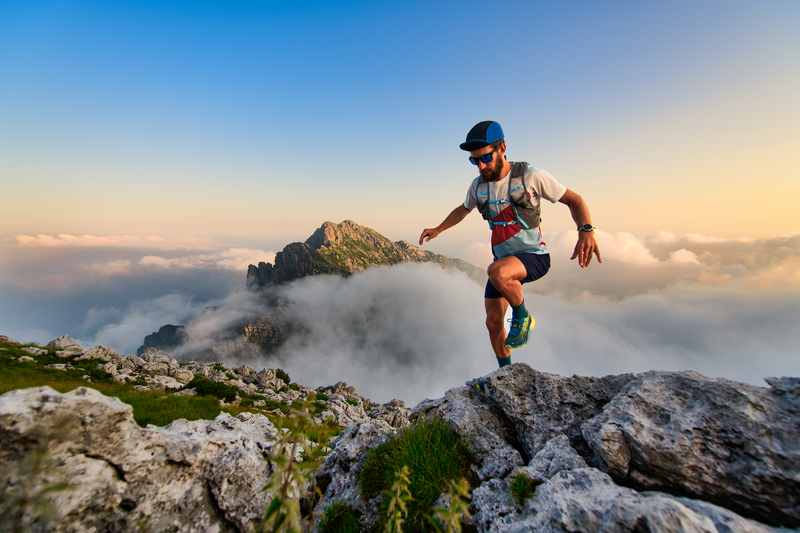
94% of researchers rate our articles as excellent or good
Learn more about the work of our research integrity team to safeguard the quality of each article we publish.
Find out more
ORIGINAL RESEARCH article
Front. Chem. , 18 February 2020
Sec. Nanoscience
Volume 7 - 2019 | https://doi.org/10.3389/fchem.2019.00941
This article is part of the Research Topic Functionalized Inorganic Semiconductor Nanomaterials: Characterization, Properties, and Applications View all 18 articles
Energy crises and environmental pollution are two serious threats to modern society. To overcome these problems, graphitic carbon nitride (g-C3N4) nanosheets were fabricated and functionalized with SnO2 nanoparticles to produce H2 from water splitting and degrade 2-chlorophenol under visible light irradiation. The fabricated samples showed enhanced photocatalytic activities for both H2 evolution and pollutant degradation as compared to bare g-C3N4 and SnO2. These enhanced photoactivities are attributed to the fast charge separation as the excited electrons transfer from g-C3N4 to the conduction band of SnO2. This enhanced charge separation has been confirmed by the photoluminescence spectra, steady state surface photovoltage spectroscopic measurement, and formed hydroxyl radicals. It is believed that this work will provide a feasible route to synthesize photocatalysts for improved energy production and environmental purification.
Exhaustion of hydrocarbon fuels and addition of toxic and hazardous materials from agricultural, medicinal, dyes, and cosmetic industries to the environment have resulted in increased pressure on the scientific community to address these problems adequately. A number of methods have been chalked out such as cracking of hydrocarbons and thermal splitting of water at elevated temperature to get H2 (future fuel). However, these methods require highly costly and controlled operational environment and huge labor under normal conditions. On the other hand, different pollutants removal technologies such as adsorption, coagulation, and electrochemical methods have their own shortcomings and did not receive much popularity in the purification of the environment (Zhao et al., 2015; Gautam et al., 2016; Li et al., 2016; Ali et al., 2018b; Wang et al., 2018; Ali S. et al., 2019). Therefore, modern techniques are urgently required to address energy and environmental issues properly with the least operational cost and time.
Photocatalysis has opened a brilliant chapter to realize the energy crises and environmental issues. The photocatalysts have shown their remarkable influence in the production of H2 from water, production of hydrocarbon fuels from CO2, and removal of pollutants from air and wastewater with minimum cost and least working labor (Singh et al., 2017; Li et al., 2018a,b; Qu et al., 2018; Ullah et al., 2019; Xu et al., 2019a). Although different photocatalysts such as TiO2, ZnO, and ZrO2 have been widely utilized, there are some typical problems such as activeness only under ultraviolet (UV) light and fast recombination of photogenerated charges (Raizada et al., 2017; Qi et al., 2018a,b, 2020a). Since visible light contributes a major portion to electromagnetic radiations, therefore, photocatalysts active under visible light are more effective and efficient. Graphitic carbon nitride, g-C3N4, is a promising polymeric photocatalyst with a band gap of 2.7 eV. Its conduction band (CB) and valence band (VB) have characteristic abilities to reduce water and oxidize organic pollutants, respectively (Qi et al., 2019a,b). Its metal-free nature is of particular importance as its release to the environment does not produce harmful threats to the aquatic animals and plants (Nie et al., 2018; Ran et al., 2018; Fu et al., 2019; Liu M. et al., 2019). However, low surface area and poor excited charge separation capability of this photocatalyst is still a marked question on its utilization for fuel production and organic oxidation (Dong et al., 2019; Liu Y. et al., 2019; Zhu et al., 2019c; Qi et al., 2020b). Therefore, these problems need to be tackled in future generation of semiconductor photocatalysis.
SnO2 is an excellent UV responsive metal oxide photocatalyst with a band gap of 3.5 eV. Its excellent stability and tunable optical properties make it a suitable candidate for photocatalysis, solar cells, and gas sensors. More interestingly, its CB is situated at a suitable position below the CB of g-C3N4 and thermodynamically acts as a sink to accept the excited electrons from g-C3N4 during photocatalysis (Jana and Mondal, 2014; Xu et al., 2018a; Qi et al., 2019c,d). Therefore, its heterojunctional combination with g-C3N4 will significantly improve excited charge separation for enhanced photocatalysis.
In this work, we coupled SnO2 nanoparticles with g-C3N4 to form different ratio composites and applied for the photocatalytic production of H2 and 2-chlorophenol (2-CP) degradation under visible light, keeping in view to excite only g-C3N4 and use SnO2 as excited charge acceptor. The optimized composite (6SnO2/g-C3N4) showed much improved photoactivities for H2 production and pollutant degradation compared to bare SnO2 and g-C3N4. These activities are solely attributed to the better charge separation in the composites.
Polymeric g-C3N4 was prepared from dicyandiamide. A given amount of dicyandiamide was taken in crucible and heated at 550°C in a muffle furnace for 4 h at the rate of 2°C/min. After the completion of the heating duration, the cooled sample was grinded into fine powder and used for further study.
Chloride salt of Sn(IV) was dissolved in water, and the solution was made basic with the help of NaOH solution under vigorous stirring. During addition of NaOH, the solution initially became milky and then became clear with the addition of more NaOH. Finally, when the pH reached about 12, the solution was taken in an autoclave and heated at 220°C for 10 h in oven. The obtained white powder was purified three times with distilled water, dried in the oven overnight, and then calcined at 450°C for 1 h at the rate of 5°C/min.
Composites containing different mass percent of SnO2 and g-C3N4 (SnO2/g-C3N4) were prepared by taking appropriate amounts of SnO2 and g-C3N4 in water–methanol mixture containing 1 ml of concentrated HNO3. Each mixture was vigorously stirred under heating at 80°C till the whole solvent was evaporated. After that, each mixture was dried in the oven overnight and then calcined at 450°C for 1 h. The as prepared composites were represented by XSnO2/g-C3N4 where “X” shows the percent amount (2, 4, 6, and 8%) of SnO2 in the given composite.
The prepared samples were passed through different characterization techniques to confirm the formation of heterojunctional composites. The X-ray diffraction (XRD) technique was used to determine the crystalline structure of the samples with the help of Bruker D8 Advance diffractometer using CuKα radiation. The oxidation states of the constituent elements of the composites were examined by means of X-ray photoelectron spectroscopy (XPS) employing X-ray from mono-Al source with the help of a Kratos-Axis Ultra DLD apparatus. The obtained binding energies were calibrated with the binding energy of adventitious C-atom which is 284.55 eV. The microscopic structure was further revealed with the help of transmission electron microscope (TEM) and high-resolution TEM (HRTEM) operating at 200 kV. The optical properties were confirmed with the help of UV diffuse reflectance spectra, by taking BaSO4 as a reference, measured with a Shimadzu UV-2550 spectrophotometer. The photoluminescence (PL) spectrum of each sample was realized with the help of spectrofluorophotometer (Perkin-Elmer LS55) at a 390-nm excitation wavelength. The steady state surface photovoltage spectroscopic (SS-SPS) measurement of each sample was carried in a controlled atmosphere employing a homemade equipment possessing a lock-in amplifier (SR830) synchronized with a light chopper (SR540). Each sample was first thoroughly grinded and then kept between two indium tin oxide (ITO) glass electrodes in an atmosphere-controlled sealed container. Radiations from a 500-W Xe lamp (CHF XQ500W, Global Xe lamp power) were passed through a double-prism monochromator (SBP300) to get a monochromatic light.
Water splitting photocatalysis was carried out with the help of an online H2 production unit (Perfectlight, Beijing, Lab Solar III). About 0.1 g photocatalyst was taken in a glass-made reaction cell, and 100-ml aqueous solution of methanol (20% V/V) was added. The apparatus was first deaerated with the help of a vacuum pump to remove any traces of O2 and CO2 dissolved in aqueous solution. The mixture was irradiated under visible light (wavelength > 420 nm) coming from a 300-W Xe lamp under vigorous stirring. The produced gases were analyzed after each hour with the help of a gas chromatograph (7,900, TCD, molecular sieve 5 Å, N2 carrier, Tec comp.).
The photoactivities were further studied by selecting 2-CP as a pollutant. About 0.2 g of powder sample was mixed with 50 ml of aqueous solution (25 mg/L) of 2-CP and exposed to a 150-W (GYZ220) Xe lamp under visible light (wavelength > 420 nm). Before being exposed to light, each sample was first kept in complete dark for 30 min to attain adsorption–desorption equilibrium. The concentration of the pollutant was checked after each hour with the help of a Shimadzu UV-2550 spectrometer.
About 0.05 g powder sample was mixed with 50 ml of aqueous solution of coumarin (0.001 M) and exposed to a 150-W (GYZ220) Xe lamp under visible light (wavelength > 420 nm). Before exposure to light, each sample was first kept in complete dark for 30 min to attain adsorption–desorption equilibrium. After each hour, the amount of formed 7-hydroxycoumarin was checked at 390-/460-nm excitation/emission wavelengths with the help of a spectrofluorophotometer (Perkin-Elmer LS55).
The crystal structure study of the pure g-C3N4 shows two characteristic diffraction peaks at 13.04 and 27.31° as shown in Figure 1A. The former peak is due to the interplanner stacking of the aromatic rings in conjugation while the later peak is related to the interlayer structural units (Liu et al., 2017; Guan et al., 2018; Xu et al., 2018b, 2019b). Similarly, pure SnO2 shows diffraction peaks at 26.2, 33.8, 37.3, 51.2, 57.2, 61.1, 63.81, 64.77, 71.38, and 78.27°, which can be, respectively, indexed to (110), (101), (200), (220), (002), (310), (112), (301), (202), and (321) planes of tetragonal SnO2 nanoparticles (Mahjouri et al., 2020; Shokrzadeh et al., 2020). Interestingly, all the composite samples show the two characteristic peaks of g-C3N4 at 13.04 and 27.31° and SnO2 peaks at 33.8, 37.3, and 51.2°. However, the diffraction peak at 13.04° has been decreased progressively as the amount of SnO2 is increased, indicating that SnO2 nanoparticles are well packed in the nanosheets of g-C3N4.
Figure 1. X-ray diffraction (XRD) pattern (A) and diffuse reflectance spectroscopy (DRS) (B) of g-C3N4, SnO2, and XSnO2/g-C3N4. Transmission electron microscope (TEM) image (C) and high-resolution TEM (HRTEM) images (D) of 6SnO2/g-C3N4.
The UV-vis diffused reflectance spectra of the samples are shown in Figure 1B. As can be seen, g-C3N4 and SnO2, respectively, show optical thresholds at 475 and 360 nm, corresponding to band gaps of 2.61 and 3.45 eV, respectively. Although the composite samples show the same optical thresholds at 475 and 360 nm, their light absorption has been slightly decreased as SnO2 is a wide-band-gap semiconductor and its coupling with g-C3N4 slightly decreases light absorption (Zhang et al., 2018; Zada et al., 2019a; Zhu et al., 2019a,b).
The TEM image of composite shows uniform distribution of small SnO2 nanoparticles of about 10-nm size on the surface of g-C3N4 as shown in Figure 1C. The HRTEM image shows the lattice fringes of 0.33-nm interplanar distance corresponding to the (110) plane of SnO2 (Figure 1D). This shows that both g-C3N4 and SnO2 are in close contact with each other to intensify the charge separation for better photoactivities.
The oxidation states of different elements in the samples were determined using XPS measurements, and the results are shown in Figure 2. The obtained binding energies were calibrated with the binding energy of the adventitious carbon atom with a binding energy value of 284.55 eV. It is obvious that C1s in pure g-C3N4 shows two XPS peaks at binding energies of 284.7 and 288.2 eV (Figure 2A). These peaks are attributed to the sp2 hybridized C-atoms, respectively, bonded with N-atom of the aromatic ring and NH2 group linking different aromatic rings. Similarly, the XPS binding energies of N1s in pure g-C3N4 are composed of two parts at 398.4 and 400.6 eV and are, respectively, contributed by sec. and ter. N-atoms (Figure 2B) (Raziq et al., 2016; Li Q. et al., 2019). The XPS peaks of Sn in pure SnO2 are deconvoluted into two parts at 486.82 and 495.26 eV, which are, respectively, contributed by Sn3d5/2 and Sn3d3/2 and show that Sn is present in +4 oxidation state in the nanocomposite (Figure 2C) (Li H. et al., 2019). When g-C3N4 nanosheets are coupled with SnO2 nanoparticles, the C1s and N1s peaks are slightly shifted toward the low-energy side while those of Sn are shifted toward the high-energy side. The binding energies of O1s in Figure 2D are contributed at 529.6 and 531.1 eV, which are, respectively, contributed by the lattice O-atom and adsorbed oxygen molecules. The redistribution of charge density in both components of the nanocomposite indicates that both g-C3N4 and SnO2 are present in close contact with each other for improved charge separation.
Figure 2. X-ray photoelectron spectra of C1s (A), N1s (B), Sn 3d (C), and O1s (D) of g-C3N4 and SnO2.
The photoactivities of composites were first evaluated by splitting water under visible light (wavelength > 420 nm) in the presence of methyl alcohol. As shown in the Figure 3A, the H2 production activity of pure SnO2 is negligible under visible light irradiation. However, pure g-C3N4 produces about 10 μmol of H2 in 1 h under the stipulated conditions. Interestingly, these H2 photoactivities are significantly enhanced when both g-C3N4 and SnO2 are coupled to form heterojunctional composites. Further, photoactivities are increased along with the increase in the amount of SnO2 nanoparticles and the highest activities are contributed by 6SnO2/g-C3N4 sample, which are 132 μmol/h. However, further increase in the amount of SnO2 decreases H2 production as SnO2 is a wide-band-gap semiconductor and it covers most surface of the g-C3N4 to prevent absorption of visible-light photons. These enhanced H2 activities are solely attributed to the improved charge separation in the composites by transferring excited electrons from g-C3N4 to SnO2 for the reduction of water. We further extended the photoactivities by measuring the degradation of 2-CP under visible-light (wavelength > 420 nm) irradiation. Again, the degradation performance of pure SnO2 is very low due to its high-band-gap nature. The composite materials showed much improved photoactivities, and the optimized 6SnO2/g-C3N4 sample showed 42% degradation activities under the given conditions as shown in Figure 3B. We also carried out the stability test of the optimized sample for five consecutive cycles, each cycle composed of a 5-h run. It is obvious from Figure 3C that there is no detectable decrease in the H2 production activities, indicating that the optimized sample is very stable.
Figure 3. Photoactivities for H2 evolution (A), 2-CP degradation (B) of pure g-C3N4, and XSnO2/g-C3N4 and stability test of 6SnO2/g-C3N4 (C).
The improved photoactivities of the composites compared to pure g-C3N4 are attributed to the extended charge separation. In order to determine it, we carried out PL spectra, keeping excitation λ at 390 nm. It is clear from Figure 4A that g-C3N4 gives intense peak at 470 nm, indicating poor charge separation. However, the intensities of the composites are progressively decreased as the amount of SnO2 nanoparticles is increased, and the optimized sample shows relatively low PL peak, indicating excellent charge separation in it (Zhang et al., 2015; Ali et al., 2016; Lu et al., 2018; Ali N. et al., 2019; Ali S. et al., 2019). The relatively low intensities of PL peaks indicate enhanced charge separation and are responsible for improved photoactivities.
Figure 4. Photoluminescence spectra (A), SS-SPS (B), and coumarin fluorescent spectra (C) of pure g-C3N4 and XSnO2/g-C3N4.
We further extended the charge separation experiments by measuring the atmosphere-controlled steady state surface photovoltage spectra (SS-SPS), and the results are shown in Figure 4B. As evident, g-C3N4 shows very low SPS intensity. However, the SPS peak intensities are much improved when both g-C3N4 and SnO2 are coupled and the optimized 6SnO2/g-C3N4 sample shows the highest peak intensity. Since high is the intensity of the SPS peak, high is the charge separation (Zada et al., 2018, 2019a,b); therefore, we can say that the composites impart enhanced charge separation and contributing to the improved photoactivities.
We also measured the hydroxyl radical (·OH) activities of the fabricated samples by doing coumarin fluorescent experiments under visible-light irradiation. Since ·OH is the major contributor to charge separation during photocatalysis and react with coumarin to form 7-hydroxycoumarin; therefore, the higher the intensity of coumarin fluorescent peak, the higher is the charge separation. As can be seen from Figure 4C, pure g-C3N4 gives very low peak, which shows its inherited low charge separation. However, the ·OH radical activities are considerably improved when both g-C3N4 and SnO2 are coupled, indicating improved charge separation and hence extended photoactivities (Ali et al., 2018a; Yasmeen et al., 2019a).
The improved charge separation in the prepared composite results in the enhanced H2 production and 2-CP degradation. This enhanced charge separation has been schematically shown in Figure 5. The band gap of g-C3N4 is about 2.7 eV and absorbs visible-light photons (Raziq et al., 2015, 2017). Its CB present at −1.3 eV is most suitable for H2 production and superoxide generation which require reduction potential of 0.00 and −0.33 eV, respectively. Its VB is present at 1.4 eV (Yasmeen et al., 2019b). On the other side, the band gap of SnO2 is 3.5 eV, and its CB is present below the CB of g-C3N4 (Zada et al., 2016). Under visible-light irradiation, only g-C3N4 is excited, and electrons jumped to its CB, leaving positive holes in the VB. Since the excited electrons have a very short lifetime; therefore, they jumped to the CB of SnO2 to achieve some stability for a while. Here these electrons reduce water into H2 while the holes in VB of g-C3N4 are solely left to carryout oxidation of alcohol. In case of 2-CP degradation, these positive holes either directly oxidize pollutants or undergo the formation of more reactive species such as hydroxyl-free radicals, which then finally degrade the target pollutant into simple CO2 and water (Zada et al., 2018).
Figure 5. Schematic representation of charge separation, H2 production, and pollutant degradation by 6SnO2/g-C3N4.
In order to overcome energy crises and environmental pollution, here, we synthesized g-C3N4 nanosheets and coupled them with SnO2 nanoparticles. The optimized composite of 6SnO2/g-C3N4 produced about 132 μmol of H2 from water in 1 h and degraded 42% 2-CP pollutant under visible-light irradiation as compared to the photoactivities of bare g-C3N4 and SnO2. These enhanced photoactivities are attributed to the better charge separation as the excited electrons thermodynamically transfer from g-C3N4 to SnO2 as has been confirmed from photoluminescence spectra, steady state surface photovoltage spectroscopic measurement, and formed hydroxyl radicals. It is believed that this work would provide a feasible route to synthesize photocatalysts for improved energy production and environmental purification.
The raw data supporting the conclusions of this article will be made available by the authors, without undue reservation, to any qualified researcher.
All authors listed have made a substantial, direct and intellectual contribution to the work, and approved it for publication.
This work was supported by the Doctoral Scientific Research Foundation of Liaoning Province (20170520011) and Project of Education Office of Liaoning Province (LQN201712).
The authors declare that the research was conducted in the absence of any commercial or financial relationships that could be construed as a potential conflict of interest.
Ali, A., Hussain, Z., Arain, M. B., Shah, N., Khan, K. M., Gulab, H., et al. (2016). Development of microwave assisted spectrophotometric method for the determination of glucose. Spectrochim. Acta Mol. Biomol. Spectrosc. 153, 374–378. doi: 10.1016/j.saa.2015.07.104
Ali, N., Awais, K. T., Ul-Islam, Khan, M. A., Shah, S. J., and Zada, A. (2018b). Chitosan-coated cotton cloth supported copper nanoparticles for toxic dye reduction. Int. J. Biol. Macromol. 111, 832–838. doi: 10.1016/j.ijbiomac.2018.01.092
Ali, N., Zada, A., Zahid, M., Ismail, A., Rafiq, M., Riaz, A., et al. (2019). Enhanced photodegradation of methylene blue with alkaline and transition-metal ferrite nanophotocatalysts under direct sun light irradiation. J. Chin. Chem. Soc. 66, 402–408. doi: 10.1002/jccs.201800213
Ali, S., Li, Z., Chen, S., Zada, A., Khan, I., Khan, I., et al. (2019). Synthesis of activated carbon-supported TiO2-based nano-photocatalysts with well recycling for efficiently degrading high-concentration pollutants. Catal. Today 335, 557–564. doi: 10.1016/j.cattod.2019.03.044
Ali, W., Ullah, H., Zada, A., Alamgir, M. K., Muhammad, W., Ahmad, M. J., et al. (2018a). Effect of calcination temperature on the photoactivities of ZnO/SnO2 nanocomposites for the degradation of methyl orange. Mater. Chem. Phys. 213, 259–266. doi: 10.1016/j.matchemphys.2018.04.015
Dong, D., Yan, C., Huang, J., Lu, N., Wu, P., Wang, J., et al. (2019). An electron-donating strategy to guide the construction of MOF photocatalysts toward co-catalyst-free highly efficient photocatalytic H2 evolution. J. Mater. Chem. A 7, 24180–24185. doi: 10.1039/C9TA06141J
Fu, J., Xu, Q., Low, J., Jiang, C., and Yu, J. (2019). Ultrathin 2D/2D WO3/g-C3N4 step-scheme H2-production photocatalyst. Appl. Catal. B Environ. 243, 556–565. doi: 10.1016/j.apcatb.2018.11.011
Gautam, S., Shandilya, P., Singh, V. P., Raizada, P., and Singh, P. (2016). Solar photocatalytic mineralization of antibiotics using magnetically separable NiFe2O4 supported onto graphene sand composite and bentonite. J. Water Process Eng. 14, 86–100. doi: 10.1016/j.jwpe.2016.10.008
Guan, W., Zhang, Z., Tian, S., and Du, J. (2018). Ti4O7/g-C3N4 for visible light photocatalytic oxidation of hypophosphite: effect of mass ratio of Ti4O7/g-C3N4. Front. Chem. 6:313. doi: 10.3389/fchem.2018.00313
Jana, S., and Mondal, A. (2014). Fabrication of SnO2/α-Fe2O3, SnO2/α-Fe2O3-PB heterostructure thin films: enhanced photodegradation and peroxide sensing. ACS Appl. Mater. Interfaces 6, 15832–15840. doi: 10.1021/am5030879
Li, F., Wangyang, P., Zada, A., Humayun, M., Wang, B., and Qu, Y. (2016). Synthesis of hierarchical Mn2O3 microspheres for photocatalytic hydrogen production. Mater. Res. Bull. 84, 99–104. doi: 10.1016/j.materresbull.2016.07.032
Li, H., Zhang, B., Wang, X., Zhang, J., An, T., Ding, Z., et al. (2019). Heterostructured SnO2-SnS2@C embedded in nitrogen-doped graphene as a robust anode Material for lithium-ion batteries. Front. Chem. 7:339. doi: 10.3389/fchem.2019.00339
Li, Q., Shi, T., Li, X., Lv, K., Li, M., Liu, F., et al. (2018a). Remarkable positive effect of Cd(OH)2 on CdS semiconductor for visible-light photocatalytic H2 production. Appl. Catal. B: Environ. 229, 8–14. doi: 10.1016/j.apcatb.2018.01.078
Li, Q., Xia, Y., Yang, C., Lv, K., Lei, M., and Li, M. (2018b). Building a direct Z-scheme heterojunction photocatalyst by ZnIn2S4 nanosheets and TiO2 hollow spheres for highly-efficient artificial photosynthesis. Chem. Eng. J. 349, 287–296. doi: 10.1016/j.cej.2018.05.094
Li, Q., Zhao, T., Li, M., Li, W., Yang, B., Qin, D., et al. (2019). One-step construction of Pickering emulsion via commercial TiO2 nanoparticles for photocatalytic dye degradation. Appl. Catal. B Environ. 249, 1–8. doi: 10.1016/j.apcatb.2019.02.057
Liu, C., Raziq, F., Li, Z., Qu, Y., Zada, A., and Jing, L. (2017). Synthesis of TiO2/g-C3N4 nanocomposites with phosphate-oxygen functional bridges for improved photocatalytic activity. Chin. J. Catal. 38, 1072–1078. doi: 10.1016/S1872-2067(17)62850-X
Liu, M., Wageh, S., Al-Ghamdi, A. A., Xia, P., Cheng, B., Zhang, L., et al. (2019). Quenching induced hierarchical 3D porous g-C3N4 with enhanced photocatalytic CO2 reduction activity. Chem. Commun. 55, 14023–14026. doi: 10.1039/C9CC07647F
Liu, Y., Zhang, Z., Fang, Y., Liu, B., Huang, J., Miao, F., et al. (2019). IR-Driven strong plasmonic-coupling on Ag nanorices/W18O49 nanowires heterostructures for photo/thermal synergistic enhancement of H2 evolution from ammonia borane. Appl. Catal. B Environ. 252, 164–173. doi: 10.1016/j.apcatb.2019.04.035
Lu, N., Zhang, Z., Wang, Y., Liu, B., Guo, L., Wang, L., et al. (2018). Direct evidence of IR-driven hot electron transfer in metal-free plasmonic W18O49/Carbon heterostructures for enhanced catalytic H2 production. Appl. Catal. B Environ. 233, 19–25. doi: 10.1016/j.apcatb.2018.03.073
Mahjouri, S., Nasab, M. K., Kazemi, E. M., Divband, B., and Movafeghi, A. (2020). Effect of Ag-doping on cytotoxicity of SnO2 nanoparticles in tobacco cell cultures. J. Hazard. Mater. 381:121012. doi: 10.1016/j.jhazmat.2019.121012
Nie, N., Zhang, L., Fu, J., Cheng, B., and Yu, J. (2018). Self-assembled hierarchical direct Z-scheme g-C3N4/ZnO microspheres with enhanced photocatalytic CO2 reduction performance. Appl. Surf. Sci. 441, 12–22. doi: 10.1016/j.apsusc.2018.01.193
Qi, K., Li, Y., Xie, Y., Liu, S., Zheng, K., Chen, Z., et al. (2019a). Ag loading enhanced photocatalytic activity of g-C3N4 porous nanosheets for decomposition of organic pollutants. Front. Chem. 7:91. doi: 10.3389/fchem.2019.00091
Qi, K., Liu, S., Chen, Y., Xia, B., and Li, G. (2018b). A simple post-treatment with urea solution to enhance the photoelectric conversion efficiency for TiO2 dye-sensitized solar cells. Sol. Energy Mater. Sol. Cells 183, 193–199. doi: 10.1016/j.solmat.2018.03.038
Qi, K., Liu, S., and Qiu, M. (2018a). Photocatalytic performance of TiO2 nanocrystals with/without oxygen defects. Chin. J. Catal. 39, 867–875. doi: 10.1016/S1872-2067(17)62999-1
Qi, K., Liu, S., Selvaraj, R., Wang, W., and Yan, Z. (2019b). Comparison of Pt and Ag as co-catalyst on g-C3N4 for improving photocatalytic activity: experimental and DFT studies. Desalination Water Treat. 153, 244–252. doi: 10.5004/dwt.2019.24079
Qi, K., Liu, S., Wang, R., Chen, Z., and Selvaraj, R. (2019c). Pt/g-C3N4 composites for photocatalytic H2 production and OH formation. Desalination Water Treat. 154, 312–319. doi: 10.5004/dwt.2019.24068
Qi, K., Lv, W., Khan, I., and Liu, S. (2020a). Photocatalytic H2 generation via CoP quantum-dot-modified g-C3N4 from electroless plating synthesis. Chin. J. Catal. 41, 114–121. doi: 10.1016/S1872-2067(19)63459-5
Qi, K., Xie, Y., Wang, R., Liu, S., and Zhao, Z. (2019d). Electroless plating Ni-P cocatalyst decorated g-C3N4 with enhanced photocatalytic water splitting for H2 generation. Appl. Surf. Sci. 466, 847–853. doi: 10.1016/j.apsusc.2018.10.037
Qi, K., Xing, X., Zada, A., Li, M., Wang, Q., Liu, S., et al. (2020b). Transition metal doped ZnO nanoparticles with enhanced photocatalytic and antibacterial performances: experimental and DFT studies. Ceram. Int. 46, 1494–1502. doi: 10.1016/j.ceramint.2019.09.116
Qu, Y., Sun, N., Humayun, M., Zada, A., Xie, Y., Tang, J., et al. (2018). Improved visible-light activities of nanocrystalline CdS by coupling with ultrafine NbN with lattice matching for hydrogen evolution. Sustainable Energy Fuels 2, 549–552. doi: 10.1039/C7SE00610A
Raizada, P., Kumari, J., Shandilya, P., and Singh, P. (2017). Kinetics of photocatalytic mineralization of oxytetracycline and ampicillin using activated carbon supported ZnO/ZnWO4 nanocomposite in simulated wastewater. Desalination Water Treat. 79, 204–213. doi: 10.5004/dwt.2017.20831
Ran, J., Guo, W., Wang, H., Zhu, B., Yu, J., and Qiao, S. (2018). Metal-free 2D/2D phosphorene/g-C3N4 Van der Waals heterojunction for highly enhanced visible-light photocatalytic H2 production. Adv. Mater. 30, 1800128. doi: 10.1002/adma.201800128
Raziq, F., Li, C., Humayun, M., Qu, Y., Zada, A., Yu, H., et al. (2015). Synthesis of TiO2/g-C3N4 nanocomposites as efficient photocatalysts dependent on the enhanced photogenerated charge separation. Mater. Res. Bull. 70, 494–499. doi: 10.1016/j.materresbull.2015.05.018
Raziq, F., Qu, Y., Humayun, M., Zada, A., Yu, H., and Jing, L. (2017). Synthesis of SnO2/B-P codoped g-C3N4 nanocomposites as efficient cocatalyst-free visible-light photocatalysts for CO2 conversion and pollutant degradation. Appl. Catal. B Environ. 201, 486–494. doi: 10.1016/j.apcatb.2016.08.057
Raziq, F., Qu, Y., Zhang, X., Humayun, M., Wu, J., Zada, A., et al. (2016). Enhanced cocatalyst-free visible-light activities for photocatalytic fuel production of g-C3N4 by trapping holes and transferring electrons. J. Phys. Chem. C 120, 98–107. doi: 10.1021/acs.jpcc.5b10313
Shokrzadeh, L., Mohammadi, P., Mahmoudian, M. R., Basirun, W. J., and Bahreini, M. (2020). L-glycine-assisted synthesis of SnO2/Pd nanoparticles and their application in detection of biodeteriorating fungi. Mater. Chem. Phys. 240:122172. doi: 10.1016/j.matchemphys.2019.122172
Singh, P., Gautam, S., Shandilya, P., Priya, B., Singh, V. P., and Raizada, P. (2017). Graphene bentonite supported ZnFe2O4 as superparamagnetic photocatalyst for antibiotic degradation. Adv. Mater. Lett. 8, 229–238. doi: 10.5185/amlett.2017.1467
Ullah, M., Nazir, R., Khan, M., Khan, W., Shah, M., Afridi, S. G., et al. (2019). Effective removal of heavy metals from water by activated carbon adsorbents of Albizia lebbeck and Melia azedarach seed shells. Soil Water Res. 15, 30–37. doi: 10.17221/212/2018-SWR
Wang, J., Qin, C., Wang, H., Chu, M., Zada, A., Zhang, X., et al. (2018). Exceptional photocatalytic activities for CO2 conversion on Al-O bridged g-C3N4/α-Fe2O3 Z-scheme nanocomposites and mechanism insight with isotopesz. Appl. Catal. B Environ. 221, 459–466. doi: 10.1016/j.apcatb.2017.09.042
Xu, B., Zada, A., Wang, G., and Qu, Y. (2019a). Boosting the visible-light photoactivities of BiVO4 nanoplates by doping Eu and coupling CeOx nanoparticles for CO2 reduction and organic oxidation. Sust. Energy Fuels 3, 3363–3369. doi: 10.1039/C9SE00409B
Xu, D., Cheng, B., Wang, W., Jiang, C., and Yu, J. (2018a). Ag2CrO4/g-C3N4/graphene oxide ternary nanocomposite Z-scheme photocatalyst with enhanced CO2 reduction activity. Appl. Catal. B Environ. 231, 368–380. doi: 10.1016/j.apcatb.2018.03.036
Xu, Q., Zhu, B., Cheng, B., Yu, J., Zhou, M., and Ho, W. (2019b). Photocatalytic H2 evolution on graphdiyne/g-C3N4 hybrid nanocomposites. Appl. Catal. B Environ. 255:117770. doi: 10.1016/j.apcatb.2019.117770
Xu, Q., Zhu, B., Jiang, C., Cheng, B., and Yu, J. (2018b). Constructing 2D/2D Fe2O3/g-C3N4 direct Z-scheme photocatalysts with enhanced H2 generation performance. Sol. PRL 2:1800006. doi: 10.1002/solr.201800006
Yasmeen, H., Zada, A., Li, W., Xu, M., and Liu, S. (2019b). Suitable energy platform of Bi2WO6 significantly improves visible-light degradation activity of g-C3N4 for highly toxic diuron pollutant. Mater. Sci. Semicond. Process. 102:104598. doi: 10.1016/j.mssp.2019.104598
Yasmeen, H., Zada, A., and Liu, S. (2019a). Dye loaded MnO2 and chlorine intercalated g-C3N4 coupling impart enhanced visible light photoactivities for pollutants degradation. J. Photochem. Photobiol. A Chem. 380:111867. doi: 10.1016/j.jphotochem.2019.111867
Zada, A., Ali, N., Subhan, F., Anwar, N., Shah, M. I. A., Ateeq, M., et al. (2019a). Suitable energy platform significantly improves charge separation of g-C3N4 for CO2 reduction and pollutant oxidation under visible-light. Prog. Nat. Sci. Mat. Int. 29, 138–144. doi: 10.1016/j.pnsc.2019.03.004
Zada, A., Humayun, M., Raziq, F., Zhang, X., Qu, Y., Bai, L., et al. (2016). Exceptional visible-light-driven cocatalyst-free photocatalytic activity of g-C3N4 by well-designed nanocomposites with plasmonic Au and SnO2. Adv. Energy Mater. 6:1601190, doi: 10.1002/aenm.201601190
Zada, A., Muhammad, P., Ahmad, W., Hussain, Z., Ali, S., Khan, M., et al. (2019b). Surface plasmonic-assisted photocatalysis and optoelectronic devices with noble metal nanocrystals: design, synthesis, and applications. Adv. Funct. Mater. 6:1906744. doi: 10.1002/adfm.201906744
Zada, A., Qu, Y., Ali, S., Sun, N., Lu, H., Yan, R., et al. (2018). Improved visible-light activities for degrading pollutants on TiO2/g-C3N4 nanocomposites by decorating SPR Au nanoparticles and 2,4-dichlorophenol decomposition path. J. Hazard. Mater. 342, 715–723. doi: 10.1016/j.jhazmat.2017.09.005
Zhang, Z., Huang, J., Zhang, M., Yuan, Q., and Dong, B. (2015). Ultrathin hexagonal SnS2 nanosheets coupled with g-C3N4 nanosheets as 2D/2D heterojunction photocatalysts toward high photocatalytic activity. Appl. Catal. B Environ. 163, 298–305. doi: 10.1016/j.apcatb.2014.08.013
Zhang, Z., Jiang, X., Liu, B., Guo, L., Lu, N., Wang, L., et al. (2018). IR-driven ultrafast transfer of plasmonic hot electrons in nonmetallic branched heterostructures for enhanced H2 generation. Adv. Funct. Mater. 30:1705221. doi: 10.1002/adma.201705221
Zhao, X., Zhang, J., Wang, B., Zada, A., and Humayun, M. (2015). Biochemical synthesis of Ag/AgCl nanoparticles for visible-light-driven photocatalytic removal of colored dyes. Materials 8, 2043–2053. doi: 10.3390/ma8052043
Zhu, B., Cheng, B., Zhang, L., and Yu, J. (2019a). Review on DFT calculation of s-triazine-based carbon nitride. Carbon Energy 1, 32–56. doi: 10.1002/cey2.1
Zhu, B., Wageh, S., Al-Ghamdi, A. A., Yang, S., Tian, Z., and Yu, J. (2019b). Adsorption of CO2, O2, NO and CO on s-triazine-based g-C3N4 surface. Catal. Today 335, 117–127. doi: 10.1016/j.cattod.2018.09.038
Keywords: g-C3N4, SnO2, photocatalysis, hydrogen production, organic pollutant
Citation: Zada A, Khan M, Qureshi MN, Liu S and Wang R (2020) Accelerating Photocatalytic Hydrogen Production and Pollutant Degradation by Functionalizing g-C3N4 With SnO2. Front. Chem. 7:941. doi: 10.3389/fchem.2019.00941
Received: 12 November 2019; Accepted: 26 December 2019;
Published: 18 February 2020.
Edited by:
Liwei Wang, Guangxi University, ChinaReviewed by:
Zhenyi Zhang, Dalian Nationalities University, ChinaCopyright © 2020 Zada, Khan, Qureshi, Liu and Wang. This is an open-access article distributed under the terms of the Creative Commons Attribution License (CC BY). The use, distribution or reproduction in other forums is permitted, provided the original author(s) and the copyright owner(s) are credited and that the original publication in this journal is cited, in accordance with accepted academic practice. No use, distribution or reproduction is permitted which does not comply with these terms.
*Correspondence: Shu-yuan Liu, bGl1c2h1eXVhbkBzeW1jLmVkdS5jbg==; Ruidan Wang, d2FuZ3J1aWRhbjE5ODBAMTYzLmNvbQ==
Disclaimer: All claims expressed in this article are solely those of the authors and do not necessarily represent those of their affiliated organizations, or those of the publisher, the editors and the reviewers. Any product that may be evaluated in this article or claim that may be made by its manufacturer is not guaranteed or endorsed by the publisher.
Research integrity at Frontiers
Learn more about the work of our research integrity team to safeguard the quality of each article we publish.