- 1School of Materials Science and Engineering, Jiangsu Collaborative Innovation Center of Photovoltaic Science and Engineering, Changzhou University, Changzhou, China
- 2Hunan Province Cooperative Innovation Center for the Construction & Development of Dongting Lake Ecological Economic Zone, College of Chemistry and Materials Engineering, Hunan University of Arts and Science, Changde, China
Silicon oxides (SiOx) have been considered to be the likeliest material to substitute graphite anode for lithium-ion batteries (LIBs) due to its high theoretical capacity, appropriate working potential plus rich abundance. Nevertheless, the two inherent disadvantages of volume expansion and low electrical conductivity of SiOx have been a main obstacle to its application. Here, SiOx-G/PAA-PANi/graphene composite has been successfully synthesized by in-situ polymerization, in which SiOx-G particles linked together by a graphene-doped polyacrylic acid-polyaniline conductive flexible hydrogel and SiOx-G is encapsulated inside the conductive hydrogel. We demonstrate that SiOx-G/PAA-PANi/graphene composite possesses a discharge-specific capacity of 842.3 mA h g−1 at a current density of 500 mA g−1 after a cycle life of 100 cycles, and a good initial coulombic efficiency (ICE) of 74.77%. The superior performance probably due to the lithium ion transmission rate and the electric conductivity enhanced by the three-dimensional (3D) structured conductive polymer hydrogel.
Introduction
Lithium-ion batteries (LIBs), presumably among the most prospective devices for energy storage, are featured with higher energy density, longer cycle life, lower self-discharging, and more safety. Developing advanced LIBs with highly advanced energy density and cyclability is an immediate need for lightweight electronics and range expansion of electric vehicles. Yet the current graphite anode with an unsatisfactory specific capacity of ~372 mA h g−1 (LiC6) can′t follow the development of modern equipment for high energy storage system (Casimir et al., 2016; Zuo et al., 2017; Han et al., 2018; Yi et al., 2018, 2019; Zheng et al., 2018, 2020; Xiao et al., 2019). Hence, silicon has become a potential candidate to replace commercial graphite anode for LIBs in that it has higher capacity (~4,200 mA h g−1), suitable discharge platform (~0.4 V vs. Li/Li+) and sufficient resources (Casimir et al., 2016; Jiang et al., 2016; Xu et al., 2018; An et al., 2019; Liu Y. et al., 2019a; Yang et al., 2019; Zhou et al., 2019; Zuo et al., 2019). Nevertheless, the two stubborn disadvantages of silicon, including the deterioration of electrode structure integrity as a result of gradual enhancement of pulverization happening in the repetition of discharge/charge process, as well as poor conductivity, have been the main obstacles to its application (Zhao et al., 2016; Zuo et al., 2017). To address the above-mentioned key issues, a series of countermeasures have been taken, such as optimizing structure, doping and coating with carbon or other conductive materials.
Compared with Si-based materials, SiOx-based anodes are prone to achieve remarkable electrochemical performance due to the formation of Li2O and Li silicates, which can form the stable solid electrolyte interphase (SEI) layer and adapt to the volume expansion of SiOx during the insertion of Li+ (Nguyen et al., 2013; Xu et al., 2017; Liu D. et al., 2019; Liu Y. et al., 2019b; Zheng et al., 2019). Although the capacity of SiOx is high, the volume multiplication usually causes this material to crack and pulverize. Besides, the low intrinsic conductivity of SiOx would lead to poor rate performance (Xu et al., 2017; Xiao et al., 2018; Fang et al., 2019; Li et al., 2019; Wang et al., 2020). Different ways have been adopted to overcome these issues, including doping the host framework with conductive particle, coating the electrodes with buffer materials, bettering the morphology, cutting the size of dimension and applying more effective binders. For example, Yu et al. obtained the pomegranate-liked nano-scale SiOx-C by spray drying, the prepared composite presents a discharge specific capacity of 1,024 mA h g−1 when the current density is 500 mA g−1 after 200 cycles (Yu et al., 2018). Jiang et al. taken advantage of graphene bubbles to encapsulate the SiOx inside to accelerate ion transmission rate of the material, achieving 80% capacity retention after 1,000 cycles (Jiang et al., 2016).
Conducting polymers (CPs), such as polyaniline (PANi), polyacetylene, polythiophene (PTh), Polypyrrole (PPy), and et al., providing special 3D network nanostructures and high conductivity as a result of their large conjugated π bonds structure (Li et al., 2009; Li J. et al., 2018; Li P. et al., 2018). At the same time, CPs have been verified as significant materials for the advancement of modern society, consisting of energy storage, semiconductor sensors and catalysis. For example, a PANi/CNT composite electrode synthesized via the way of in-situ chemical polymerization of aniline in a well-dispersed CNT solution revealed excellent electrochemical behavior. As the cathode material for LIBs, the PANi/CNT possessed a high energy density of 86 mA h g−1 at the 80th cycle and an average coulombic efficiency of 98% (Li et al., 2009). Also, SiOx-PANi–Ag composite electrodes were synthesized by Zhang et al. via in-situ polymerization, and exhibited great cycling performances (with a reversible capacity of 1,149 mA h g−1 after 100 cycles) (Wang et al., 2014). Wu et al. prepared SiNP-PANi with a capacity retention rate of more than 90% after 5,000 cycles at a current density of 6.0 A g−1 (Wu et al., 2013).
Besides, the carbon bonds of graphene are sp2 hybridized, exhibiting a number of intriguing and unique properties such as high surface area, admirable electronic conductivity and superior mechanical properties (Huang et al., 2011; Li P. et al., 2018). It is very meaningful that the properties make graphene-based materials useful for modifying silicon-based materials (Jiang et al., 2016). For example, Zhu et al. constructed Si@SiOx/GH composite with a stable storage capacity of 1,020 mA h g−1 at 4 A g−1 (Zhu et al., 2019).
Herein, we provide a flexible and harmless method for synthesizing SiOx-G/PAA-PANi/graphene (Scheme 1). Firstly, SiOx-G was synthesized by a method of high-energy mechanical ball milling (Jiang et al., 2016). Then, Polyacrylic acid and Polyaniline are doped with each other by in-situ polymerization as a framework to synthesize SiOx-G/PAA-PANi. Finally, the graphene dispersion is added to obtain the SiOx-G/PAA-PANi/graphene. This intertwined doping not only can provide a continuous path for electron conduction, but also stabilize the material structure. Hence, the SiOx-G/PAA-PANi/graphene exhibits superior electrochemical performance.
Experimental
Material Preparation
Synthesis of SiOx-G
SiOx-G was obtained by a solid state method. Firstly, SiOx is prepared by ball milling (QM-3SP4, Nanjing, China) SiO in Ar for 6 h (500 rpm, the ball-to-particulate weight ratio of 30:1). Secondly, 6.0 g of Graphite was heated at 600°C for 20 min in Ar flow and then mixed with 6.0 g of SiOx by ball milling for 6 h under the same ball milling conditions as the process for preparation of SiOx. The above composite material is recorded as SiOx-G.
Synthesis of SiOx-G/PAA-PANi
Firstly, 0.005 g of PAA was weighed and dissolved in a 25 ml beaker and placed in an oven at 60°C for 1 h, and then an appropriate amount of 0.5 M NaOH was added. Secondly, 0.2 g of SiOx-G was dissolved in the above sodium polyacrylate solution, and ultrasonicated for 1 h, followed by stirring in an ice bath (Li P. et al., 2018). Subsequently, aniline and (NH4)2S2O8 were added and stirred for 40 min. The target production was obtained after standing, dialysis, and lyophilization.
Synthesis of SiOx-G/PAA-PANi/Graphene
The preparation steps are the same as the synthesis process of SiOx-G/PAA-PANi, except that the dispersion of 0.002 g of graphene is stirred for 1 h before standing. The obtained sample was named as SiOx-G/PAA-PANi/graphene.
Material Characterization
The as-prepared product was characteristic of X-ray diffraction (XRD, D/max 2500 PC) with the use of Cu Ka radiation. X-ray photoelectron spectroscopy (XPS) data was recorded by the Electronic detection system (Thermo VG Scientific ESCA Lab 250). Thermogravimetric analysis (TGA) data is recorded from indoor temperature to 800°C at a rate of 10°C min−1 under an oxygen atmosphere. The data analysis of Fourier transform infrared (FT-IR) spectroscopy was undertaken by an IR spectrophotometer (Thermo Fisher, American). The morphology and element distribution are presented by high-resolution field emission scanning electron microscopy (FESEM, Zeiss, Germany). Transmission electron microscopy (TEM) were performed on a JEOL 2100 worked at 200 kV.
Electrochemical Measurements
In preparation for the working electrode, the active material (75%), the conductive agent (Acetylene black,10%) and the binder (Sodium alginate,15%) were uniformly mixed to obtain the slurry, and coated on the upper part of the current collector (copper foil) and placed in a vacuum oven at 105°C for 8 h. CR2032-type coin half-cell was converged in a glove box filled with high purity argon (O2 and H2O < 0.5 ppm), with 1 M LiPF6 dissolved in ethylene carbonate: dimethyl carbonate: ethyl methyl carbonate (1:1:1 in volume), and 10% of fluorinated ethylene carbonate was further added as the electrolyte, Celgard 2500 film as the separator, and lithium foil as the counter electrode. The cyclic stability test and rate performance test of the material can be achieved on Land-CT 2001A instrument where the potential is 0.01 V−3 V and the current density is 500 mA g−1 at 25°C. Cyclic Voltammetry (CV) was recorded by electrochemical analyzer (CHI 604E, Chen He Instruments, Shanghai).
Results and Discussion
XRD patterns of four samples were compared in Figure 1A. As illustrated, commercial silicon monoxide is amorphous, which is consistent with previous findings (Hwa et al., 2013; Yu et al., 2013). Compared with SiO, the position of the broad peak of SiOx shifted from 22° to 24.8°. Also, it is observed that there is a distinct characteristic peak of Si (111) located at 27.2° in as-obtained SiOx sample, which might be due to that a part of SiO is reduced to Si after ball milling (Jiang et al., 2016). In addition, two peaks at 43.4° and 44.5° were observed in SiOx samples, corresponding to the characteristic peak of Fe2Si, which might be created from the stainless steel ball and Si during the process of ball milling. Similar phenomenon was also demonstrated by Qian et al. (2017). Figure 1B shows the XRD pattern of graphite. The crystal plane characteristic peak of graphite (002) is situated at 26.5° in SiOx-G, SiOx-G/PAA-PANi and SiOx-G/PAA-PANi/graphene samples (Figure 1A), showing that the existence of graphite in the three samples.
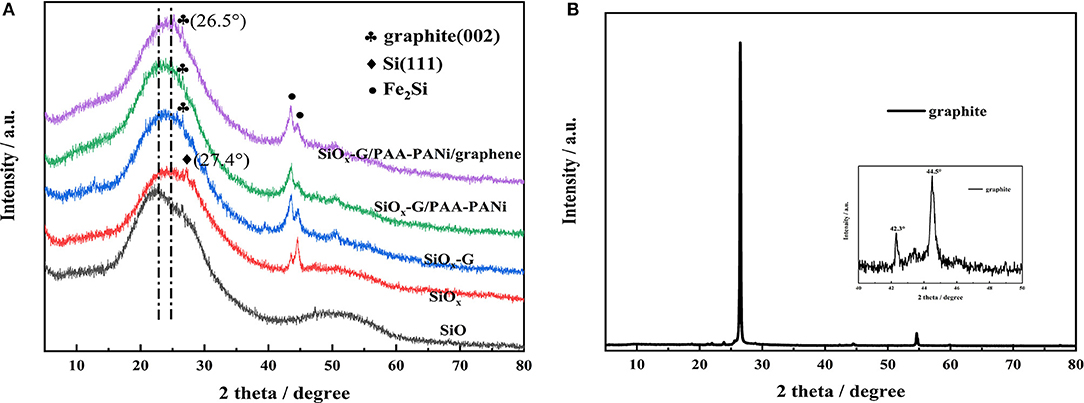
Figure 1. (A) XRD image of SiO, SiOx, SiOx-G, SiOx-G/PAA-PANi, and SiOx-G/PAA-PANi/graphene; (B) XRD image of graphite.
Fourier transform infrared (FT-IR) spectrum of SiOx-G/PAA-PANi/graphene is shown in Figure 2. The absorption peaks at 3,430 and 2,920 cm−1 correspond to the N-H bending vibration absorption peak and -CH2- stretching vibration absorption peak, respectively. In addition, the bands at 1,720 and 1,090 cm−1 are attributed to C=O bending vibration and C-H bending vibration. The presence of above several absorption peaks are sufficient to prove the existence of PAA (Wang et al., 2015). The weaker absorption peak from 1,400 to 1,650 cm−1 belongs to the polyaniline. The two characteristic bands at 1,490 and 1,580 cm−1 are attributed to the stretching vibration of benzenoid C=C and the stretching vibration of quinonoid C=C, respectively. The band at 1,630 cm−1 can be assigned to N-H bending vibration (Li et al., 1999; Sivakkumar and Kim, 2007). The results confirm that the in-suit polymerization of PAA and aniline lead to the formation of PAA-PANi.
The element details of SiOx-G/PAA-PANi/graphene were reflected by XPS. Figure 3 shows the spectrum of all elements (Figure 3A), Si2p (Figure 3B), C1s (Figure 3C), and N1s (Figure 3D). The detail information about valence states of Si obtained from Figure 3B and described in Table 1. Clearly, the existence of Si0(99.98 eV), Si1+(102.06eV), Si2+(102.85eV), Si3+(103.68eV), and Si4+(105.00eV) can be observed in the SiOx-G/PAA-PANi/graphene sample, and the corresponding atomic percentages are 15.55, 20.11, 29.63, 29.63, and 5.08%, respectively (Zheng et al., 2018). The average valence of Si calculated from the Si 2p spectrum is 1.88. The XPS results of Figure 3B verifies the successful synthesis of SiOx from the commercial SiO. The three peaks (Sp2-bonded C, C-O and C-N) deconvoluted from the C1s (Figure 3C) spectrum are situated at 286.75 eV, and C=O located at 288.8 eV. The bonding energy of C1s for graphite is located at 284.8 eV, indicating that graphite is present in SiOx-G/PAA-PANi/graphene. It can be obtained from the N1s spectrum (Figure 3D) that there is a strong peak at 400.00 eV, being in correspondence with the characteristic chemical N-H of PANi. The protonated amines are located at 402.20 and 403.70 eV. N1s spectrum (Figure 3D) and FT-IR (Figure 2) images further confirmed the successful preparation of PAA-PANi by in-situ polymerization.
Figures 4a,b shows the SEM images of SiOx-G/PAA-PANi/graphene. As clearly seen, graphene is distributed around SiOx-G/PAA-PANi, which plays a supporting connection in the structure and can improve the conductivity. This results match with the results shown by the TEM (Figures 4c,d). Figure 4c shows the TEM image of SiOx-G/PAA-PANi. As obtained from Figure 4a, the SiOx-G particles are encapsulated inside the conductive hydrogel, and the coating thickness range of PAA-PANi ranges from 100 nm to 200 nm. Figure 4d depicts the TEM image of SiOx-G/PAA-PANi/graphene. It can be observed that SiOx-G/PAA-PANi particles are distributed between graphene.
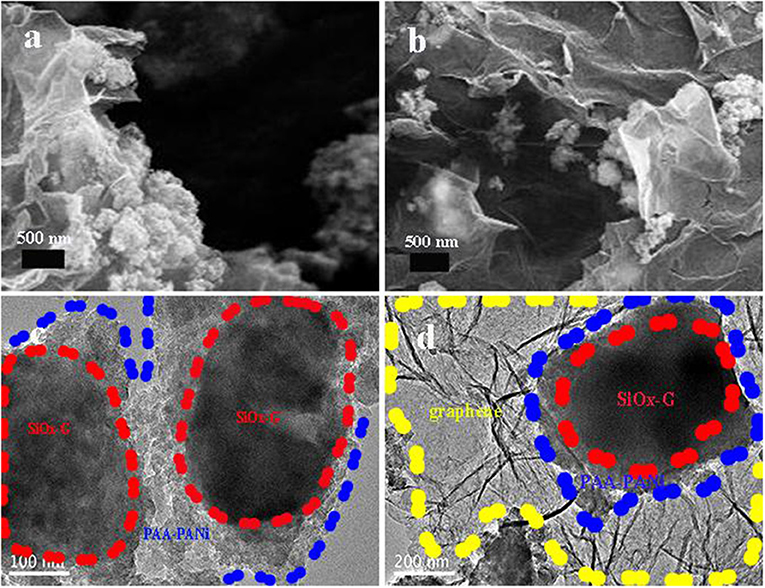
Figure 4. (a,b) SEM images of SiOx-G/PAA-PANi/graphene; (c) TEM image of SiOx-G/PAA-PANi; (d) TEM image of SiOx-G/PAA-PANi/graphene.
The estimate of the carbon contents based on the three samples was obtained via TG measurement. The carbon contents of SiOx-G, SiOx-G/PAA-PANi, and SiOx-G/PAA-PANi/graphene were about 46.85, 55.22, and 56.08 wt%, respectively (Figure 5). The superficial passivation of SiOx lead to the oxidation of Si in air was not distinguished between 600 and 800°C (Chen et al., 2014; He et al., 2019; Jiang Y. et al., 2019; Jiang Z. et al., 2019). They are beneficial to improve the cycle performance of SiO to varying degrees.
Figure 6 displays the charge/discharge curves for SiOx-G/PAA-PANi/graphene, SiOx-G/PAA-PANi and SiO at a current density of 100 mA g−1. The charge/discharge curves of the SiO electrode deliver a high initial discharge specific capacity of 2,156 mA h g−1 and an initial charge specific capacity of 1,165 mA h g−1 (ICE is 54%) and show a stable and obvious voltage platform around 0.1 V, in which the gentle slopes appears between 0.2 and 0.5 V. The first discharge-charge profiles of the SiOx-G/PAA-PANi and SiOx-G/PAA-PANi/graphene electrodes are similar to that of the Si-SiOx-Cristobalite/Graphite electrode (Ren and Li, 2014). The first discharge and charge capacities of SiOx-G/PAA-PANi composite are 1006.4 and 692.3 mA h g−1, respectively, with an ICE of about 68.8%. The capacity of the first discharge and charge capacity of SiOx-G/PAA-PANi/graphene is 1420.8 and 1062.3 mA h g−1, respectively, with an ICE of about 74.77%. The large initial irreversible capacity is ascribed to the Li+ consumption of to form the SEI film as well as the chemical reactions between Li and SiOx.
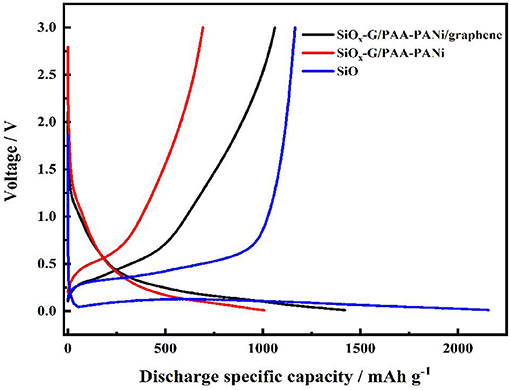
Figure 6. The charge/discharge curves of SiOx-G/PAA-PANi/graphene, SiOx-G/PAA-PANi, and SiO anodes under the current density of 500 mA g−1.
Figure 7A displays the cyclic voltammetry curves of the SiOx-G/PAA-PANi. A relatively flat reduction peak appearing at 1.2 V and a strong reduction peak appearing at 0.65 V in the first lithiation process are corresponding to the decomposition of liquid electrolytes and formation of the SEI film, respectively (Ren and Li, 2014). The oxidation peak appearing at 0.18 V indicates that Li is detached from LixC, and the voltage position representing Li detached from LixSi is at 0.6 V in the first delithiation (Sivakkumar and Kim, 2007; Chen et al., 2014, 2018; Li et al., 2019). The reason for the incomplete reversibility of the electrode capacity is attributed to the by-products (Li2O and Li2Si2O5) formed by the irreversible chemical reactions between SiOx and Li (Sivakkumar and Kim, 2007). There are two reduction peaks around 0.6 V and 0.2 V, which represent the lithiation process. Figure 7B shows the cyclic voltammetry curves of the SiOx-G/PAA-PANi/graphene composite. Comparing with the CV curves of the SiOx-G/PAA-PANi, there are the same reduction peaks around 0.6 and 0.2 V in the first cycle of SiOx-G/PAA-PANi/graphene, which are attributed to lithium extraction from the SiOx-G/PAA-PANi/graphene.
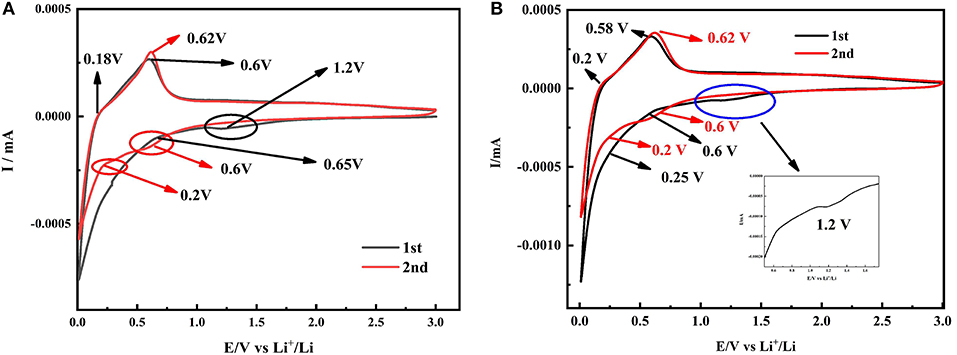
Figure 7. CV curves of SiOx-G/PAA-PANi (A) and SiOx-G/PAA-PANi/graphene (B) electrodes at the scanning rate of 0.1 mV s−1.
The rate performance of the SiOx-G/PAA-PANi/graphene, SiOx-G/PAA-PANi, and SiO electrodes is illustrated in Figure 8. The rate performance of the SiO electrode is significantly different from that of the SiOx-G/PAA-PANi electrode, in which the rate performance of the SiO electrode is so unsatisfactory. Based on these results, the doping of conductive graphene further enhances the rate performance. The capacities of the SiOx-G/PAA-PANi/graphene are 1022.3, 828.1, 675.7, 588.1, 556.2 mA h g−1 at a current density of 100, 200, 400, 800, and 1,000 mA g−1, respectively. Moreover, the capacity can rise to 967.1 mA h g−1 as the current density returns to 100 mA g−1, which demonstrates that SiOx-G/PAA-PANi/graphene electrode can maintain a good structural stability.
Figure 9 indicates the cycling stability of SiOx-G/PAA-PANi/graphene, SiOx-G/PAA-PANi, and SiO electrodes at a current density of 500 mA g−1. Although the initial discharge specific capacity of the SiO electrode reaches 1916.6 mA h g−1, the capacity suddenly drops due to the rupture of the SiO structure. Compared with SiO, the cycle performance of SiOx-G/PAA-PANi is significantly improved, and a high discharge specific capacity (685.4 mA h g−1) is maintained when it is cycled to the 100th cycle, which can be attributed to the 3D structure of the conductive hydrogel that can serve as an effective buffer for the volume change of SiOx nanoparticles. In contrast to SiOx-G/PAA-PANi, SiOx-G/PAA-PANi/graphene exhibits a significantly improved performance, with a discharge specific capacity of 842.3 mA h g−1 at current density of 500 mA g−1 at 100th cycle, and the coulombic efficiency is about 99% from 8th to 100th cycles, which is due to the improved electron transport.
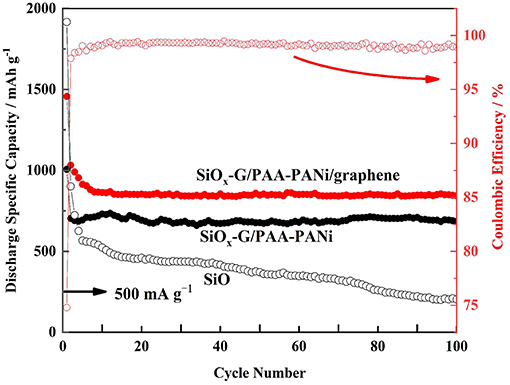
Figure 9. Long-time cycling performances (discharge capacities) of SiOx-G/PAA-PANi/graphene, SiOx-G/PAA-PANi, and SiO under the current density of 500 mA g−1.
Conclusions
In summary, the three-dimensional SiOx-G/PAA-PANi/graphene hydrogel was prepared by a facile ball milling and in-situ polymerization process. The amorphous SiOx-G was encapsulated within 3D mesh structure of PAA-PANi/graphene. According to the preparation method of the study, the specific capacity of SiOx-G/PAA-PANi/graphene can be as high as 842.3 mA h g−1 at 500 mA g−1 when Li+ are subjected to the 100th deintercalation, as well as the ICE is increased to 74.77% compared with SiO. The superior performance could be due to that PAA-PANi offers fast channels for electronic and ionic transfer and free space for SiOx-G volume changes, and also the conductively active graphene effectively improves the electron transport.
Data Availability Statement
The raw data supporting the conclusions of this article will be made available by the authors, without undue reservation, to any qualified researcher.
Author Contributions
YR contributed conception, design of the study, and revised the manuscript. YL carried out experiments and wrote the manuscript. KL performed analyzed experimental results. XH revised the manuscript.
Funding
This work was financially supported by the National Nature Science Foundation of China (21576030 and U1607127).
Conflict of Interest
The authors declare that the research was conducted in the absence of any commercial or financial relationships that could be construed as a potential conflict of interest.
References
An, C., Yuan, Y., Zhang, B., Tang, L., Xiao, B., He, Z., et al. (2019). Graphene wrapped FeSe2 nano-microspheres with high pseudocapacitive contribution for enhanced Na-ion storage. Adv. Energy Mater. 9:1900356. doi: 10.1002/aenm.201900356
Casimir, A., Zhang, H., Ogoke, O., Amine, J., Lu, J., and Wu, G. (2016). Silicon-based anodes for lithium-ion batteries: effectiveness of materials synthesis and electrode preparation. Nano Energy 27, 359–376. doi: 10.1016/j.nanoen.2016.07.023
Chen, D., Yi, R., Chen, S., Xu, T., Gordin, M., and Wang, D. (2014). Facile synthesis of graphene–silicon nanocomposites with an advanced binder for high-performance lithium-ion battery anodes. Solid State Ionics. 254, 65–71. doi: 10.1016/j.ssi.2013.11.020
Chen, Y., Mao, Q., Bao, L., Yang, T., Lu, X., Du, N., et al. (2018). Rational design of coaxial MWCNTs@Si/SiOx@C nanocomposites as extending-life anode materials for lithium-ion batteries. Ceram. Int. 44, 16660–16667. doi: 10.1016/j.ceramint.2018.06.093
Fang, R., Miao, C., Mou, H., and Xiao, W. (2019). Facile synthesis of Si@TiO2@rGO composite with sandwich-like nanostructure as superior performance anodes for lithium ion batteries. J. Alloys. Compd. 818:152884. doi: 10.1016/j.jallcom.2019.152884
Han, X., Cui, X., Yi, T., Li, Y., and Yue, C. (2018). Recent progress of NiCo2O4-based anodes for high-performance lithium-ion batteries. Curr. Opin. Solid State Mater Sci. 22, 109–126. doi: 10.1016/j.cossms.2018.05.005
He, X., Sun, Z., Zou, Q., Yang, J., and Wu, L. (2019). Codeposition of nanocrystalline Co-Ni catalyst based on 1-ethyl-3-methylimidazolium bisulfate and ethylene glycol system for hydrogen evolution reaction. J. Electrochem. Soc. 166, D908–D915. doi: 10.1149/2.0171916jes
Huang, X., Yin, Z., Wu, S., Qi, X., He, Q., Zhang, Q., et al. (2011). Graphene-based materials: synthesis, characterization, properties, and applications. Small 7, 1876–1902. doi: 10.1002/smll.201002009
Hwa, Y., Park, C., and Sohn, H. (2013). Modified SiO as a high performance anode for Li-ion batteries. J. Power Sour. 22, 129–134. doi: 10.1016/j.jpowsour.2012.08.060
Jiang, B., Zeng, S., Wang, H., Liu, D., Qian, J., Cao, Y., et al. (2016). Dual core–shell structured Si@SiOx@C nanocomposite synthesized via a one-step pyrolysis method as a highly stable anode material for lithium-ion batteries. Appl. Mater. Interfaces 8, 31611–31616. doi: 10.1021/acsami.6b09775
Jiang, Y., Feng, X., Cheng, G., Li, Y., Li, C., He, Z., et al. (2019). Electrocatalytic activity of MnO2 nanosheet array-decorated carbon paper as superior negative electrode for vanadium redox flflow Batteries. Electrochim. Acta. 322:134754. doi: 10.1016/j.electacta.2019.134754
Jiang, Z., Li, Y., Zhu, J., Li, B., Li, C., Wang, L., et al. (2019). Synthesis and performance of a graphene decorated NaTi2(PO4)3/C anode for aqueous lithium-ion batteries. Alloys J. Compd. 791, 176–183. doi: 10.1016/j.jallcom.2019.03.289
Li, C., Bai, H., and Shi, G. (2009). Conducting polymer nanomaterials: electrosynthesis and applications. Chem. Soc. Rev. 38, 2397–2409. doi: 10.1039/b816681c
Li, D., Jiang, Y., Li, C., Wu, Z., Chen, X., and Li, Y. (1999). Self-assembly of polyaniline/polyacrylic acid films via acid–base reaction induced deposition. Polymer 40, 7065–7070. doi: 10.1016/S0032-3861(99)00118-4
Li, J., Zhang, G., Yang, Y., Yao, D., Lei, Z., Li, S., et al. (2018). Glycinamide modified polyacrylic acid as high-performance binder for silicon anodes in lithium-ion batteries. Power Sour. J. 406, 102–109. doi: 10.1016/j.jpowsour.2018.10.057
Li, P., Jin, Z., Peng, L., Zhao, F., Xiao, D., Jin, Y., et al. (2018). Stretchable all-gel-state fiber-shaped supercapacitors enabled by macromolecularly interconnected 3D graphene/nanostructured conductive polymer hydrogels. Adv. Mater. 20181800124. doi: 10.1002/adma.201800124
Li, R., Xiao, W., Miao, C., Fang, R., Wang, Z., and Zhang, M. (2019). Sphere-like SnO2/TiO2 composites as high-performance anodes for lithium ion batteries. Ceram. Int. 45, 13530–13535. doi: 10.1016/j.ceramint.2019.04.059
Liu, D., Fan, X., Li, Z., Liu, T., Ling, M., Liu, Y., et al. (2019). A cation/anion co-doped Li1.12Na0.08Ni0.2Mn0.6O1.95F0.05 cathode for lithium ion batteries. Nano Energy 58, 786–796. doi: 10.1016/j.nanoen.2019.01.080
Liu, Y., Fan, X., Zhang, Z., Wu, H., Liu, D., Dou, A., et al. (2019b). Enhanced electrochemical performance of Li-rich layered cathode materials by combined Cr doping and LiAlO2 coating. ACS Sustainable Chem. Eng. 7, 2225–2235. doi: 10.1021/acssuschemeng.8b04905
Liu, Y., Tang, L., Wei, H., Zhang, X., He, Z., Li, Y., et al. (2019a). Enhancement on structural stability of Ni-Rich cathode materials by in-situ fabricating dual-modified layer for lithium-ion batteries. Nano Energy 65:104043. doi: 10.1016/j.nanoen.2019.104043
Nguyen, D., Nguyen, C., Kim, J., Kim, J., and Song, S. (2013). Facile synthesis and high anode performance of carbon fiber-interwoven amorphous nano-SiOx/graphene for rechargeable lithium batteries. ACS Appl. Mater. Interfaces 5, 11234–11239. doi: 10.1021/am4034763
Qian, L., Lan, J., Xue, M., Yu, Y., and Yang, X. (2017). Two-step ball-milling synthesis of a Si/SiOx/C composite electrode for lithium ion batteries with excellent long-term cycling stability. RSC Adv. 7, 36697–36704. doi: 10.1039/C7RA06671F
Ren, Y., and Li, M. (2014). Si-SiOx-cristobalite/graphite composite as anode for Li-ion batteries. Electrochim. Acta. 142, 11–17. doi: 10.1016/j.electacta.2014.07.101
Sivakkumar, S., and Kim, D. (2007). Polyaniline/carbon nanotube composite cathode for rechargeable lithium polymer batteries assembled with gel polymer electrolyte. J. Electrochem. Soc. 54:A134. doi: 10.1149/1.2404901
Wang, K., Huang, X., Zhou, T., Wang, H., Xie, H., and Ren, Y. (2020). Boosted electrochemical properties of porous Li2FeSiO4/C based on Fe-MOFs precursor for lithium ion batteries. Vacuum. 171:108997. doi: 10.1016/j.vacuum.2019.108997
Wang, L., Zhang, P., Su, L., and Ma, C. (2014). SiOx-PANI-Ag composites with Homogeneously-embedded Si nanocrystals and nanopores as high-performance anodes for lithium ion batteries. J. Mater. Chem. A 2, 3776–3782. doi: 10.1039/c3ta14498d
Wang, Y., Wang, X., Tang, S., and Vongehr, S. (2015). Highly processible and electrochemically active graphene-doped polyacrylic acid/polyaniline allowing the preparation of defect-free thin films for solid-state supercapacitors. RSC Adv. 5, 62670–62677. doi: 10.1039/C5RA05486A
Wu, H., Yu, G., Pan, L., Liu, N., McDowell, M., Bao, Z., et al. (2013). Stable Li-ion battery anodes by in-situ polymerization of conducting hydrogel to conformally coat silicon nanoparticles. Nat. Commun. 4:1943. doi: 10.1038/ncomms2941
Xiao, B., Wang, P., Zhang, B., He, Z., Yang, Z., Tang, L., et al. (2019). Effect of MgO and TiO2 coating on electrochemical performance of Li-Rich cathode materials for lithium-ion batteries. Energy Technol. 7:1800829. doi: 10.1002/ente.201800829
Xiao, W., Wang, Z., Zhang, Y., Fang, R., Yuan, Z., Miao, C., et al. (2018). Enhanced performance of P(VDF-HFP)-based composite polymer electrolytes doped with organic-inorganic hybrid particles PMMA-ZrO2 for lithium ion batteries. J. Power Sour. 382, 128–134. doi: 10.1016/j.jpowsour.2018.02.012
Xu, Q., Sun, J., Yin, Y., and Yu, G. (2017). Facile synthesis of blocky SiOx/C with graphite-like structure for high-performance lithium-ion battery anodes. Adv. Funct. Mater.28:1705235. doi: 10.1002/adfm.201705235
Xu, Q., Sun, J., Yu, Z., Yin, Y., Xin, S., Yu, S., et al. (2018). SiOx encapsulated in graphene bubble film: an ultrastable Li-on battery anode. Adv. Mater. 30:1707430. doi: 10.1002/adma.201707430
Yang, S., Wang, P., Wei, H., Tang, L., Zhang, X., He, Z., et al. (2019). Li4V2Mn(PO4)4-stablized Li[Li0.2Mn0.54Ni0.13Co0.13]O2 cathode materials for lithium ion batteries. Nano Energy 63:103889. doi: 10.1016/j.nanoen.2019.103889
Yi, T., Peng, P., Fang, Z., Zhu, Y., Xie, Y., and Luo, S. (2019). Carbon-coated LiMn1−xFexPO4 (0 ≤ x ≤ 0.5) nanocomposites as high-performance cathode materials for Li-ion battery. Compos. Part B. 175:107067. doi: 10.1016/j.compositesb.2019.107067
Yi, T., Zhu, Y., Tao, W., Luo, S., Xie, Y., and Li, X. (2018). Recent advances in the research of MLi2Ti6O14 (M=2Na, Sr, Ba, Pb) anode materials for Li-ion batteries. J. Power Sour. 339, 26–41. doi: 10.1016/j.jpowsour.2018.07.086
Yu, B., Hwa, Y., Park, C., and Sohn, H. (2013). Reaction mechanism and enhancement of cyclability of SiO anodes by surface etching with NaOH for Li-ion batteries. J. Mater. Chem. A. 1:4820. doi: 10.1039/c3ta00045a
Yu, Q., Ge, P., Liu, Z., Xu, M., Yang, W., Zhou, L., et al. (2018). Ultrafine SiOx/C nanospheres and their pomegranate-like assemblies for high performance lithium storage. J. Mater. Chem. A 10:1039. doi: 10.1039/C8TA03987A
Zhao, L., Dvorak, D., and Obrovac, M. (2016). Layered amorphous silicon as negative electrodes in lithium-ion batteries. J. Power Sour. 332, 290–298. doi: 10.1016/j.jpowsour.2016.09.124
Zheng, J., Yang, Z., He, Z., Tong, H., Yu, W., and Zhang, J. (2018). In situ formed LiNi0.8Co0.15Al0.05O2@Li4SiO4 composite cathode material with high rate capability and long cycling stability for lithium-ion batteries. Nano Energy 53, 613–621. doi: 10.1016/j.nanoen.2018.09.014
Zheng, S., Dou, A., Su, M., and Liu, Y. (2020). Influence of Nb doping on electrochemical performance of nanostructured cation disordered Li1+x/100Ni1/2−x/100Ti1/2−x/100Nbx/100O2 composites cathode for Li-ion batteries. J. Nanosci. Nanotechnol. 20, 452–459. doi: 10.1166/jnn.2020.16884
Zheng, S., Liu, D., Tao, L., Fan, X., Liu, K., Liang, G., et al. (2019). Electrochemistry and redox characterization of rock-salt-type lithium metal oxides Li1+z/3Ni1/2−z/2Ti1/2+z/6O2 for Li-ion batteries. Alloys J. Compd. 773, 1–10. doi: 10.1016/j.jallcom.2018.09.261
Zhou, C., Wang, P., Zhang, B., Tang, L., Tong, H., He, Z., et al. (2019). Formation and effect of residual lithium compounds on Li-rich cathode material Li1.35[Ni0.35Mn0.65]O2. ACS Appl. Mater. Interfaces 11, 11518–11523. doi: 10.1021/acsami.9b01806
Zhu, L., Du, F., Zhuang, Y., Dai, H., Cao, H., Adkins, J., et al. (2019). Effect of crosslinking binders on Li-storage behavior of silicon particles as anodes for lithium ion batteries. J. Electroanal. Chem. 845, 22–30. doi: 10.1016/j.jelechem.2019.05.019
Zuo, D., Song, S., An, C., Tang, L., He, Z., and Zheng, J. (2019). Synthesis of sandwich-likestructured Sn/SnOx@MXene composite through in-situ growth for highly reversible lithium storage. Nano Energy 62, 401–409. doi: 10.1016/j.nanoen.2019.05.062
Keywords: lithium-ion batteries, anode material, SiOx, PAA-PANi, graphene, conductive hydrogel
Citation: Liao Y, Liang K, Ren Y and Huang X (2020) Fabrication of SiOx-G/PAA-PANi/Graphene Composite With Special Cross-Doped Conductive Hydrogels as Anode Materials for Lithium Ion Batteries. Front. Chem. 8:96. doi: 10.3389/fchem.2020.00096
Received: 29 October 2019; Accepted: 31 January 2020;
Published: 21 February 2020.
Edited by:
Zhangxing He, North China University of Science and Technology, ChinaReviewed by:
Mingyong Wang, University of Science and Technology Beijing, ChinaXifei Li, Xi'an University of Technology, China
Copyright © 2020 Liao, Liang, Ren and Huang. This is an open-access article distributed under the terms of the Creative Commons Attribution License (CC BY). The use, distribution or reproduction in other forums is permitted, provided the original author(s) and the copyright owner(s) are credited and that the original publication in this journal is cited, in accordance with accepted academic practice. No use, distribution or reproduction is permitted which does not comply with these terms.
*Correspondence: Yurong Ren, cnlyY2hlbSYjeDAwMDQwOzE2My5jb20=