- 1The Second Affiliated Hospital, Affiliated Traditional Chinese Medicine Hospital, Southwest Medical University, Luzhou, China
- 2Key Laboratory of Medical Electrophysiology of Ministry of Education, Drug Discovery Research Center, Southwest Medical University, Luzhou, China
- 3State Key Laboratory of Bioelectronics, School of Biological Science and Medical Engineering, Southeast University, Nanjing, China
Infections caused by antibiotic-resistant bacteria have become one of the most serious global public health crises. Early detection and effective treatment can effectively prevent deterioration and further spreading of the bacterial infections. Therefore, there is an urgent need for time-saving diagnosis as well as therapeutically potent therapy approaches. Development of nanomedicine has provided more choices for detection and therapy of bacterial infections. Ultrasmall gold nanoclusters (Au NCs) are emerging as potential antibacterial agents and have drawn intense attention in the biomedical fields owing to their excellent biocompatibility and unusual physicochemical properties. Recent significant efforts have shown that these versatile Au NCs also have great application potential in the selective detection of bacteria and infection treatment. In this review, we will provide an overview of research progress on the development of versatile Au NCs for bacterial detection and infection treatment, and the mechanisms of action of designed diagnostic and therapeutic agents will be highlighted. Based on these cases, we have briefly discussed the current issues and perspective of Au NCs for bacterial detection and infection treatment applications.
Introduction
The prevalence of pathogenic bacteria, especially multidrug-resistant bacteria, has become a serious global health crisis (Blair et al., 2015). Conventional antibiotics often appear to be incapable of responding to the prevalence of multidrug-resistant bacteria, either ineffective or inducing the emergence of new resistance after a period of use (Huh and Kwon, 2011). In particular, the emergence of ESKAPE superbugs even worsens the situation (Boucher et al., 2009). According to a statement from the Centers for Disease Control and Prevention, the world is on the verge of entering the “post-antibiotic era,” one where the death toll from bacterial infections than from cancer (Gupta et al., 2019). Therefore, there is an urgent need to develop alternative therapeutically effective antibacterial agents that are powerful and cost-effective enough to fight multidrug-resistant bacterial infections.
In addition to the treatment, the effective diagnosis of multidrug-resistant bacterial infections is also a huge challenge. Accurate and early detection of pathogenic bacteria is critical to identify infectious disease. Current techniques to detect bacteria include culture-dependent method, biochemical assays, PCR and sequencing, which are expensive and time-consuming (Lazcka et al., 2007; Ray et al., 2012; Yuan et al., 2018; Li D. et al., 2019). The lack of timely diagnosis has further worsened the condition of many patients with bacterial infections (Palestro and Love, 2009).
To overcome the drawbacks of conventional infection diagnostic and therapeutic strategies, various engineered nanomaterials have been used for diagnosis and treatment of bacterial infections (Disney et al., 2004; Kulagina et al., 2005; Yuan et al., 2014, 2018; Mahlapuu et al., 2016). Among these alternative agents, ultrasmall metal nanoclusters, in particular Au NCs, have attracted significant attention for diagnosis and treatment of bacterial infections. Gold-based NCs have intrinsic advantages such as facile syntheses, extremely large surface area, excellent biocompatibility, strong photoluminescence, high photostability, and easy functionalization with other biomolecules. Benefits from these excellent physicochemical properties, Au NCs have great promise in biomedical applications, such as sensing, imaging, and diseases treatment (Chen L. Y. et al., 2015; Zheng Y. et al., 2017; Hu et al., 2018; Chen et al., 2019). The antibacterial activity of Au NCs has been also innovatively explored over the past few years (Zheng K. et al., 2017; Zheng et al., 2018a,c; Xie et al., 2018). Apart from antibacterial activity, unusual photoluminescence properties of Au NCs also provide potential applications for their use as detection/imaging agents for bacterial pathogens (Chan and Chen, 2012; Zheng et al., 2018d; Li D. et al., 2019). Obviously, both diagnosis and treatment are essential to control the prevalence of multidrug-resistant bacterial infections. Moreover, the in-depth understanding of the fundamental principles of diagnosis and treatment plays a key role in designing bacterial biosensors and antimicrobial agents. In this review, we will summarize the efforts of Au NCs for diagnosis and treatment of bacterial infections in the recent decade as Au NCs may provide solutions to address these intractable challenges for bacterial infections (Scheme 1). Based on the overview of Au NCs, we firstly summarize the recent progress of Au NCs for bacterial detection, containing the probes design, sensitivity, and selectivity of miscellaneous gold-based NCs. Then we discuss the antibacterial activity on basis of the mechanisms by different Au NCs. Physicochemical properties of Au NCs such as surface chemistry, photoluminescence, and size that affect the antibacterial behavior or detection performance are analyzed to offer insight on the further rational design of new diagnostic and therapeutic agents. Finally, a brief discussion of current problems and future developments of Au NCs for diagnosis and treatment of bacterial infections is provided.
ABOUT Au NCs
Au NCs refer to gold species containing a few to several hundred Au atoms, with their dimensions below a critical size for electronic energy quantization. According to the free-electron model, the critical size for Au is ~2 nm, which is comparable to the Fermi wavelength of electrons (Zhang and Wang, 2014; Yang et al., 2015). In this size regime, the strong quantum confinement of free electrons leads to the discrete electronic states and thus Au NCs exhibit molecule-like properties, such as HOMO-LUMO transition, large Stokes shift, and strong photoluminescence (Goswami et al., 2016; Song et al., 2016). Au NCs show dramatically different optical and chemical properties from those of larger gold nanoparticles (NPs). For example, Au NCs do not possess surface plasmon resonance (SPR) absorption in the visible region but exhibit apparent fluorescence emission in the near-infrared (NIR) to visible region (Zheng Y. et al., 2017). In addition to ultrasmall size, many studies have also revealed that the optical properties of Au NCs highly depend on their structures, oxidation states, and surface ligands as well as environmental parameters such as temperature, pH, and ionic strength (Zheng Y. et al., 2017; Chen et al., 2019). As a bridge between single Au atom and plasmonic NPs, Au NCs have received increasing attention in many fields, including bacterial detection described in the following sections. Up to now, several reviews have been dedicated to the ultrasmall Au NCs (Luo et al., 2014; Jin et al., 2016; Zheng Y. et al., 2017; Chen et al., 2019).
To prepare stable and high quality Au NCs, z, polymers, peptides, DNA, and proteins that act as capping agents are required when using various synthetic methods, including chemical reduction, photoreduction, electroreduction, and chemical etching (Zheng Y. et al., 2017). To further benefit and broaden applications of Au NCs, it is necessary for further functionalization with surface ligands (e.g., folic acid, proteins) on Au NCs, commonly via ligand exchange, bioconjugation, and non-covalent interaction (Jin et al., 2016; Song et al., 2016; Zheng Y. et al., 2017).
In addition to the advantages of easy preparation and unique physicochemical properties, the excellent biocompatibility is also the reason why Au NCs have received widespread attention, especially in biomedical fields. Indeed, as the “noblest” metals, gold, is inert, highly stable, and would not easily dissociate into ions (Hammer and Norskov, 1995). These features contribute to the widely accepted notion of Au NPs as being highly biocompatible in mammalian system, both in vitro and in vivo (Connor et al., 2005; Lewinski et al., 2008). This biocompatibility in mammalian cells still remains when the size locates in the range of NCs (Pan et al., 2007; Li et al., 2016). For example, utilizing the in vitro multiple cell models, no cytotoxic effect was observed on the cells exposed with Au NCs (Zheng et al., 2018b). Conversely, they were found to improve cell metabolism and overall cell proliferation. In animal studies, they show improved tumor uptake and high renal clearance (Zhang et al., 2012; Liang et al., 2017; Yu et al., 2019). Interestingly, they showed significant cytotoxicity against prokaryotic bacterial cells as opposed to eukaryotic mammalian cells. This selective cytotoxicity may be resulted from the limitations of lysosomal phagocytosis and mitochondrial obstacles in mammalian cells (Marrache and Dhar, 2012). Note that it is always critical to tightly control the surface properties of Au NCs as they can potentially affect the toxicity.
Bacterial Detection With Gold-Based Ncs
Label-Free Detection of Bacteria
Depending on the specific fluorescence changes caused by bacterial cells, photoluminescent gold-based NCs can be employed for label-free fluorescence detection of bacteria. For example, Chan and Chen found that human serum albumin protected gold nanoclusters (HSA-Au NCs) can act as selective fluorescent probes for S. aureus and methicillin-resistant S. aureus (MRSA) (Chan and Chen, 2012). HSA-Au NCs can bind to S. aureus and MRSA with high specificity, resulting in a significant fluorescence enhancement (Figure 1A). In another study, Yan and coworkers designed an on-off-on probe based fluorescent Au NC for rapid and selective detection of Escherichia coli, by hijacking the unique Cu2+-binding and redox pathways of E. coil to recover the photoluminescence of Au NC from copper-caused quenching (Figure 1B) (Yan et al., 2018). Based on this fluorescent probe, it can successfully allow the rapid determination and detection of E. coli in artificially contaminated water with trace concentrations of bacteria (89 CFU/mL) within 30 min, showing great application prospects for rapid point-of-care analysis of pathogenic E. coli in environment monitoring and clinical diagnosis.
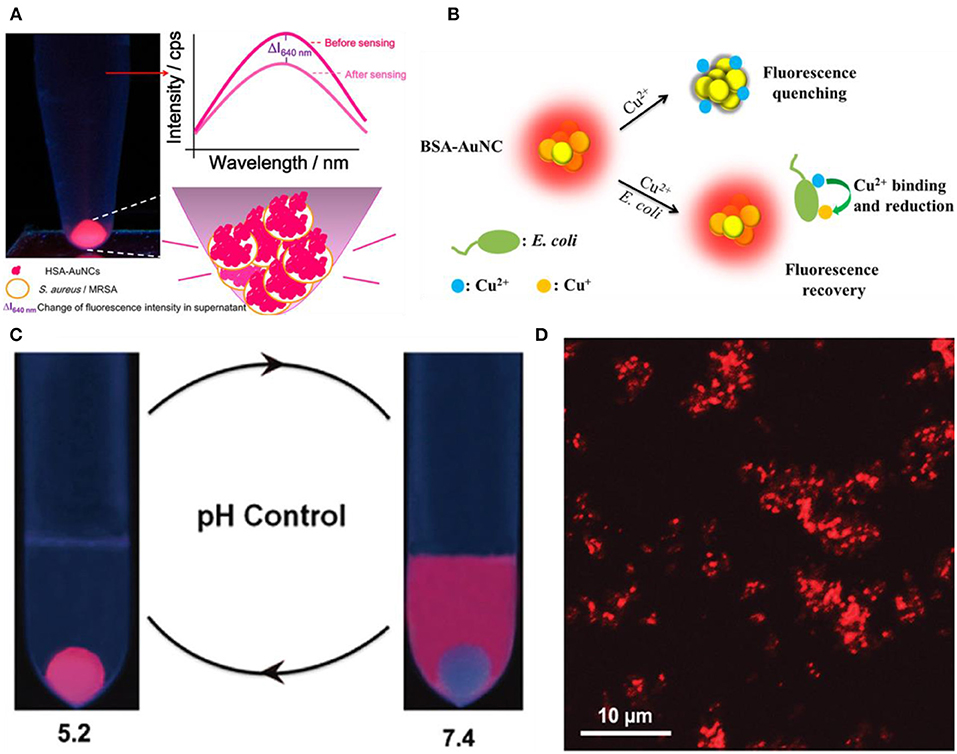
Figure 1. (A) Luminescent HSA-Au NCs as selective probes for Staphylococcus aureus and MRSA. Reproduced from Chan and Chen (2012) with permission from American Chemical Society. (B) Schematic illustration of the working principle for the Cu2+ mediated on-off-on Au NC-based fluorescent probe for rapid Escherichia coli detection. Reproduced from Yan et al. (2018) with permission from American Chemical Society. (C) Simplified scheme of pH controllable adherence of CP-GNC to E. coli cells. Specially, CP-GNC was fully attached to the cells at pH 5.2, whereas all the CP-GNC detached from the surface of E. coli cells at pH 7.4. (D) Bacterial cells can be efficiently labeled and form cell clusters using CP-GNC. Reproduced from Liu P. et al. (2015) with permission from Wiley-VCH Verlag & Co. KGaA, Weinheim.
On the other hand, pH-responsive Au NCs can control the labeling of bacterial cells through pH regulation. Liu et al. developed a new method for the preparation of cross-linked protein (bovine serum albumin, BSA) with Au NCs (CP-GNC) (Liu P. et al., 2015). BSA is a typical amphoteric electrolyte, which means the surface net charges of CP-GNC can be adjusted by environmental pH due to the gain or loss of protons. Since bacterial cells are mainly negatively charged, the adhesion to and release of CP-GNC from E. coli cells can be easily controlled via modulating the pH (Figure 1C). Notably, CP-GNC-based fluorescent probe provides a solution for the label-free detection of E. coli. The confocal microscopy images showed that bacteria were efficiently labeled by this probe and formed cell clusters at pH 5.2 (Figure 1D). In another work, the antimicrobial peptide stabilized Au NCs also exhibits pH-responsive bacterial binding effect, which were useful for the fluorescence detection and imaging of bacterial infection (Pranantyo et al., 2019). Our recent study showed that the photoluminescence intensity of thiolated Au NCs can be significantly enhanced by silver ion doping (Zheng et al., 2018d). The strong photoluminescence of AuAg NCs (Ag-doped Au NCs) can be selectively and rapidly quenched by Acinetobacter baumannii via agglomeration of NCs, which allows the label-free detection of A. baumannii with a limit of detection (LOD) of 2.3 × 103 colony forming unit (CFU)/mL (Figure 2). This study may provide a rapidly alternative strategy for the analysis of A. baumannii in clinical samples. Nevertheless, further study is still essential to produce an updated version of these materials with high selectivity and sensitivity toward specific bacterial species by combining bacteria recognizing components.
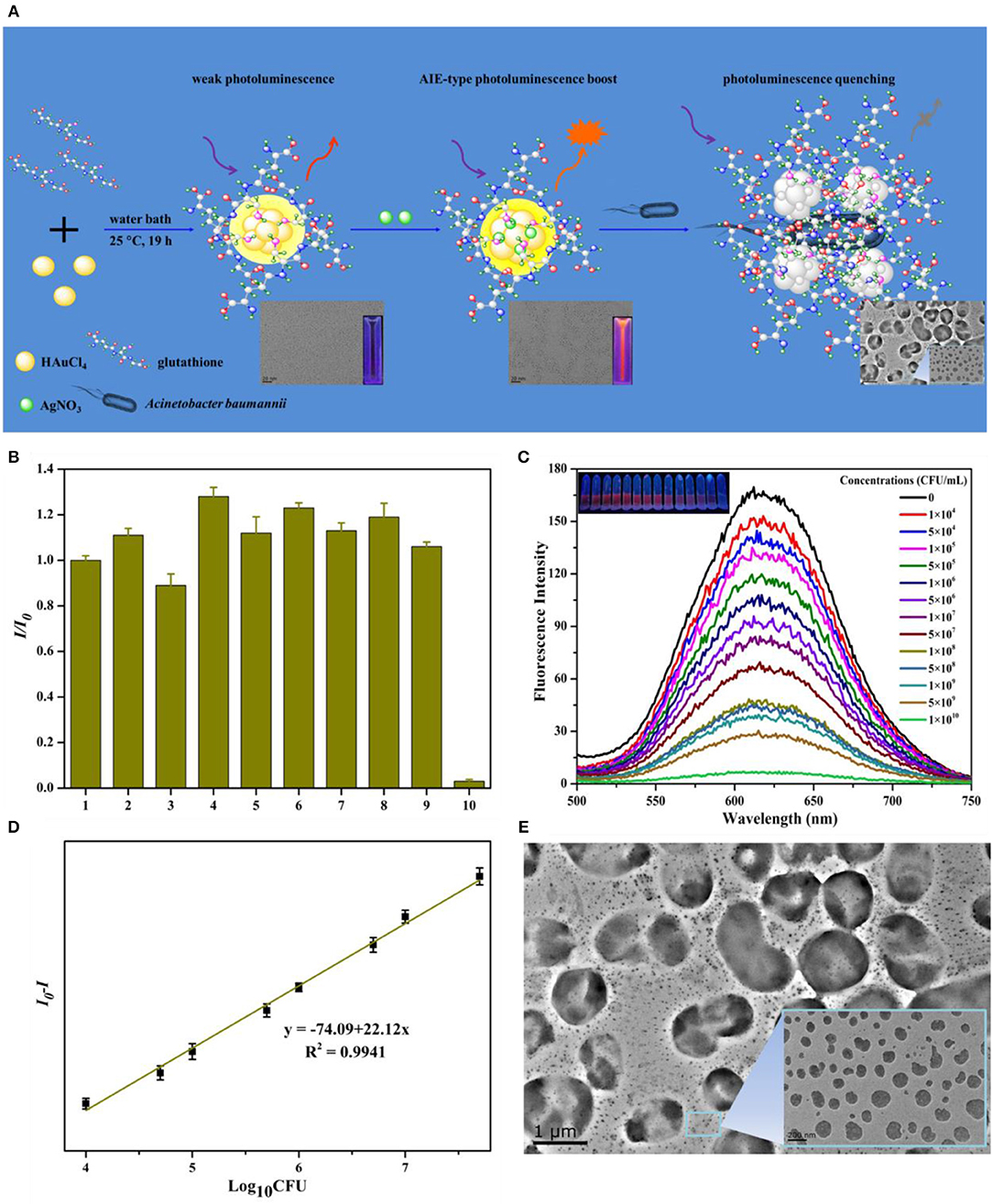
Figure 2. (A) Schematic illustration of the syntheses of AuAg NCs and photoluminescent quenching by Acinetobacter baumannii. (B) The fluorescence of AuAg NCs was selectively quenched by A. baumannii. The order numbers from 1 to 10 in turn represent the group in the presence of no bacteria (control), Bacillus mycoides, Staphylococcus aureus, methicillin-resistant S. aureus, Candida albicans, P. aeruginosa, E. coli, vancomycin-resistant Enterococcus faecium, Saccharomyces cerevisiae, and A. baumannii, respectively. (C) The concentration dependent quenching effect of A. baumannii toward AuAg NCs. Insets: Digital photos of AuAg NCs under UV illumination after treatment with different concentrations A. baumannii. (D) Relative fluorescence intensity (I0-I) of AuAg NCs in contrast to the logarithm of the A. baumannii concentrations. (E) The transmission electron microscopy (TEM) micrographs of photoluminescent AuAg NCs treated with 1 × 105 CFU/mL A. baumannii demonstrate the bacteria induced agglomeration of NCs. Reproduced from Zheng et al. (2018d) with permission from Elsevier Ltd.
Recognition Through Molecular Motifs
The main drawback of using fluorescence Au NCs for bacterial detection is that their selectivity is generally non-ideal (Chen et al., 2019). To significantly improve the selectivity and efficiency, the common strategy is to decorate the clusters with ligands that recognize receptors on bacterial cells. For instance, Mukherji and coworkers functionalized Au NCs with acyl homoserine lactone (AHL) quorum sensing signal molecules that could recognize the Lux-R family regulators in E. coli (Figure 3A) (Mukherji et al., 2013). This decoration allows differentiation of E. coli from S. aureus suspensions that do not produce this special receptor. Khlebtsov et al. used highly fluorescent BSA-capped Au NCs decorated with human antistaphylococcal immunoglobulin (antiSAlgG) for targeted detection of S. aureus in bacterial mixtures (Khlebtsov et al., 2015). Compared with non-specific electrostatic binding of HSA-Au NCs to S. aureus at pH around 5–6, this biosensor can show an enhanced selectivity at the physiological pH of 7.4.
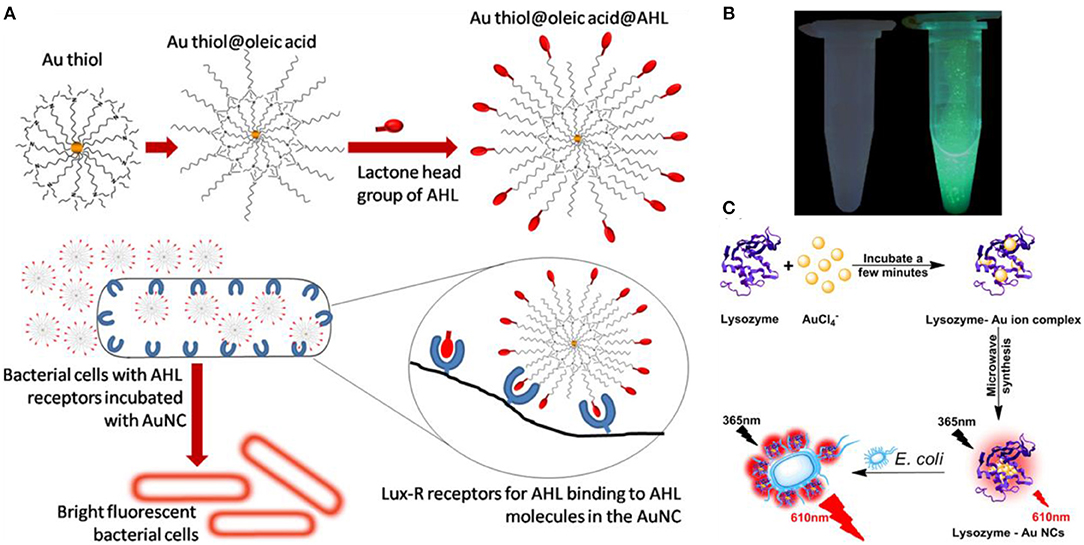
Figure 3. (A) Interaction of the fluorescent probe with bacterial cells: structure of the probe with AHL signal molecules deployed on the surface with lactone and amide moieties intact (top) and specific binding of AHL head groups to receptor sites in Lux-R regulators within bacteria (bottom). Reproduced from Mukherji et al. (2013) with permission from American Chemical Society. (B) Visualization of mannose-protected Au NCs (25 nM) in the absence (left) and presence (right) of E. coli (2.5 × 108 CFU/mL) upon excitation under a hand-held UV lamp (365 nm). Reproduced from Tseng et al. (2011) with permission from Elsevier B.V. (C) Schematic diagram of the synthesis of the red fluorescent lysozyme-Au NCs and fluorescence enhancement detection of E. coli. Reproduced from Liu J. et al. (2015) with permission from Elsevier B.V.
Type 1 fimbriae present on the surface of Enterobacteriaceae, such as E. coil, are responsible for their mannose- and mannoside-binding active sites (Soto and Hultgren, 1999; Harris et al., 2001). This family of proteins contains FimA, FimF, FimG, and FimH, and FimH is uniquely responsible for the binding to mannose. Mannose-etched Au NCs were used as special recognizer to develop a simple approach for fluorescence detection of E. coli (Huang et al., 2009; Tseng et al., 2011). The Au NCs bind to E. coli through the multivalent interactions between the NCs and FimH on the bacterial pili of E. coli, resulting in brightly fluorescent cell clusters (Figure 3B). The fluorescent signal was linearly proportional to the bacterial concentration, monitoring the fluorescence changes of Au NCs allowed the detection of E. coli with a LOD of 150 CFU/mL (Tseng et al., 2011). In addition, microwave-assistant synthesized mannose-protected Au NCs are also capable of selectively detecting the E. coli J96, a urinary tract infection isolate, by binding to FimH protein expressed on the type 1 pili (Chan et al., 2013). A similar recognition mechanism was also employed to establish specific probe for detection of Listeria monocytogenes (Hossein-Nejad-Ariani et al., 2018).
Besides surface receptors, enzymes such as lysozyme can recognize bacteria by binding to their specific site on cell surface (Li D. et al., 2019). Therefore, lysozyme-decorated Au NCs may be used for specific identification of bacteria. For instance, a point-of-care detection strategy for analysis bacteria has been established by using lysozyme-protected Au NCs that are prepared through a one-pot synthesis and reserved specific identification capability for E. coli (Liu J. et al., 2015). Based on the specific recognition, lysozyme-decorated Au NCs could selectively anchor onto the surface of E. coli, leading to strong red photoluminescence boost (Figure 3C). This strategy should be generalizable, and fluorescent Au NCs decorated with other recognition motifs could also be used to sense pathogenic bacteria.
To further improve the selectivity and sensitivity of bacterial detection, dual recognition probes based on fluorescent Au NCs have also been developed. Song and coworkers developed a dual recognition approach that integrates DNA aptamer and antibiotic-based dual recognition units, which enables sensitive and selective fluorescent detection of S. aureus in presence of ultrahigh concentrations of other bacteria strains (Figure 4A) (Cheng et al., 2016). Aptamer-decorated magnetic beads were used for specific capture of S. aureus. In another work, vancomycin-stabilized fluorescent Au NCs (Au NCs@Van) were employed for sensitive quantification of S. aureus with a LOD of 16 CFU/mL by measuring their photoluminescence intensity. Indeed, vancomycin can combine with S. aureus by binding onto terminal D-alanyl-D-alanine residues of N-acetylmuramic acid and N-acetylglucosamine peptide subunits on the cell wall of the gram-positive bacteria, which will make the Au NCs adhere to the surface of S. aureus (Xing et al., 2002; Chung et al., 2011). Using this strategy, about 70 CFU/mL of S. aureus in complex samples could be successfully sensed. Relying on vancomycin and aptamer as dual recognition molecules, Song et al. further generalized a universal strategy for selective detection of S. aureus using a dual-recognition motif-based fluorescence resonance energy transfer (FRET) platform (Figure 4B) (Yu et al., 2017). Within 30 min, by using Au NCs@Van and aptamer-modified Au NPs as the energy donor and acceptor, respectively, the FRET signal shows a linear variation with the concentration of S. aureus in the range from 20 to 108 CFU/mL with a LOD of 10 CFU/mL. This dual-recognition FRET strategy showed recoveries from 99.00% to the 109.75% for sensing S. aureus in real samples, which have great application potential in infectious disease diagnosis and environmental monitoring. In another study, nanocapsules with antibody-functionalized Au NCs combined in chitosan (Au NCs@CS) and immunomagnetic NPs were employed to ultrasensitive recognize E. coli O157:H7 (Figure 4C) (Cheng et al., 2018). After separation by magnetic fields, E. coli O157:H7 were isolated attached to the immunomagnetic NPs and quantified by the fluorescent changes of Au NCs@CS linked to bacteria.
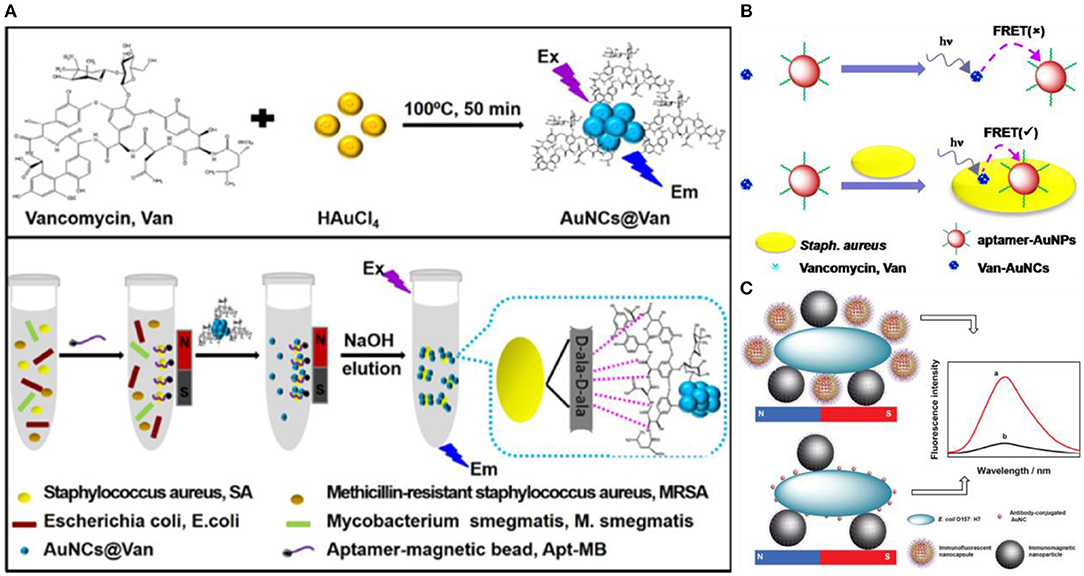
Figure 4. (A) Schematic illustrations of (top) one-step preparation of Au NCs@Van and (bottom) determination of S. aureus in mixtures using the aptamer-coated magnetic beads and Au NCs@Van dual recognition strategy. Reproduced from Cheng et al. (2016) with permission from American Chemical Society. (B) Illustration of the vancomycin and aptamer dual-recognition molecule based FRET assay platform for S. aureus. Reproduced from Yu et al. (2017) with permission from American Chemical Society. (C) Illustration of the immunoassay of E. coli O157:H7 using Au NCs@CS nanocapsules and Au NCs as labels. Reproduced from Cheng et al. (2018) with permission from The Royal Society of Chemistry.
In addition, a recent study showed that the mimic enzyme catalytic properties of Au NCs can also be exploited for colorimetric differentiation of pathogenic bacteria (Xie et al., 2019). A UV-assisted peroxidase-like Au NC sensor with an aptamer specific to S. aureus was developed. S. aureus was attached to the probe, which allows the catalyzed decomposition of hydrogen peroxide to hydroxyl radicals (•OH). The substrate 3,3′,5,5′-tetramethylbenzidine (TMB) was concomitantly oxidized to blue product ox-TMB by •OH. This colorimetric sensor easily differentiates S. aureus from E. coli and B. subtilis within 30 min, with a LOD of 4 × 102 CFU/mL. Indeed, nanoenzymes are widely used in analytical chemistry (Wang et al., 2018; Huang et al., 2019), and the development of biosensors for the bacterial detection based on Au NC with mimic enzyme-like catalytic activities has very attractive application prospects.
Sensor Arrays
To achieve simultaneous detection of multiple bacteria, sensor arrays based on Au NCs have also been developed. For instance, Qu and coworkers designed and prepared a bacterial sensor array based on the integration of HSA-Au NCs, lysozyme (Lyz)-Au NCs, lactoferrin (Lf)-Au NCs, and vancomycin decorated HSA-Au NCs (Van-Au NCs) (Figure 5) (Ji et al., 2018). HSA-Au NCs are selected based on the interaction between the peptide motifs on the surface of HSA and the bacterial cell wall (Chan and Chen, 2012). Lysozyme can recognize and kill bacteria by binding to the cell surface polysaccharide (Vocadlo et al., 2001). Lf-Au NCs can serve as a probe since many bacteria can express lactoferrin receptors with high affinity to lactoferrin (Xavier et al., 2010). The strong affinity of Van to D-alanyl-D-alanine dipeptide on bacterial cell walls endows Van-Au NCs with a high binding affinity to both gram-positive and gram-negative bacteria (Xing et al., 2002; Chung et al., 2011). The subtle changes in the physicochemical properties on different bacterial surfaces would induce different interactions with the probes in the sensor array. Based on the sensor array, six types of bacteria, including Alcaligenes faecalis, B. subtilis, S. aureus, MRSA, E. coli, and kanamycin-resistant E. coli were distinguished on the sensor array. Similarly, a bacterial sensor array based on metal ion modified Au NCs was established (Wu et al., 2018). In another study, Yang and coworkers fabricated a sensor array based on Ag-Au alloy NC-Au NP composite for the discrimination of sulfur-oxidizing bacteria (Yang et al., 2019). The non-sulfur (S. aureus and E. coli) and sulfur-oxidizing bacteria (Citreicella thiooxidans, Thiobacimonas profunda, and Acidithiobacillus caldus) were well distinguished at a level of OD600 = 0.005. In summary, the development of these sensor arrays might offer new perspectives for analyzing intricate bacterial infections.
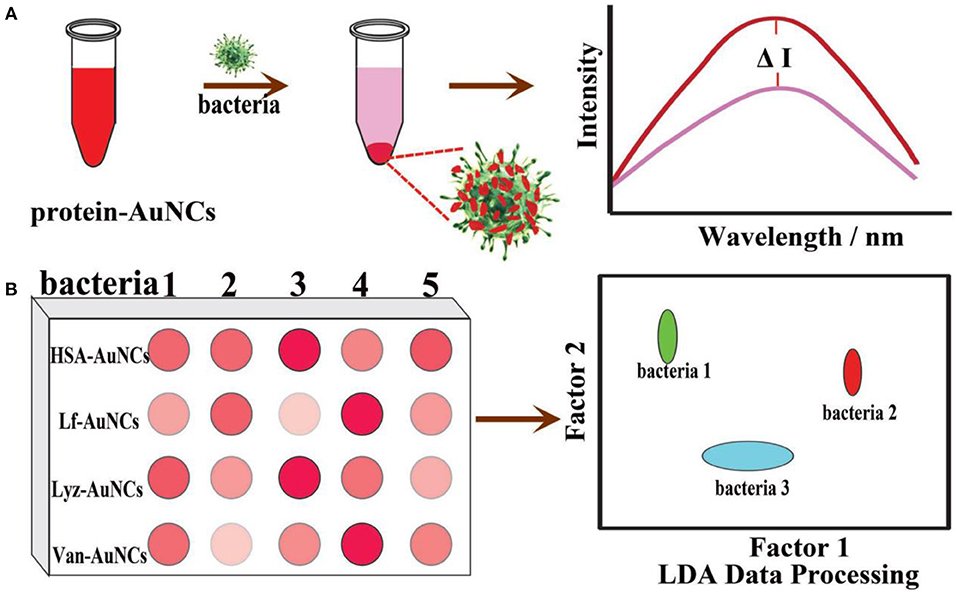
Figure 5. Schematic illustration of protein-Au NC-based fluorescence sensor array for discrimination of various bacteria. (A) The fluorescence intensity of protein-Au NCs was significantly reduced in the presence of bacteria. (B) A schematic fluorescence pattern generated from the different responses of the protein-Au NCs probes toward bacteria. Reproduced from Ji et al. (2018) with permission from Wiley-VCH Verlag & Co. KGaA, Weinheim.
As mentioned above, Au NCs-based bacterial biosensors may provide a promising alternative platform for detection and discrimination of pathogenic bacteria. However, there are still great challenges that limited their practical applications. First of all, the stability of Au NCs is essential in their practical use. In general, the as-synthesized Au NCs are not comprised of a single component, and they are usually a complicated mixture system of multiple Au species, leading to the bacterial biosensors based on Au NCs are less reproducible. To overcome this problem, atomic precision controlled synthesis of Au NCs could be utilized to improve their reproducibility. Secondly, the detection selectivity should be further improved to work in the biomatrices. Functionalization of fluorescent Au NCs with specific recognition motifs via surface chemistry may be helpful to address this problem. In addition, fundamental understanding of the fluorescence mechanisms of Au NCs is vital to develop bacterial sensors. In this aspect, understanding of their photoluminescence mechanisms such as FRET will help to optimize the detection strategy (Yu et al., 2017).
Au NCs as Antibacterial Agents
In addition to bacterial detection, ultrasmall Au NCs are also developed as an innovative nanomedicine for the treatment of multidrug-resistant bacterial infections in recent years. The antibacterial activity of these Au NCs usually results from the antibiotics delivery, generation of reactive oxygen species (ROS), and damage of cell membrane and cellular contents. Usually the antibacterial activity is affected by the size and surface chemistry of NCs. In this section, we will summarize in details the progress made by Au NCs in the treatment of bacterial infections and classify them into different antibacterial systems based on the fundamental components of Au NCs, including antibiotic-Au NCs systems, antimicrobial peptide-Au NCs systems, small molecule-Au NCs systems, macromolecule-Au NCs systems, and Au NCs-containing combination systems (Table 1).
Antibiotic-Au NCs Systems
The abuse of antibiotics and low utilization rate are one of the main causes of antibiotic resistance (Li X. et al., 2019). One feasible solution for reducing the abuse of antibiotics and improving the effect involves the use of assembled structures that have adjustable antibacterial activity. As a versatile platform, Au NCs can be widely used in antibiotic loading to improve internalization of antibiotics into bacteria, thereby improving the efficacy of antibiotics. In addition, some Au NCs possess inherent antibacterial activity, and may exert certain polyvalent and synergistic effects through antibiotic loading to enhance the antibacterial activity of nanosystems (Zhang et al., 2014; Zheng Y. et al., 2019). For example, Kalita et al. developed a potent antibacterial hybrid prepared through surface functionalization of lysozyme-capped Au NCs (Lys-Au NCs) with β-lactam antibiotic ampicillin (Lys-Au NCs-Amp) (Kalita et al., 2018). The antibacterial hybrid not only reverses the MRSA resistance toward ampicillin but also exhibits enhanced antimicrobial activity against non-resistant bacterial strains. With the help of cis-2-decenoic acid, Lys-Au NCs-Amp can also inhibit the MRSA persister, a dormant body of bacteria. This antibacterial hybrid may eradicate MRSA infections from difficult-to-treat diabetic wound of rat and accelerate the healing process. Antibacterial mechanism studies have shown that the antibiotic effect of Lys-Au NCs-Amp against MRSA and its persister is due to the increased concentration of ampicillin at the action site, the multivalent presentation and the enhanced permeation of ampicillin via lysozyme-mediated cell lysis. In another study, the self-regulated vancomycin loading and release capabilities of custom-designed pentapeptide-capped Au NCs (Pep-Au NCs) were developed on basis of the strong binding affinity of vancomycin with D-alanine-D-alanine termini (Li et al., 2018). The self-assembly Au NCs super-structure can spontaneously release vancomycin upon exposure to gram-positive bacteria due to the stronger binding affinity of vancomycin with bacteria than that with Pep-Au NCs. Note that the formation of this structure does not mitigate the efficacy of the vancomycin. The on-demand drug release of Pep-Au NCs avoids the systemic distribution of vancomycin and reduces the potential side effect. Besides as a cargo, vancomycin can also be directly used as a reducing agent and template to fabricate the water-soluble, monodispersed Au NC (Liang et al., 2018), which have excellent antibacterial activities toward both gram-positive and gram-negative bacteria. This encouraging result suggests that loading antibiotics with Au NCs may broaden the antibacterial spectrum of antibiotics themselves, rendering their broader antibacterial applications. In addition, bacitracin-templated Au NCs (Wang S. et al., 2019), cefradine-synthesized Au NCs (Khandelwal et al., 2015), and chloramphenicol-loaded Au NCs (Liu P. et al., 2015) have also been developed for the treatment of multidrug resistant infections.
Antimicrobial Peptide-Au NCs Systems
Except antibiotics, antimicrobial peptide-functionalized Au NCs have also been developed as promising therapeutic for multidrug-resistant infections. Antimicrobial peptides are produced by organisms to defend themselves against pathogenic bacteria (Rajchakit and Sarojini, 2017). The common and generally accepted mechanism of action of antimicrobial peptides is perturbation or complete lysis of bacterial membranes relying on their distinctive amino sequences that can insert into bacterial membrane (Hancock and Sahl, 2006; Hassan et al., 2012; Hilchie et al., 2013). In a previous study, Chen et al. prepared a ~2.5 nm Au NCs-based antibacterial structure via etching and co-deposition of 1-dodecanethiol (DT) and antimicrobial peptide surfaction (SFT) on gold NPs (Figure 6A) (Chen W. Y. et al., 2015). The as-synthesized SFT/DT-Au nanodots (NDs, ~2.5 nm) show significant antibacterial behavior and their antibacterial activities are highly dependent on the density of SFT on NDs. Relative to SFT alone, the antibacterial hybrid exhibit stronger antibacterial activity to multidrug-resistant bacteria (Figure 6B). The total antibacterial activity is mainly attributed to the synergistic effect of SFT and NDs on the disruption of the bacterial cell membrane. In our recent study, we developed an effective antibacterial hybrid (Dap-Au NCs) by incorporating antimicrobial peptide (daptomycin, Dap) and antibacterial Au NCs (Zheng et al., 2018c; Zheng Y. et al., 2019). The antibacterial hybrid could high-efficiently damage bacterial cell membrane because Dap moiety may induce the creation of holes on the cell membranes, and motivate the entry of Dap-Au NCs inside bacteria and even lead to serious DNA destruction (Figure 7A). In addition, Dap-Au NCs can also promote the generation of free radicals such as ROS within bacteria, which may also limit the evolution of drug resistance in bacteria. In another work, cysteine-terminated antimicrobial peptide was employed as a reducing ligand to prepare Au NCs (Pranantyo et al., 2019). The citraconyl amide on the surface of Au NCs could auto-cleave to re-expose the cationic amine at low pH. As a result, the NCs are stable and non-cytotoxic under physiological conditions, but can switch into a cationic bactericidal mode in an acidic environment that is commonly encountered at bacterial infection areas (Figure 7B).
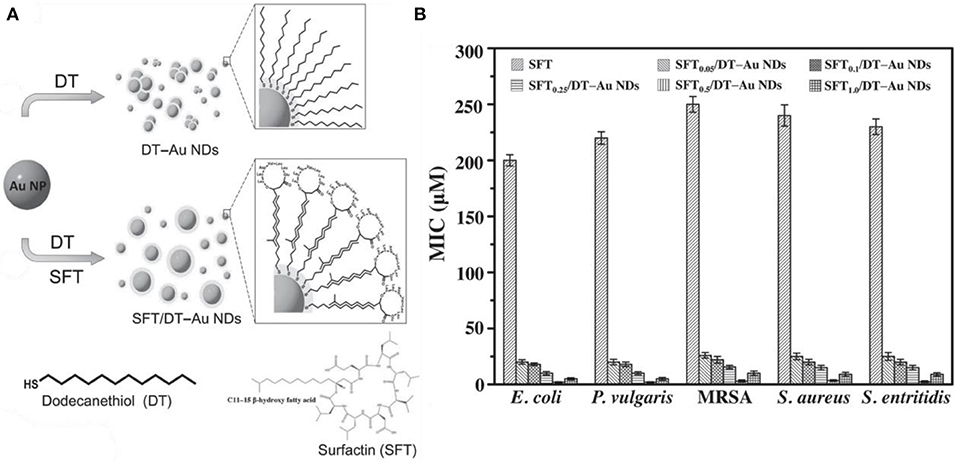
Figure 6. (A) Synthesis of photoluminescent SFT/DT-Au NDs. (B) Comparison of MICs (in terms of the concentration of SFT) of SFT, SFT0.05/DT-Au NDs, SFT0.1/DT-Au NDs, SFT0.25/DT-Au NDs, SFT0.5/DT-Au NDs, and SFT1.0/DT-Au NDs against E. coli, P. vulgaris, MRSA, S. aureus, and Salmonella enterica, respectively. Error bars represent the standard deviation of three repeated measurements. Reproduced from citetbib9 with permission from Wiley-VCH Verlag & Co. KGaA, Weinheim.
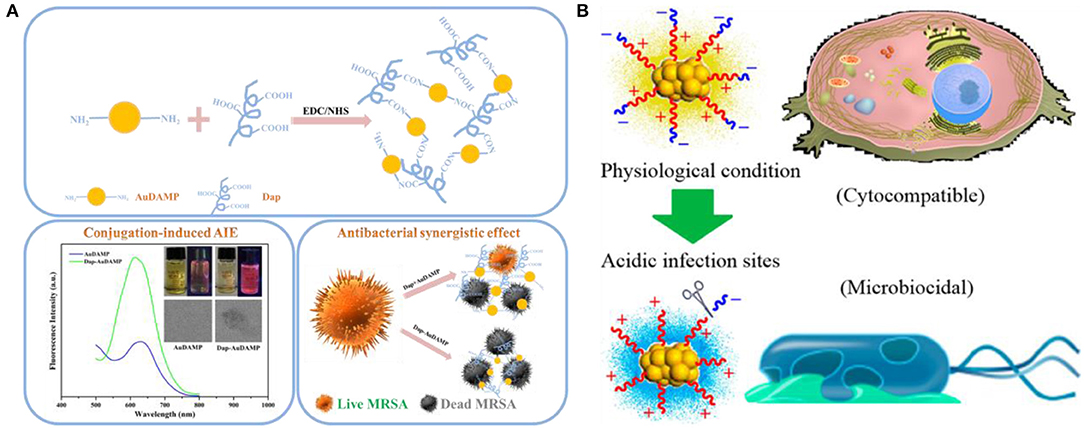
Figure 7. (A) Schematic illustrations of the conjugation strategy for antibacterial Au NCs and Dap, conjugation-induced aggregation-induced emission enhancement, and antibacterial synergistic effect. Reproduced from Zheng Y. et al. (2019) with permission from Elsevier Inc. (B) Schematic illustration of antimicrobial peptide-reduced Au NCs with charge-reversal moieties for antibacterial application. Reproduced from Pranantyo et al. (2019) with permission from American Chemical Society.
Small Molecule-Au NCs Systems
Compared with passive drug carriers, non-antibiotic small molecule-functionalized Au NCs can directly obtain antibacterial ability through precise size and surface chemistry regulations, which showed great potential as an alternative for commercial antibiotics. In a pioneering work, Zheng et al. demonstrated that ultasmall Au NCs (<2 nm) may own antibacterial activity that not observed for large-sized Au NPs with same ligands (Zheng K. et al., 2017). The synthesized atomic precision 6-mercaptohexanoic acid (MHA)-templated Au NCs (Au25MHA18) showed a wide-spectrum antibacterial activity and exhibited interactions with both gram-negative and gram-positive bacteria to induce intracellular metabolic disorders after the internalization of Au25MHA18, and result in an increase of intracellular ROS generation that killed bacteria consequently (Figure 8A). However, the large-sized MHA-Au NPs (~6 nm) cannot induce ROS generation and therefore did not possess antibacterial capability. Indeed, the induction of ROS generation is the dominant antibacterial mechanism of action of Au NCs (Chang et al., 2019). Similar circumstances have been witnessed in our recent work. We demonstrated that mercaptopyrimidine analogs templated Au NCs can serve as potent nanoantibiotics for ESKAPE superbugs (Zheng et al., 2018c). Mercaptopyrimidine analogs, including 4,6-dihydroxyl-2-mercaptopyrimidine (DHMP), 4-amino-6-hydroxyl-2-mercaptopyrimidine (AHMP), 4,6-diamino-2-mercaptopyrimidine (DAMP), and 4-amino-2-mercaptopyrimidine (AMP), were employed as templates and reducing agents to prepare Au NCs. Unlike large-sized Au NPs, the as-prepared Au NCs especially AuDAMP possess excellent antibacterial capabilities against both gram-negative and gram-positive bacteria (Figure 8B). The Au NCs kill ESKAPE via a combined mechanism including cell membrane damage, DNA destruction, and ROS production. Moreover, the induction of ROS generation in bacteria is mainly attributed to intrinsic oxidase- and peroxidase-like catalysis by Au NCs. In contrast, large-sized AuDAMP NPs exhibit relatively weak antibacterial activity due to their weak enzyme mimic activity. Broadening the antibacterial spectrum of Au NCs by co-functionalizing albumin and DAMP has also been reported (Sun et al., 2019). Quaternary ammonium-functionalized Au NCs (QA-Au NCs) have been utilized to treat bacterial infections through the combined physicochemical mechanisms including cell membrane disruption, ROS generation, and disturbance of intracellular metabolic pathways (Figure 9) (Xie et al., 2018). These NCs can specifically target and kill antibiotic-resistant gram-positive superbugs including MRSA and vancomycin-resistant Enterococcus. Mannose-protected Au NCs were also found to selectively and efficiently inhibit the proliferation of E. coli through Au NCs-induced agglutination (Tseng et al., 2011). Furthermore, the use of antibacterial Au NCs for biofilm inhibition of multidrug-resistant bacteria has also been demonstrated (Boda et al., 2015).
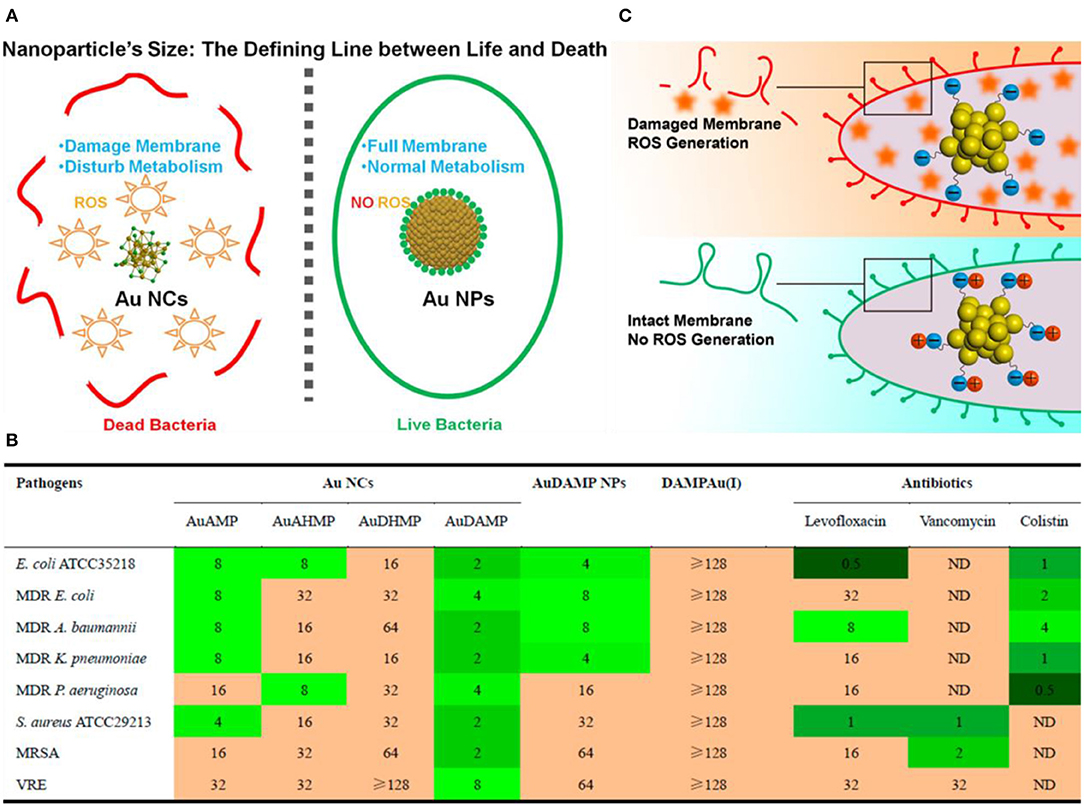
Figure 8. (A) Schematic illustration of the size regulation of Au NPs to significantly affect their antibacterial properties. Reproduced from Zheng K. et al. (2017) with permission from American Chemical Society. (B) Antibacterial activities of mercaptopyrimidine-conjugated Au NCs indicated with MIC (μg/mL). Here DAMPAu(I) is the precursor complex during the synthesis of Au NCs. Reproduced from Zheng et al. (2018c) with permission from American Chemical Society. (C) Surface ligand chemistry of gold nanoclusters determines their antimicrobial ability. Reproduced from Zheng et al. (2018a) with permission from American Chemical Society.
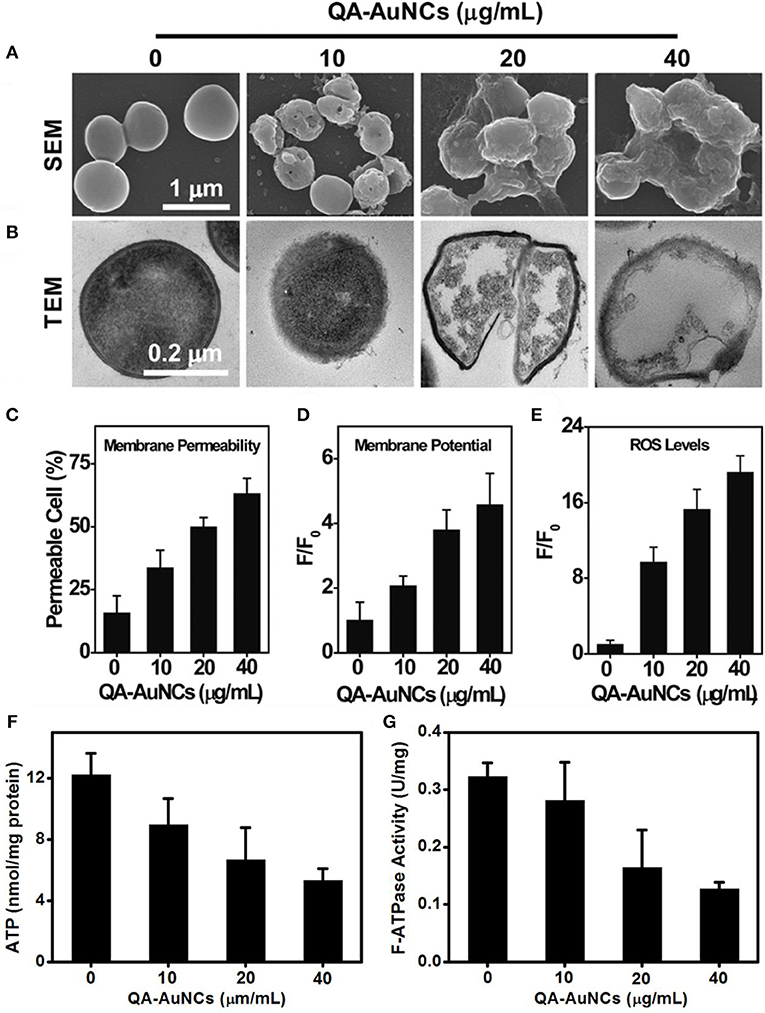
Figure 9. QA-Au NCs combat bacteria through a multipath mechanism. (A) Scanning electron microscopy (SEM) and (B) TEM images showing the morphological changes of S. aureus after treatment with QA-Au NCs. The administration of QA-Au NCs leads to an increase in the membrane permeability (C), a dissipation of the membrane potential (D) and the generation of ROS (E). The intracellular ATP level (F) and F-type ATPase activity (G) of S. aureus decrease upon treatment with increasing concentrations of QA-Au NCs. Reproduced from Xie et al. (2018) with permission from Wiley-VCH Verlag & Co. KGaA, Weinheim.
In addition to size effects, the surface ligand chemistry of Au NCs also profoundly affects their antibacterial properties. In general, antibacterial agents with positive surface charges are considered to lead higher antibacterial abilities (El Badawy et al., 2011; Chen W. Y. et al., 2015; Le Ouay and Stellacci, 2015; Zheng et al., 2018c). However, Zheng et al. obtained the opposite results when using Au25(SR)18 NCs (SR means thiolate ligands) (Zheng et al., 2018a). The molecular features and the surface properties of Au25(SR)18 NCs could be precisely tailored at the atomic level, producing a series of Au NCs with same Au atom numbers but different surface properties. By adjusting the type and ratio of surface ligands on Au25(SR)18, more negatively charged Au25(SR)18 would produce more ROS, leading to a better bacterial killing efficiency (Figure 8C). This unexpected result indicates the intricacies of the nano-bio interactions and may offer some inspiration on the design of high-performance Au NCs-based antibacterial drugs.
Macromolecule-Au NCs Systems
Macromolecules such as proteins, DNA, and dendrimers are also commonly used as the surface ligands attached to Au NCs for antibacterial treatments. Functionalization of macromolecules endowed potent antibacterial therapeutic capabilities on Au NCs. Chen et al. have prepared lysozyme-directed Au NCs as potential antibacterial agent for multidrug-resistant bacteria, including notorious pandrug resistant A. baumannii (Chen et al., 2010). Setyawati et al. have used DNA nanopyramid as the scaffold to intercalate red-emissive glutathione-capped Au NCs and actinomycin D to form an image-guided nanoantibiotics (DPAu/AMD) (Figure 10A) (Setyawati et al., 2014). The nanotheranostic agents of DPAu/AMD show a significant antibacterial efficiency and have been applied for the simultaneous diagnosis and treatment of E. coli and S. aureus infections. Furthermore, Liao and coworkers have constructed dendrimer-capped Au NCs that can effectively retard endotoxin activity to protect against sepsis (Liao et al., 2018). The retardant consists of an Au NC that acts as a flake-like substrate and a coating of short alkyl motifs that serve as an adhesive to dock with lipopolysaccharide by compacting the intramolecular hydrocarbon chain-chain distance of lipid A, which is an endotoxicity active site that can cause overwhelming cytokine induction resulting in sepsis progression (Figure 10B). The treatment of the antiendotoxin Au NCs prominently extended the survival time in lipopolysaccharide-induced septicemic mouse. This work might present a potential treatment for the early prophylaxis of septicemia. In addition, a photodynamic antibacterial treatment strategy based on Au NCs has also been developed through photosensitizer conjugated BSA-capped Au NCs (Khlebtsov et al., 2015).
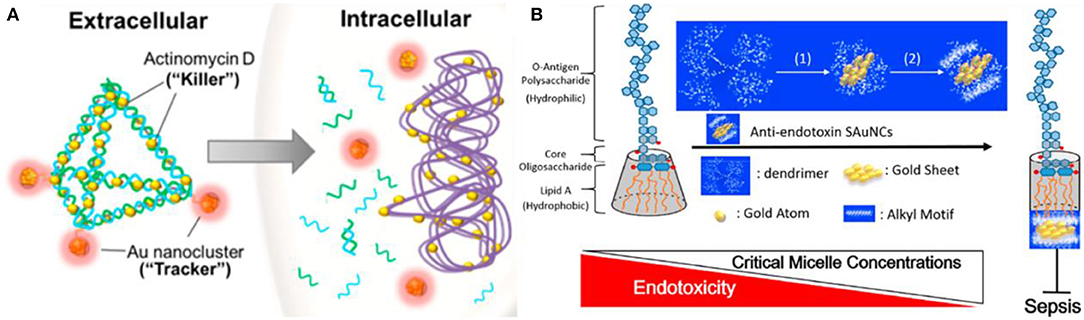
Figure 10. (A) Schematic illustration of DPAu/AMD as an image-guided nanotheranostic agent. Reproduced from Setyawati et al. (2014) with permission from American Chemical Society. (B) A simple model representing the possible correlation between the packing density of lipid A of lipopolysaccharide and sepsis progression in the presence of Au NCs. Reproduced from Liao et al. (2018) with permission from American Chemical Society.
Au NCs-Containing Combination Systems
In order to improve the antibacterial performances, antibacterial composites based on Au NCs have also been developed as nanoantibiotics. For instance, Zheng et al. established a synergistic antibacterial agent through assembly of paramagnetic Ho ions and Au NCs onto graphene oxide (GO) nanosheets (Zheng K. et al., 2019). GO is a new type of antibiotic substance combined with multiple mechanisms, and its rich functional groups enable the functionalization of nanomaterials to further ameliorate antimicrobial performances (Ji et al., 2016; Xia et al., 2019). The assembled nanostructures could be effectively piercing the bacteria. Meanwhile, the decorated Au NCs could efficiently induce bacteria to generate high-concentration ROS, severely interfere with bacterial metabolism, leading to the death of multidrug-resistant bacteria. TiO2/graphene/Au NC nanocomposites were also developed to ameliorate the antimicrobial capability of Au NCs under sunlight (Zhou et al., 2019). Conjugation of graphene and Au NCs into TiO2 NPs can dramatically improve the solar energy utilization efficiency and increase ROS levels, resulting in enhanced antibacterial activity. Alsaiari et al. designed and fabricated an intelligent antimicrobial mixed-matrix membrane coating comprising lysozyme-Au NCs and kanamycin as nanofillers (Alsaiari et al., 2017). The mixed-matrix coating can successfully treat healthcare-associated infections. In another study, protamine (Prot) functionalized Au NCs (Prot-Au NCs) with a highly stable ability to load positively charged antibacterial agents were developed, which may penetrate into the bacteria, thereby enhancing the ability to treat bacterial infections (Zhu et al., 2019). In addition, chitosan (CS)-induced antibacterial Au NCs nanoaggregates have also been found to significantly enhance antibacterial activity and facilitate rapid wound healing compared with their individual components (Figure 11) (Girija et al., 2019). Indeed, it appears that aggregation of nanomaterials can significantly improve their physicochemical properties and subsequently affect their therapeutic effects (Goswami et al., 2016; Qin et al., 2019). These investigations provide new options for improving the antibacterial properties of Au NCs.
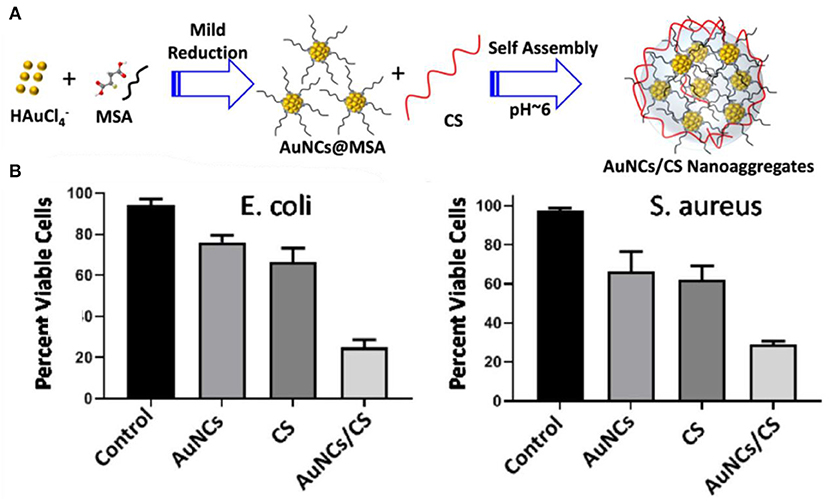
Figure 11. (A) Schematic representation of the formation of AuNCs/CS nanoaggregates. (B) The viability of E. coli and S. aureus post treatment with nanoaggregates. Reproduced from Girija et al. (2019) with permission from Wiley-VCH Verlag & Co. KGaA, Weinheim.
As a kind of innovative antibacterial nanomedicine, Au NCs have very attractive prospects in dealing with increasingly severe multidrug resistant infections. However, considering that the research of Au NCs as an antibacterial agent is in its infancy, there still remain several problems to be solved. Firstly, the synthesis of Au NCs with antibacterial activity at atomic precision is still a major challenge. Although the current research found that reducing the size of Au nanomaterials can make them have antibacterial capabilities, the structure of Au NCs is still not unique, which is a major obstacle to understanding the accurate antibacterial mechanism of Au NCs. Therefore, it is imperative to synthesize water-soluble Au NCs with a confirmed structure for the evaluation of antibacterial activity. Second, the general antibacterial mechanisms of Au NCs need further investigation. Although various mechanisms are proposed to explain the antibacterial property of Au NCs up to now, the metabolisms of Au NCs in bacterial cells are still needed for in-depth understanding their antibacterial activity by both experimental and theoretical studies. In addition, the possible development of bacterial resistance to Au NCs needs to be concerned. Although no reports of bacterial resistance to Au NCs have been reported, bacteria resistant to antibacterial Ag NPs have emerged (Panácek et al., 2018). Therefore, it is necessary to study the development of bacteria resistance to antibacterial Au NCs in a longer period by using genome-wide analysis. Finally, the biological safety of antibacterial Au NCs on animals, especially the effect on intestinal flora needs further exploration (Li J. et al., 2019; Wang L. et al., 2019).
Conclusions
In summary, we have attempted to present a review of the recent efforts on Au NCs from the multipath bacterial diagnostics and treatment. Due to the unique physicochemical properties, excellent biocompatibility, as well as advantages of easy surface functionalization of Au NCs, the recent mushrooming in fabrication and modification of Au NCs has empowered the exploitation of these nanomaterials for applications in selective detection of bacteria and infection treatment. In terms of bacterial detection, label-free detection strategies, specific molecular recognition strategies, and sensor arrays based on gold nanoclusters, have been established. In terms of bacterial infection treatment, Au NCs-based different antibacterial systems, including antibiotic-Au NCs systems, antimicrobial peptide-Au NCs systems, small molecule-Au NCs systems, macromolecule-Au NCs systems, and Au NCs-containing combination systems have been used for the treatment of multidrug-resistant bacterial infections. These studies reveal that ultrasmall Au NCs can offer promising opportunity in biomedicine to promote the mushrooming in this field. With the continuing development to unravel the structure-function relationships, we believe that the ultrasmall Au NCs will eventually serve as an important platform for bacterial detection and infection treatment.
Author Contributions
MT and YZ performed literature search as well as the majority of the authoring and editing. JZ and CY performed the literature arrangement and writing for future perspectives. HJ proposed topic of paper and provided overall direction of manuscript.
Funding
This work was supported by the National Natural Science Foundation of China (21675023 and 21974019) and the Scientific Research Foundation of Southwest Medical University (2019ZZD018 and 2019ZQN104).
Conflict of Interest
The authors declare that the research was conducted in the absence of any commercial or financial relationships that could be construed as a potential conflict of interest.
References
Alsaiari, S. K., Hammami, M. A., Croissant, J. G., Omar, H. W., Neelakanda, P., Yapici, T., et al. (2017). Colloidal gold nanoclusters spiked silica fillers in mixed matrix coatings: simultaneous detection and inhibition of healthcare-associated infections. Adv. Healthcare Mater. 6:1601135. doi: 10.1002/adhm.201601135
Blair, J. M., Webber, M. A., Baylay, A. J., Ogbolu, D. O., and Piddock, L. J. (2015). Molecular mechanisms of antibiotic resistance. Nat. Rev. Microbiol. 13, 42–51. doi: 10.1038/nrmicro3380
Boda, S. K., Broda, J., Schiefer, F., Weber-Heynemann, J., Hoss, M., Simon, U., et al. (2015). Cytotoxicity of ultrasmall gold nanoparticles on planktonic and biofilm encapsulated gram-positive staphylococci. Small 11, 3183–3193. doi: 10.1002/smll.201403014
Boucher, H. W., Talbot, G. H., Bradley, J. S., Edwards, J. E., Gilbert, D., Rice, L. B., et al. (2009). Bad bugs, no drugs: no ESKAPE! An update from the infectious diseases society of America. Clin. Infect. Dis. 48, 1–12. doi: 10.1086/595011
Chan, P. H., and Chen, Y. C. (2012). Human serum albumin stabilized gold nanoclusters as selective luminescent probes for Staphylococcus aureus and methicillin-resistant Staphylococcus aureus. Anal. Chem. 84, 8952–8956. doi: 10.1021/ac302417k
Chan, P. H., Ghosh, B., Lai, H. Z., Peng, H. L., Mong, K. K. T., and Chen, Y. C. (2013). Photoluminescent gold nanoclusters as sensing probes for uropathogenic Escherichia coli. PLoS ONE 8:e58064. doi: 10.1371/journal.pone.0058064
Chang, T. K., Cheng, T. M., Chu, H. L., Tan, S. H., Kuo, J. C., Hsu, P. H., et al. (2019). Metabolic mechanism investigation of antibacterial active cysteine-conjugated gold nanoclusters in Escherichia coli. ACS Sustain. Chem. Eng. 7, 15479–15486. doi: 10.1021/acssuschemeng.9b03048
Chen, L. Y., Wang, C. W., Yuan, Z., and Chang, H. T. (2015). Fluorescent gold nanoclusters: recent advances in sensing and imaging. Anal. Chem. 87, 216–229. doi: 10.1021/ac503636j
Chen, W. Y., Chang, H. Y., Lu, J. K., Huang, Y. C., Harroun, S. G., Tseng, Y. T., et al. (2015). Self-assembly of antimicrobial peptides on gold nanodots: against multidrug-resistant bacteria and wound-healing application. Adv. Funct. Mater. 25, 7189–7199. doi: 10.1002/adfm.201503248
Chen, W. Y., Lin, J. Y., Chen, W. J., Luo, L., Wei-Guang Diau, E., and Chen, Y. C. (2010). Functional gold nanoclusters as antimicrobial agents for antibiotic-resistant bacteria. Nanomedicine 5, 755–764. doi: 10.2217/nnm.10.43
Chen, Y., Li, L., Gong, L., Zhou, T., and Liu, J. (2019). Surface regulation towards stimuli-responsive luminescence of ultrasmall thiolated gold nanoparticles for ratiometric imaging. Adv. Funct. Mater. 29:1806945. doi: 10.1002/adfm.201806945
Cheng, C., Yang, L., Zhong, M., Deng, W., Tan, Y., Xie, Q., et al. (2018). Au nanocluster-embedded chitosan nanocapsules as labels for the ultrasensitive fluorescence immunoassay of Escherichia coli O157: H7. Analyst 143, 4067–4073. doi: 10.1039/C8AN00987B
Cheng, D., Yu, M., Fu, F., Han, W., Li, G., Xie, J., et al. (2016). Dual recognition strategy for specific and sensitive detection of bacteria using aptamer-coated magnetic beads and antibiotic-capped gold nanoclusters. Anal. Chem. 88, 820–825. doi: 10.1021/acs.analchem.5b03320
Chung, H. J., Reiner, T., Budin, G., Min, C., Liong, M., Issadore, D., et al. (2011). Ubiquitous detection of gram-positive bacteria with bioorthogonal magnetofluorescent nanoparticles. ACS Nano 5, 8834–8841. doi: 10.1021/nn2029692
Connor, E. E., Mwamuka, J., Gole, A., Murphy, C. J., and Wyatt, M. D. (2005). Gold nanoparticles are taken up by human cells but do not cause acute cytotoxicity. Small 1, 325–327. doi: 10.1002/smll.200400093
Disney, M. D., Zheng, J., Swager, T. M., and Seeberger, P. H. (2004). Detection of bacteria with carbohydrate-functionalized fluorescent polymers. J. Am. Chem. Soc. 126, 13343–13346. doi: 10.1021/ja047936i
El Badawy, A. M., Silva, R. G., Morris, B., Scheckel, K. G., Suidan, M. T., and Tolaymat, T. M. (2011). Surface charge-dependent toxicity of silver nanoparticles. Environ. Sci. Technol. 45, 283–287. doi: 10.1021/es1034188
Girija, A. R., Balasubramanian, S., Bright, R., Cowin, A. J., Goswami, N., and Vasilev, K. (2019). Ultrasmall gold nanocluster based antibacterial nanoaggregates for infectious wound healing. ChemNanoMat. 5, 1176–1181. doi: 10.1002/cnma.201900366
Goswami, N., Lin, F., Liu, Y., Leong, D. T., and Xie, J. (2016). Highly luminescent thiolated gold nanoclusters impregnated in nanogel. Chem. Mater. 28, 4009–4016. doi: 10.1021/acs.chemmater.6b01431
Gupta, A., Mumtaz, S., Li, C. H., Hussain, I., and Rotello, V. M. (2019). Combatting antibiotic-resistant bacteria using nanomaterials. Chem. Soc. Rev. 48, 415–427. doi: 10.1039/C7CS00748E
Hammer, B., and Norskov, J. (1995). Why gold is the noblest of all the metals. Nature 376, 238–240. doi: 10.1038/376238a0
Hancock, R. E., and Sahl, H. G. (2006). Antimicrobial and host-defense peptides as new anti-infective therapeutic strategies. Nat. Biotechnol. 24, 1551–1557. doi: 10.1038/nbt1267
Harris, S. L., Spears, P. A., Havell, E. A., Hamrick, T. S., Horton, J. R., and Orndorff, P. E. (2001). Characterization of Escherichia coli type 1 pilus mutants with altered binding specificities. J. Bacteriol. 183, 4099–4102. doi: 10.1128/JB.183.13.4099-4102.2001
Hassan, M., Kjos, M., Nes, I. F., Diep, D. B., and Lotfipour, F. (2012). Natural antimicrobial peptides from bacteria: characteristics and potential applications to fight against antibiotic resistance. J. Appl. Microbiol. 113, 723–736. doi: 10.1111/j.1365-2672.2012.05338.x
Hilchie, A. L., Wuerth, K., and Hancock, R. E. (2013). Immune modulation by multifaceted cationic host defense (antimicrobial) peptides. Nat. Chem. Biol. 9, 761–768. doi: 10.1038/nchembio.1393
Hossein-Nejad-Ariani, H., Kim, T., and Kaur, K. (2018). Peptide-based biosensor utilizing fluorescent gold nanoclusters for detection of Listeria monocytogenes. ACS Appl. Nano Mater. 1, 3389–3397. doi: 10.1021/acsanm.8b00600
Hu, X., Zheng, Y., Zhou, J., Fang, D., Jiang, H., and Wang, X. (2018). Silver-assisted thiolate ligand exchange induced photoluminescent boost of gold nanoclusters for selective imaging of intracellular glutathione. Chem. Mater. 30, 1947–1955. doi: 10.1021/acs.chemmater.7b04926
Huang, C. C., Chen, C. T., Shiang, Y. C., Lin, Z. H., and Chang, H. T. (2009). Synthesis of fluorescent carbohydrate-protected Au nanodots for detection of concanavalin A and Escherichia coli. Anal. Chem. 81, 875–882. doi: 10.1021/ac8010654
Huang, Y., Ren, J., and Qu, X. (2019). Nanozymes: classification, catalytic mechanisms, activity regulation, and applications. Chem. Rev. 119, 4357–4412. doi: 10.1021/acs.chemrev.8b00672
Huh, A. J., and Kwon, Y. J. (2011). “Nanoantibiotics”: a new paradigm for treating infectious diseases using nanomaterials in the antibiotics resistant era. J. Control. Release 156, 128–145. doi: 10.1016/j.jconrel.2011.07.002
Ji, H., Sun, H., and Qu, X. (2016). Antibacterial applications of graphene-based nanomaterials: recent achievements and challenges. Adv. Drug Deliver. Rev. 105, 176–189. doi: 10.1016/j.addr.2016.04.009
Ji, H., Wu, L., Pu, F., Ren, J., and Qu, X. (2018). Point-of-care identification of bacteria using protein-encapsulated gold nanoclusters. Adv. Healthcare Mater. 7:1701370. doi: 10.1002/adhm.201701370
Jin, R., Zeng, C., Zhou, M., and Chen, Y. (2016). Atomically precise colloidal metal nanoclusters and nanoparticles: fundamentals and opportunities. Chem. Rev. 116, 10346–10413. doi: 10.1021/acs.chemrev.5b00703
Kalita, S., Kandimalla, R., Bhowal, A. C., Kotoky, J., and Kundu, S. (2018). Functionalization of β-lactam antibiotic on lysozyme capped gold nanoclusters retrogress MRSA and its persisters following awakening. Sci. Rep. 8:5778. doi: 10.1038/s41598-018-22736-5
Khandelwal, P., Singh, D. K., Sadhu, S., and Poddar, P. (2015). Study of the nucleation and growth of antibiotic labeled Au NPs and blue luminescent Au8 quantum clusters for Hg2+ ion sensing, cellular imaging and antibacterial applications. Nanoscale 7, 19985–20002. doi: 10.1039/C5NR05619E
Khlebtsov, B., Tuchina, E., Tuchin, V., and Khlebtsov, N. (2015). Multifunctional Au nanoclusters for targeted bioimaging and enhanced photodynamic inactivation of Staphylococcus aureus. RSC Adv. 5, 61639–61649. doi: 10.1039/C5RA11713E
Kulagina, N. V., Lassman, M. E., Ligler, F. S., and Taitt, C. R. (2005). Antimicrobial peptides for detection of bacteria in biosensor assays. Anal. Chem. 77, 6504–6508. doi: 10.1021/ac050639r
Lazcka, O., Del Campo, F. J., and Munoz, F. X. (2007). Pathogen detection: a perspective of traditional methods and biosensors. Biosens. Bioelectron. 22, 1205–1217. doi: 10.1016/j.bios.2006.06.036
Le Ouay, B., and Stellacci, F. (2015). Antibacterial activity of silver nanoparticles: a surface science insight. Nano Today 10, 339–354. doi: 10.1016/j.nantod.2015.04.002
Lewinski, N., Colvin, V., and Drezek, R. (2008). Cytotoxicity of nanoparticles. Small 4, 26–49. doi: 10.1002/smll.200700595
Li, D., Kumari, B., Makabenta, J. M., Gupta, A., and Rotello, V. (2019). Effective detection of bacteria using metal nanoclusters. Nanoscale 11, 22172–22181. doi: 10.1039/C9NR08510F
Li, J., Cha, R., Zhao, X., Guo, H., Luo, H., Wang, M., et al. (2019). Gold nanoparticles cure bacterial infection with benefit to intestinal microflora. ACS Nano 13, 5002–5014. doi: 10.1021/acsnano.9b01002
Li, Q., Pan, Y., Chen, T., Du, Y., Ge, H., Zhang, B., et al. (2018). Design and mechanistic study of a novel gold nanocluster-based drug delivery system. Nanoscale 10, 10166–10172. doi: 10.1039/C8NR02189A
Li, X., Bai, H., Yang, Y., Yoon, J., Wang, S., and Zhang, X. (2019). Supramolecular antibacterial materials for combatting antibiotic resistance. Adv. Mater. 31:1805092. doi: 10.1002/adma.201805092
Li, Z., Tang, S., Wang, B., Li, Y., Huang, H., Wang, H., et al. (2016). Metabolizable small gold nanorods: size-dependent cytotoxicity, cell uptake and in vivo biodistribution. ACS Biomater. Sci. Eng. 2, 789–797. doi: 10.1021/acsbiomaterials.5b00538
Liang, G., Jin, X., Zhang, S., and Xing, D. (2017). RGD peptide-modified fluorescent gold nanoclusters as highly efficient tumor-targeted radiotherapy sensitizers. Biomaterials 144, 95–104. doi: 10.1016/j.biomaterials.2017.08.017
Liang, J., Xiong, H., Wang, W., Wen, W., Zhang, X., and Wang, S. (2018). “Luminescent-off/on” sensing mechanism of antibiotic-capped gold nanoclusters to phosphate-containing metabolites and its antibacterial characteristics. Sens. Actuat. B Chem. 255, 2170–2178. doi: 10.1016/j.snb.2017.09.032
Liao, F. H., Wu, T. H., Huang, Y. T., Lin, W. J., Su, C. J., Jeng, U. S., et al. (2018). Subnanometer gold clusters adhere to lipid A for protection against endotoxin-induced sepsis. Nano Lett. 18, 2864–2869. doi: 10.1021/acs.nanolett.7b05464
Liu, J., Lu, L., Xu, S., and Wang, L. (2015). One-pot synthesis of gold nanoclusters with bright red fluorescence and good biorecognition abilities for visualization fluorescence enhancement detection of E. coli. Talanta 134, 54–59. doi: 10.1016/j.talanta.2014.10.058
Liu, P., Wang, H., Hiltunen, J. K., Chen, Z., and Shen, J. (2015). Cross-linked proteins with gold nanoclusters: a dual-purpose pH-responsive material for controllable cell imaging and antibiotic delivery. Part. Part. Syst. Charact. 32, 749–755. doi: 10.1002/ppsc.201400243
Luo, Z., Zheng, K., and Xie, J. (2014). Engineering ultrasmall water-soluble gold and silver nanoclusters for biomedical applications. Chem. Commun. 50, 5143–5155. doi: 10.1039/C3CC47512C
Mahlapuu, M., Håkansson, J., Ringstad, L., and Björn, C. (2016). Antimicrobial peptides: an emerging category of therapeutic agents. Front. Cell. Infect. Microbiol. 6:194. doi: 10.3389/fcimb.2016.00194
Marrache, S., and Dhar, S. (2012). Engineering of blended nanoparticle platform for delivery of mitochondria-acting therapeutics. Proc. Natl. Acad. Sci. U.S.A. 109, 16288–16293. doi: 10.1073/pnas.1210096109
Mukherji, R., Samanta, A., Illathvalappil, R., Chowdhury, S., Prabhune, A., and Devi, R. N. (2013). Selective imaging of quorum sensing receptors in bacteria using fluorescent Au nanocluster probes surface functionalized with signal molecules. ACS Appl. Mater. Interfaces 5, 13076–13081. doi: 10.1021/am404093m
Palestro, C. J., and Love, C. (2009). Nuclear medicine and diabetic foot infections. Semin. Nucl. Med. 39, 52–65. doi: 10.1053/j.semnuclmed.2008.08.006
Pan, Y., Neuss, S., Leifert, A., Fischler, M., Wen, F., Simon, U., et al. (2007). Size-dependent cytotoxicity of gold nanoparticles. Small 3, 1941–1949. doi: 10.1002/smll.200700378
Panácek, A., Kvítek, L., Smékalová, M., Vecerová, R., Kolár, M., and Röded Zboril, R. (2018). Bacterial resistance to silver nanoparticles and how to overcome it. Nat. Nanotechnol. 13, 65–71. doi: 10.1038/s41565-017-0013-y
Pranantyo, D., Liu, P., Zhong, W., Kang, E. T., and Chan-Park, M. B. (2019). Antimicrobial peptide-reduced gold nanoclusters with charge-reversal moieties for bacterial targeting and imaging. Biomacromolecules 20, 2922–2933. doi: 10.1021/acs.biomac.9b00392
Qin, Z., Du, T., Zheng, Y., Luo, P., Zhang, J., Xie, M., et al. (2019). Glutathione induced transformation of partially hollow gold-silver nanocages for cancer diagnosis and photothermal therapy. Small 15:1902755. doi: 10.1002/smll.201902755
Rajchakit, U., and Sarojini, V. (2017). Recent developments in antimicrobial-peptide-conjugated gold nanoparticles. Bioconjugate Chem. 28, 2673–2686. doi: 10.1021/acs.bioconjchem.7b00368
Ray, P. C., Khan, S. A., Singh, A. K., Senapati, D., and Fan, Z. (2012). Nanomaterials for targeted detection and photothermal killing of bacteria. Chem. Soc. Rev. 41, 3193–3209. doi: 10.1039/c2cs15340h
Setyawati, M. I., Kutty, R. V., Tay, C. Y., Yuan, X., Xie, J., and Leong, D. T. (2014). Novel theranostic DNA nanoscaffolds for the simultaneous detection and killing of Escherichia coli and Staphylococcus aureus. ACS Appl. Mater. Interfaces 6, 21822–21831. doi: 10.1021/am502591c
Song, X. R., Goswami, N., Yang, H. H., and Xie, J. (2016). Functionalization of metal nanoclusters for biomedical applications. Analyst 141, 3126–3140. doi: 10.1039/C6AN00773B
Soto, G. E., and Hultgren, S. J. (1999). Bacterial adhesins: common themes and variations in architecture and assembly. J. Bacteriol. 181, 1059–1071. doi: 10.1128/JB.181.4.1059-1071.1999
Sun, Z., Zheng, W., Zhu, G., Lian, J., Wang, J., Hui, P., et al. (2019). Albumin broadens the antibacterial capabilities of non-antibiotic small molecule-capped gold nanoparticles. ACS Appl. Mater. Interfaces 11, 45381–45389. doi: 10.1021/acsami.9b15107
Tseng, Y. T., Chang, H. T., Chen, C. T., Chen, C. H., and Huang, C. C. (2011). Preparation of highly luminescent mannose-gold nanodots for detection and inhibition of growth of Escherichia coli. Biosens. Bioelectron. 27, 95–100. doi: 10.1016/j.bios.2011.06.021
Vocadlo, D. J., Davies, G. J., Laine, R., and Withers, S. G. (2001). Catalysis by hen egg-white lysozyme proceeds via a covalent intermediate. Nature 412, 835–838. doi: 10.1038/35090602
Wang, L., Zhang, C., Zhi, X., Hou, W., Li, T., Pan, S., et al. (2019). Impact of short-term exposure of AuNCs on the gut microbiota of BALB/c mice. J. Biomed. Nanotechnol. 15, 779–789. doi: 10.1166/jbn.2019.2731
Wang, Q., Wei, H., Zhang, Z., Wang, E., and Dong, S. (2018). Nanozyme: an emerging alternative to natural enzyme for biosensing and immunoassay. TrAC Trends Anal. Chem. 105, 218–224. doi: 10.1016/j.trac.2018.05.012
Wang, S., Wang, Y., Peng, Y., and Yang, X. (2019). Exploring the antibacteria performance of multicolor Ag, Au, and Cu nanoclusters. ACS Appl. Mater. Interfaces 11, 8461–8469. doi: 10.1021/acsami.8b22143
Wu, Y., Wang, B., Wang, K., and Yan, P. (2018). Identification of proteins and bacteria based on a metal ion-gold nanocluster sensor array. Anal. Methods 10, 3939–3944. doi: 10.1039/C8AY00558C
Xavier, P. L., Chaudhari, K., Verma, P. K., Pal, S. K., and Pradeep, T. (2010). Luminescent quantum clusters of gold in transferrin family protein, lactoferrin exhibiting FRET. Nanoscale 2, 2769–2776. doi: 10.1039/c0nr00377h
Xia, M. Y., Xie, Y., Yu, C. H., Chen, G. Y., Li, Y. H., Zhang, T., et al. (2019). Graphene-based nanomaterials: the promising active agents for antibiotics-independent antibacterial applications. J. Control. Release 307, 16–31. doi: 10.1016/j.jconrel.2019.06.011
Xie, X., Tan, F., Xu, A., Deng, K., Zeng, Y., and Huang, H. (2019). UV-induced peroxidase-like activity of gold nanoclusters for differentiating pathogenic bacteria and detection of enterotoxin with colorimetric readout. Sens. Actuat. Chem. 279, 289–297. doi: 10.1016/j.snb.2018.10.019
Xie, Y., Liu, Y., Yang, J., Liu, Y., Hu, F., Zhu, K., et al. (2018). Gold nanoclusters for targeting methicillin-resistant Staphylococcus aureus in vivo. Angew. Chem. Int. Ed. 57, 3958–3962. doi: 10.1002/anie.201712878
Xing, B., Yu, C. W., Chow, K. H., Ho, P. L., Fu, D., and Xu, B. (2002). Hydrophobic interaction and hydrogen bonding cooperatively confer a vancomycin hydrogel: a potential candidate for biomaterials. J. Am. Chem. Soc. 124, 14846–14847. doi: 10.1021/ja028539f
Yan, R., Shou, Z., Chen, J., Wu, H., Zhao, Y., Qiu, L., et al. (2018). On-off-on gold nanocluster-based fluorescent probe for rapid Escherichia coli differentiation, detection and bactericide screening. ACS Sustain. Chem. Eng. 6, 4504–4509. doi: 10.1021/acssuschemeng.8b00112
Yang, J. Y., Yang, T., Wang, X. Y., Wang, Y. T., Liu, M. X., Chen, M. L., et al. (2019). A novel three-dimensional nanosensing array for the discrimination of sulfur-containing species and sulfur bacteria. Anal. Chem. 91, 6012–6018. doi: 10.1021/acs.analchem.9b00476
Yang, X., Yang, M., Pang, B., Vara, M., and Xia, Y. (2015). Gold nanomaterials at work in biomedicine. Chem. Rev. 115, 10410–10488. doi: 10.1021/acs.chemrev.5b00193
Yu, M., Wang, H., Fu, F., Li, L., Li, J., Li, G., et al. (2017). Dual-recognition förster resonance energy transfer based platform for one-step sensitive detection of pathogenic bacteria using fluorescent vancomycin-gold nanoclusters and aptamer-gold nanoparticles. Anal. Chem. 89, 4085–4090. doi: 10.1021/acs.analchem.6b04958
Yu, M., Xu, J., and Zheng, J. (2019). Renal clearable luminescent gold nanoparticles: from the bench to the clinic. Angew. Chem. Int. Ed. 58, 4112–4128. doi: 10.1002/anie.201807847
Yuan, H., Liu, Z., Liu, L., Lv, F., Wang, Y., and Wang, S. (2014). Cationic conjugated polymers for discrimination of microbial pathogens. Adv. Mater. 26, 4333–4338. doi: 10.1002/adma.201400636
Yuan, P., Ding, X., Yang, Y. Y., and Xu, Q. H. (2018). Metal nanoparticles for diagnosis and therapy of bacterial infection. Adv. Healthcare Mater. 7:1701392. doi: 10.1002/adhm.201701392
Zhang, J., Chen, Y. P., Miller, K. P., Ganewatta, M. S., Bam, M., Yan, Y., et al. (2014). Antimicrobial metallopolymers and their bioconjugates with conventional antibiotics against multidrug-resistant bacteria. J. Am. Chem. Soc. 136, 4873–4876. doi: 10.1021/ja5011338
Zhang, L., and Wang, E. (2014). Metal nanoclusters: new fluorescent probes for sensors and bioimaging. Nano Today 9, 132–157. doi: 10.1016/j.nantod.2014.02.010
Zhang, X. D., Wu, D., Shen, X., Liu, P. X., Fan, F. Y., and Fan, S. J. (2012). In vivo renal clearance, biodistribution, toxicity of gold nanoclusters. Biomaterials 33, 4628–4638. doi: 10.1016/j.biomaterials.2012.03.020
Zheng, K., Li, K., Chang, T. H., Xie, J., and Chen, P. Y. (2019). Synergistic antimicrobial capability of magnetically oriented graphene oxide conjugated with gold nanoclusters. Adv. Funct. Mater. 29:1904603. doi: 10.1002/adfm.201904603
Zheng, K., Setyawati, M. I., Leong, D. T., and Xie, J. (2017). Antimicrobial gold nanoclusters. ACS Nano 11, 6904–6910. doi: 10.1021/acsnano.7b02035
Zheng, K., Setyawati, M. I., Leong, D. T., and Xie, J. (2018a). Surface ligand chemistry of gold nanoclusters determines their antimicrobial ability. Chem. Mater. 30, 2800–2808. doi: 10.1021/acs.chemmater.8b00667
Zheng, Y., Lai, L., Liu, W., Jiang, H., and Wang, X. (2017). Recent advances in biomedical applications of fluorescent gold nanoclusters. Adv. Colloid Interface Sci. 242, 1–16. doi: 10.1016/j.cis.2017.02.005
Zheng, Y., Liu, W., Chen, Y., Jiang, H., and Wang, X. (2018b). Mercaptopyrimidine-directed gold nanoclusters: a suitable fluorescent probe for intracellular glutathione imaging and selective cancer cell identification. J. Mater. Chem. B 6, 3650–3654. doi: 10.1039/C8TB00791H
Zheng, Y., Liu, W., Chen, Y., Li, C., Jiang, H., and Wang, X. (2019). Conjugating gold nanoclusters and antimicrobial peptides: from aggregation-induced emission to antibacterial synergy. J. Colloid Interface Sci. 546, 1–10. doi: 10.1016/j.jcis.2019.03.052
Zheng, Y., Liu, W., Qin, Z., Chen, Y., Jiang, H., and Wang, X. (2018c). Mercaptopyrimidine conjugated gold nanoclusters as nanoantibiotics for combating multidrug-resistant superbugs. Bioconjugate Chem. 29, 3094–3103. doi: 10.1021/acs.bioconjchem.8b00452
Zheng, Y., Wang, X., and Jiang, H. (2018d). Label-free detection of Acinetobacter baumannii through the induced fluorescence quenching of thiolated AuAg nanoclusters. Sens. Actuat. Chem. 277, 388–393. doi: 10.1016/j.snb.2018.09.028
Zhou, T., Cheng, Y., Zhang, H., and Wang, G. (2019). Sunlight-mediated antibacterial activity enhancement of gold nanoclusters and graphene co-decorated titanium dioxide nanocomposites. J. Cluster Sci. 30, 985–994. doi: 10.1007/s10876-019-01558-z
Keywords: gold nanoclusters, photoluminescence, bacteria detection, antibacterial activity, multidrug-resistant bacteria
Citation: Tang M, Zhang J, Yang C, Zheng Y and Jiang H (2020) Gold Nanoclusters for Bacterial Detection and Infection Therapy. Front. Chem. 8:181. doi: 10.3389/fchem.2020.00181
Received: 03 January 2020; Accepted: 26 February 2020;
Published: 24 March 2020.
Edited by:
Chen Zhou, University of Central Missouri, United StatesReviewed by:
Zheng Lu, Pfizer, United StatesShasha Sun, Jiangsu University of Science and Technology, China
Copyright © 2020 Tang, Zhang, Yang, Zheng and Jiang. This is an open-access article distributed under the terms of the Creative Commons Attribution License (CC BY). The use, distribution or reproduction in other forums is permitted, provided the original author(s) and the copyright owner(s) are credited and that the original publication in this journal is cited, in accordance with accepted academic practice. No use, distribution or reproduction is permitted which does not comply with these terms.
*Correspondence: Youkun Zheng, enlrdG14JiN4MDAwNDA7c3dtdS5lZHUuY24=; Hui Jiang, c3VuZ2kmI3gwMDA0MDtzZXUuZWR1LmNu