chem. 58, 8111–8119. doi: 10.1021/acs.inorgchem.9b00857
Wang, J., Greenfield, J. T., and Kovnir, K. (2017). Synthesis, crystal structure, and magnetic properties of R2Mg3SiPn6 (R = La, Ce, Pn = P, As). Inorg. Chem. 56, 8348–8354. doi: 10.1021/acs.inorgchem.7b01015
Wang, J., Lee, K., and Kovnir, K. (2015). Distorted phosphorus and copper square-planar layers in LaCu1+xP2 and LaCu4P3: synthesis, crystal structure, and physical properties. Inorg. Chem. 54, 890–897. doi: 10.1021/ic502234w
Wang, J., Mark, J., Woo, K. E., Voyles, J., and Kovnir, K. (2019). Chemical flexibility of mg in pnictide materials: structure and properties diversity. Chem. Mater. 31, 8286–8300. doi: 10.1021/acs.chemmater.9b03740
Wang, J., Xia, S-Q., and Tao, X-T. (2013). Syntheses, crystal structure and physical properties of new Zintl phases Ba3T2As4 (T = Zn, Cd). J. Solid State Chem. 198, 6–9. doi: 10.1016/j.jssc.2012.09.029
Wang, J., Yox, P., Voyles, J., and Kovnir, K. (2018). Synthesis, crystal structure, and properties of three La–Zn–P compounds with different dimensionalities of the Zn–P framework. Cryst. Growth Des. 18, 4076–4083. doi: 10.1021/acs.cgd.8b00445
Woo, K., Lee, K., and Kovnir, K. (2016). BP: synthesis and properties of boron phosphide. Mater. Res. Expr. 3:074003. doi: 10.1088/2053-1591/3/7/074003
Woo, K. E., Dolyniuk, J., and Kovnir, K. (2019a). Superseding van der Waals with electrostatic interactions: Intercalation of Cs into the interlayer space of SiAs2. Inorg. Chem. 58, 4997–5005. doi: 10.1021/acs.inorgchem.9b00017
Woo, K. E., Wang, J., Mark, J., and Kovnir, K. (2019b). Directing boron-phosphorus bonds in crystalline solid: oxidative polymerization of P=B=P monomers into 1D chains. J. Amer. Chem. Soc. 141, 13017–13021. doi: 10.1021/jacs.9b06803
Woo, K. E., Wang, J., Wu, K., Lee, K., Dolyniuk, J.-A., Pan, S., et al. (2018). Mg-Si-As: an unexplored system with promising nonlinear optical properties. Adv. Funct. Mater. 28:180158. doi: 10.1002/adfm.201801589
Yan, J.-Q., Sales, B. C., Susner, M. A., and McGuire, M. A. (2017). Flux growth in a horizontal configuration: an analog to vapor transport growth. Phys. Rev. Mater. 1:023402. doi: 10.1103/PhysRevMaterials.1.023402
Yao, T.-S., Tang, C.-Y., Yao Yang, M., Zhu, K.-J., Yan, D.-Y., Yi, C.-J., et al. (2019). Machine learning to instruct single crystal growth by flux method. Chin. Phys. Lett. 36:068101. doi: 10.1088/0256-307X/36/6/068101
Yu, T., Wang, S., Zhang, X., Li, C., Qiao, J., Jia, N., et al. (2019). MnSiP2: a new Mid-IR ternary phosphide with strong SHG effect and ultrabroad transparency range. Chem. Mater. 31, 2010–2018. doi: 10.1021/acs.chemmater.8b05015
Keywords: flux, crystal growth, phosphide, arsenide, salt flux, metal flux, self-flux
Citation: Wang J, Yox P and Kovnir K (2020) Flux Growth of Phosphide and Arsenide Crystals. Front. Chem. 8:186. doi: 10.3389/fchem.2020.00186
Received: 14 December 2019; Accepted: 28 February 2020;
Published: 02 April 2020.
Edited by:
Hans-Conrad zur Loye, University of South Carolina, United StatesReviewed by:
Dirk Johrendt, Ludwig Maximilian University of Munich, GermanyArthur Mar, University of Alberta, Canada
Copyright © 2020 Wang, Yox and Kovnir. This is an open-access article distributed under the terms of the Creative Commons Attribution License (CC BY). The use, distribution or reproduction in other forums is permitted, provided the original author(s) and the copyright owner(s) are credited and that the original publication in this journal is cited, in accordance with accepted academic practice. No use, distribution or reproduction is permitted which does not comply with these terms.
*Correspondence: Jian Wang, amlhbi53YW5nQHdpY2hpdGEuZWR1; Kirill Kovnir, a292bmlyQGlhc3RhdGUuZWR1
†ORCID: Jian Wang orcid.org/0000-0003-1326-4470
Kirill Kovnir orcid.org/0000-0003-1152-1912