- Institute of Chemistry, Saint-Petersburg State University, Saint-Petersburg, Russia
A layered perovskite-type oxide intercalated with n-butylamine is reported as an efficient photocatalyst for hydrogen production from aqueous solutions of alcohols for the first time. The hybrid photocatalyst H2Nd2Ti3O10×BuNH2 was synthesized by solid-state ceramic method followed by protonation, intercalation of methylamine and subsequent substitution by n-butylamine. The product was characterized by powder XRD, TGA, STA-MS, DRS, IR, and Raman spectroscopy, CHN analysis, SEM. Intercalation of n-butylamine caused a dramatic increase in photocatalytic activity of H2Nd2Ti3O10 in the reaction of hydrogen evolution from aqueous solutions of methanol, ethanol, and n-butanol under UV radiation. While the non-intercalated Pt-loaded H2Nd2Ti3O10 showed a maximum quantum efficiency of only 2% in the 220–340 nm range, the efficiency for hybrid samples reached 23% under the same conditions and after variation of experimental parameters even 52% efficiency was achieved. This effect may be associated with the significant expansion of the interlayer space, which is considered as a separate reaction zone.
Introduction
Excessive consumption of fuel resources and related environmental problems, which together could lead to an energy crisis, forces scientists to look for alternative energy sources, more attractive from the environmental point of view. One of the solutions to this problem is the environmentally friendly production of hydrogen fuel by splitting of water or available organic substrates using photocatalysts (Maeda, 2011). In this regard, semiconductor photocatalysts with a layered structure, especially layered perovskite-like oxides, have been intensively studied (Rodionov and Zvereva, 2016). In particular, compounds related to the Dion–Jacobson (DJ), Aurivillius (AV), and Ruddlesden–Popper (RP) phases consisting of negatively charged perovskite layers and interlayer cations with general formula A′[An−1BnO3n+1] and A′2[An−1BnO3n+1] were most actively investigated as photocatalysts (Machida et al., 2005; Compton et al., 2007; Huang et al., 2011; Chen et al., 2012; Rodionov et al., 2012, 2017a,d; Sabio et al., 2012; Zvereva and Rodionov, 2013). These materials exhibit unique photocatalytic properties derived from their layered structure, including ion-exchange and intercalation (Zvereva et al., 2011; Silyukov et al., 2015; Rodionov et al., 2017c; Shelyapina et al., 2019).
Since photocatalysts should be capable of absorbing visible light, have a large number of active catalytic sites and also support mechanisms for the efficient charge separation, different attempts have been made to modify layered oxides by cationic and anionic substitution and doping (Zou et al., 2001; Reddy et al., 2003; Kumar et al., 2011; Zhou et al., 2016; Kawashima et al., 2017a), sensitization with dyes (Youngblood et al., 2009), intercalation of metals or other inorganic particles (Huang et al., 2006, 2009) and also the creation of composites with other materials (Cui et al., 2012, 2013, 2014; Liu et al., 2018).
Hybrid organic-inorganic compounds are of great importance for modern materials science, since they allow combining the already known organic and inorganic materials and compounds with completely different properties in one composite (Gómez-Romero and Sanchez, 2006; Kickelbick, 2007). Such hybrids are interesting because the combination of components leads to improved or even new properties that were not observed in the individual components. Particularly the organic-inorganic hybrids of perovskite-like oxides can be prepared by introducing organic guests into the interlayer space while maintaining the structural features of the inorganic host. These modifications of the perovskite hosts do not require extreme conditions and lead to organic-inorganic hybrids that are stable to moderate chemical and physical impacts.
Perovskite-based hybrids are usually the result of either grafting reaction, where organic compounds are bonded to terminal interlayer oxygen atoms through strong ion-valence bonds (Tahara et al., 2007; Boykin and Smith, 2015; Shori et al., 2015), or intercalation, where organic amines form ammonium ions and interact with negatively charged perovskite layers (Tsunoda et al., 2003; Shimizu et al., 2006; Wang et al., 2007). Organic modification of layered oxide perovskites was first performed by intercalation of amines (Jacobson et al., 1987), and then expanded by covalent grafting of alcohols (Takahashi et al., 1995). Since then, the spectrum of organic compounds introduced into various protonated derivatives of the DJ, RP and AV phases has been significantly expanded (including amino alcohols, carboxylic acids, amino acids, etc.) (Hong and Kim, 1996; Han et al., 2001; Tsunoda et al., 2003; Tong et al., 2005; Takeda et al., 2006, 2008; Tahara, 2007; Wang et al., 2012, 2018; Boykin and Smith, 2015; Shori et al., 2015; Sato et al., 2017; Silyukov et al., 2018).
Despite the rather large number of currently synthesized hybrid compounds based on layered oxides, little attention has been paid to the study of the photocatalytic activity of such compounds. Perhaps this is due to concerns about the photodegradation of embedded organics during the photocatalytic process. For example, Machida et al. studied the photocatalytic activity of the layered tantalate HCa2Ta3O10 intercalated with C6H13NH2 in the reaction of water splitting under UV-light and noted a significantly higher activity of the hybrid in hydrogen evolution compared to MCa2Ta3O10 (M = Cs, Na, and H) (Machida et al., 2005). At the same time, the studied hybrid turned out to be unstable to oxidation during the photocatalytic process, which was confirmed by the collapse of the interlayer gallery and insignificant oxygen evolution, followed by a dramatic decrease in activity after the first 8 h of irradiation. More optimistic results were obtained by Wang et al. (2014) who used derivatives of the double-layered perovskite H2CaTa2O7 with n-alcohols as photocatalyst for the decomposition of rhodamine B and methyl orange under UV-visible light irradiation. Grafting of long-chain n-alcohols (n > 3) into the perovskite layers of H2CaTa2O7 improved the photocatalytic activity drastically. One can also note interesting results obtained for layered oxides intercalated by polyaniline. Such hybrids have shown themselves to be effective photocatalysts in the visible spectral region, having been tested on the model decomposition reaction of methylene blue dye (Guo et al., 2010; Zhu et al., 2013; Liu et al., 2014). Although aniline-intercalated compounds have also been obtained for layered perovskite-like oxides (Uma and Gopalakrishnan, 1994, 1995; Tong et al., 2005), the photocatalytic activity of such hybrids has not yet been studied.
A2Ln2Ti3O10 (A = Li, Na, K, Rb; Ln = La, Nd, Gd, Sm, Dy, Eu) are layered RP perovskite-type compounds with alkali cations in the interlayer space. These compounds are known to exhibit ion exchange and intercalation properties (Gopalakrishnan and Bhat, 1987; Richard et al., 1994; Rodionov et al., 2017d) and are one of the most extensively studied photocatalytic materials (Takata et al., 1997a,b; Rodionov et al., 2012; Kawashima et al., 2017b). Ni-loaded Rb2La2Ti3O10 was one of the first highly active photocatalysts for water splitting with a quantum efficiency estimated around 5% (Takata et al., 1997a). H2Ln2Ti3O10 are protonated forms of A2Ln2Ti3O10 compounds that can be obtained by acid leaching of interlayer alkali cations (Gopalakrishnan and Bhat, 1987; Richard et al., 1994). They can be used to develop new materials by intercalating organic molecules into the interlayer space (Tong et al., 2005; Tahara et al., 2007; Akbarian-Tefaghi and Wiley, 2018) as well as by exfoliation and self-assembly processes (Huang et al., 2006; Wu et al., 2006; Ida et al., 2008). However, to our best knowledge, amine-intercalated titanites of such structure have never been investigated as photocatalysts for hydrogen production. Particularly, it is unclear, if the intercalated organic molecules always undergo photodegradation, or, at some conditions, they might be stable and promote the photocatalytic process. Therefore, our work focuses on the photocatalytic properties of the n-butylamine intercalated titanate H2Nd2Ti3O10 as an example of a hybrid organic-inorganic layered material obtained by intercalation reaction. Its photocatalytic activity was investigated in the reaction of hydrogen evolution from aqueous alcohol solutions (methanol, ethanol and n-butanol) under UV radiation and compared with the initial H2Nd2Ti3O10 oxide.
Materials and Methods
Synthesis
K2Nd2Ti3O10
The initial layered oxide K2Nd2Ti3O10 was synthesized by conventional solid-state reaction (1) using K2CO3, Nd2O3 and TiO2 as reagents (Vecton, 99.9%).
The stoichiometric amounts of oxides needed to yield 20 g of the final product (7.1497 g TiO2, 10.0401 g Nd2O3) with 50% excess of potassium carbonate (5.7736 g), were weighted with an accuracy of 10−4 g (Ohaus Pioneer PA214C balance), mixed together and ground on a Fritsch Pulverisette 7 planetary micro mill with silicon nitride accessories at a speed of 600 rpm using a program of 10 repetitions of 10 min each with 5 min interval. Hexane was also added to the bowl to prevent any hydration of the reactants and provide more uniform grinding. The obtained mixture was dried at 50°C for 1 h, then at 150°C for 30 min to remove the hexane, and finally pressed into tablets of ca. 1.5 g at 50 bar using a PI 88.00 Omec hydraulic press. The tablets were placed into corundum crucibles and heated at 700°C for 2 h in a Nabertherm L-011K2RN furnace in air atmosphere. After cooling down, the tablets were ground in an agate mortar, re-pelletized and heated at 1,000°C for 10 h as the final synthesis step. The obtained tablets were again ground in an agate mortar to prepare for XRD analysis and for the next synthesis step.
H2Nd2Ti3O10
Prior to protonation, the K2Nd2Ti3O10 oxide was transformed into its hydrated form K2Nd2Ti3O10×H2O by exposition to humid air (RH = 75%) for 24 h (Utkina et al., 2018). After the XRD analysis ensured complete hydration, the sample (15 g) was dispersed in 3 L of 0.1 M hydrochloric acid and stirred for 7 days at room temperature. Then the product was separated from the solution by centrifugation (Elmi CM-6MT centrifuge), dried in a desiccator over CaO and analyzed by XRD and TGA.
H2Nd2Ti3O10×MeNH2
To intercalate methylamine into the interlayer space of H2Nd2Ti3O10, the sample (12 g) was dispersed in 120 ml of 38% aqueous MeNH2 solution, sealed in a flask and stirred for 10 days at 60°C. Afterwards, the solid was separated by centrifugation, dried in air atmosphere for 2 days and analyzed by XRD, TGA, and CHN.
H2Nd2Ti3O10×BuNH2
n-Butylamine was introduced into the interlayer space by substitution of methylamine, because the attempts of direct intercalation into H2Nd2Ti3O10 led only to non-singe phase products. The methylamine-intercalated sample was dispersed in 80 ml of BuNH2 with the addition of 10 ml of water, sealed in a flask and stirred for 4 days at room temperature. The final product was separated by centrifugation, dried in air atmosphere for 2 days, characterized by the further described methods and subsequently used for photocatalytic experiments.
Characterization
XRD Analysis
Powder XRD analysis was performed on every step of synthesis using a Rigaku Miniflex II diffractometer (CuKα radiation, 2θ range 3–60°, scan speed 10°/min). The obtained XRD patterns were indexed and the unit cell parameters were determined with an accuracy of 0.05% using Bruker Topas software.
TGA
Thermogravimetric analysis was carried out on the Netzsch TG 209 F1 Libra microbalance. In the case of H2Nd2Ti3O10, the measurement was performed in an argon atmosphere with a heating rate of 10 K/min from room temperature to 900°C. Typically, two steps of mass loss are observed on the TG curve of the protonated layered oxide H2Nd2Ti3O10 (Rodionov et al., 2017b). The first low-temperature step (RT−250°C) corresponds to the liberation of intercalated/adsorbed water (2) and the second step (300–400°C) refers to the decomposition of the protonated layered oxide with the liberation of water (3):
The protonation degree (x/2) was calculated from the mass loss at the second step using the formula
which can be easily derived from Equation (3). Here m1 stands for the sample mass before reaction (3) starts, m2 stands for the final mass after decomposition. Mi represents the molar mass of corresponding species i (K2Nd2Ti3O10, K, O, and H).
The TGA of amine-intercalated samples was performed in a synthetic dry air atmosphere (flow rate 100 ml/min) in order to ensure complete oxidation of organics as well as titanium, which might become partially reduced by organics at high temperature if the experiment is conducted in an inert atmosphere. The temperature program consisted of two steps: heating at a rate of 10 K/min from room temperature to 950°C followed by an isothermal step for 20 min.
STA-MS
Simultaneous thermal analysis coupled with the mass spectrometric detection of evolved gases (STA-MS) was carried out on a Netzsch STA 409 CD-QMS 403/5 Skimmer system using air-containing (oxidative) atmosphere (50 ml/min) at a heating rate of 20°/min.
CHN Analysis
The amounts of carbon, hydrogen and nitrogen in the hybrids were determined by the elemental CHN-analysis on a Euro EA3028-HT analyzer.
Raman Spectroscopy
Raman spectra were obtained on a Bruker Senterra spectrometer (spectral range 100–4,000 cm−1, laser 488 nm, 20 mW, spectrum accumulation time 10 s).
IR Spectroscopy
Fourier-transformed infrared (IR) absorption spectra were recorded on a Shimadzu IRAffinity-1 spectrometer (spectral range 400–4,000 cm−1, step 1 cm−1) using KBr tabletting technique.
DRS
Diffuse reflectance spectroscopy (DRS) was performed using a Shimadzu UV-2550 spectrophotometer with ISR-2200 integrating sphere attachment. The Kubelka-Munk function (F) was calculated by the formula
where R is the reflection of the sample. If the reflectance coefficient is considered to be constant, F is proportional to the absorption coefficient of the sample. The optical band gap was determined from the Tauc plot, i.e., from the cross point of linear plot sections in the coordinates (F·hν)1/2 = f (hν) corresponding to an allowed indirect transition.
SEM
The morphology of the samples was investigated by scanning electron microscopy (SEM) on the Zeiss Merlin scanning electron microscope with field emission cathode, electron optics column GEMINI-II and oil-free vacuum system. Energy-dispersive X-ray microanalysis (Oxford Instruments INCAx-act) was also carried out in order to detect the platinum co-catalyst.
BET
The specific surface area of the samples was determined using the Brunauer-Emmett-Teller (BET) method (Quadosorb SI) by measuring the amount of adsorbed nitrogen.
Photocatalytic Experiments
Experimental Technique
The photocatalyst suspension (50 mL) was placed in an external-irradiation reaction cell (Figure 1), equipped with a magnetic stirrer, a liquid cut-off filter and connected to a closed gas circulation system (120 mL dead volume). A medium-pressure mercury lamp DRT-125 (125W) was used as a radiation source. Light reaches the reaction cell only after passing through a thermostated at 15°C light filter solution (KCl + NaBr, 6 g/L each, 2 cm optical path), which cuts off radiation with λ < 220 nm (Figure 2). During the photocatalytic reaction, hydrogen accumulates in the gas phase, which composition was analyzed by an on-line gas chromatograph at certain time intervals (Shimadzu GC-2014, Rt-Msieve 5A Column, TCD, Ar carrier). At the beginning of each experiment, the system was deaerated and argon gas was introduced at atmospheric pressure.
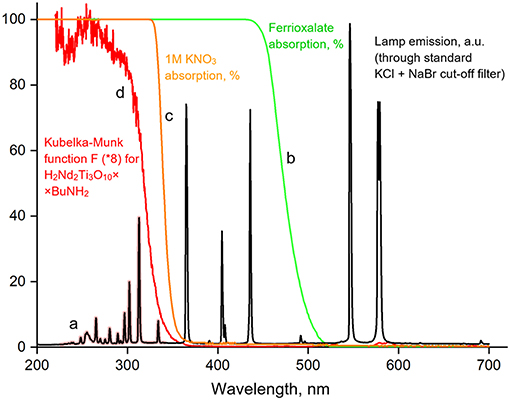
Figure 2. Emission spectrum for the DRT-125 lamp used for photocatalytic experiments (a), absorption of the light-sensitive potassium ferrioxalate solution used for light intensity measurements (b), absorption of the additional KNO3 cut-off filter (c), absorption of the H2Nd2Ti3O10×BuNH2 photocatalyst expressed by the Kubelka-Munk function (d).
To prepare the suspension for the photocatalytic experiment, 30 mg of the photocatalyst sample were added to 60 mL of aqueous alcohol solution of desired concentration (the standard concentration was 1 mol.%). The suspension was shaken and left for 1 h to establish equilibrium between the photocatalyst and the solution. Straight before the experiment, each suspension was sonicated for 10 min (Elmasonic S10H ultrasound bath) to disaggregate the photocatalyst particles.
Platinum co-catalyst nanoparticles were loaded on the photocatalyst by the common method of photocatalytic platinization in situ. During the first experimental series, 1 ml of 2.56 mmol·L−1 H2PtCl6 aqueous solution was injected into the reaction suspension after the kinetic curve of hydrogen evolution for the bare sample was collected during 2 h. The concentration corresponds to 1 wt.% of Pt with respect to the catalyst, which was chosen as a standard amount. After H2PtCl6 was introduced into the reaction cell, the system was flushed with argon for 15 min under UV-irradiation, then the system was closed and the kinetic data were collected. Since hydrogen already evolves during these 15 min at quite a high rate, its amount at the starting point of measurement is non-zero. In the second experimental series, platinization was carried out at the beginning of the experiment without the 2 h pre-radiation period. In some experiments, the platinum content was varied in the range of 0.1–5 wt.% by the corresponding adjustment of the H2PtCl6 concentration.
Determination of the Apparent Quantum Efficiency
The apparent quantum efficiency of hydrogen generation ϕ was calculated by the formula
where ω is the observed hydrogen evolution rate measured in mol·h−1 and ω0 is the theoretical maximum hydrogen evolution rate, if we assume that all incident photons with energy greater than Eg are absorbed with the generation of electron-hole pairs, which subsequently lead to hydrogen reduction and alcohol oxidation with a 100% yield without recombination and other side-reactions. According to the half-reaction
two electrons and thus two photons are needed to produce one hydrogen molecule. Therefore, ω0 equals half of the incident photon flux with λ < 340 nm (I1), which can be absorbed by the catalyst according to its bandgap. The required photon flux I1 was determined by a differential ferrioxalate actinometry method.
At the first step, we determined the photon flux with λ < 550 nm (I2) which corresponds to the absorption edge of ferrioxalate. For this aim we prepared the light-sensitive solution of potassium ferrioxalate (5 g) in 1 L of 0.1 M H2SO4, introduced 50 ml into the reaction cell and performed irradiation at the same conditions, as during photocatalytic experiments, but for shorter periods of time (10 and 20 s). The amount of generated Fe2+ ions was determined by the standard photometric method with the addition of 1,10-phenanthroline and acetate buffer. The calculated photon flux I2 was 32 mE·h−1.
At the second step, we replaced the usual light filter solution (NaBr + KCl) with a 1 M solution of KNO3, which completely cuts off light with λ < 340 nm (Figure 2). Then we performed the actinometric experiment again and thus determined the photon flux with 340 < λ < 550 nm (I3) which was found to be 17 mE·h−1. Finally, we calculated the required photon flux I1 by the difference between I2 and I3: 15 mE·h−1. This corresponds to a theoretical hydrogen evolution rate ω0 of 7.5 mmol·h−1.
Two remarks should be made about apparent quantum efficiency ϕ, determined by this approach. On the one hand, it is the lower estimate of the true quantum efficiency, because we assume 100% light absorption by the catalyst and do not take into account any scattering. On the other hand, the ϕ value is not limited by 100% because of the possible current doubling effect (Schneider and Bahnemann, 2013).
Results and Discussion
Characterization of Samples
According to powder XRD analysis (Figure 3), the layered oxide K2Nd2Ti3O10 was obtained as an almost single-phase product with a minor impurity of the hydrated form K2Nd2Ti3O10×H2O which is a result of its contact with atmospheric moisture. After prolonged contact with 75% humid air the sample completely transformed into the hydrated phase with enlarged interlayer distance d (Figure 4). The anhydrous form was indexed in the I4/mmm space group with a = 3.847 Å, c = 29.56 Å, which is close to the literature data (ICDD #01-087-0479). The hydrated form was indexed in P4/mmm with a = 3.834 Å, c = 16.65 Å.
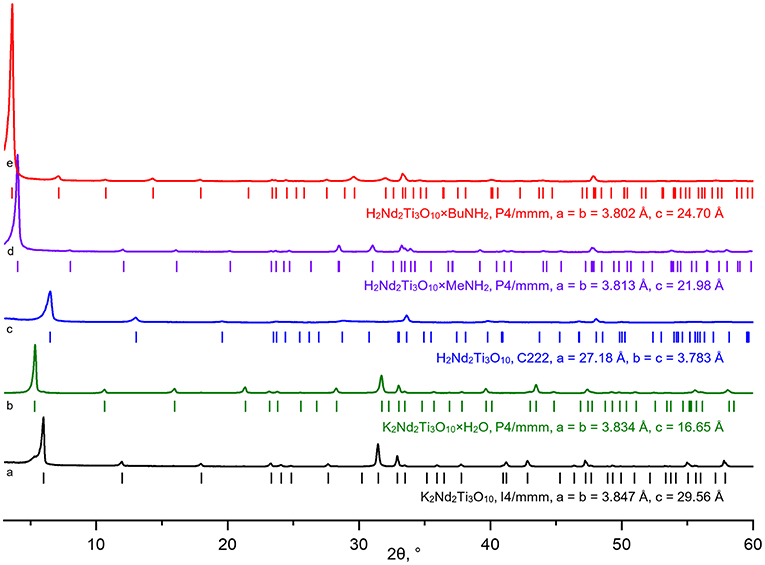
Figure 3. X-ray diffraction patterns and unit cell parameters of synthesized layered titanates K2Nd2Ti3O10 (a), K2Nd2Ti3O10×H2O (b), H2Nd2Ti3O10 (c) and amine-intercalated hybrid compounds H2Nd2Ti3O10×MeNH2 (d), H2Nd2Ti3O10×BuNH2 (e).
The ion exchange reaction in diluted hydrochloric acid led to a single-phase protonated compound H2Nd2Ti3O10 which was indexed in a C222 space group with a = 27.18 Å, b = c = 3.783 Å. The interlayer distance d, which in this case equals a/2, desreased in comparison to the initial alkaline form due to the exchange of potassium cations for more compact protons. Thermogravimetric analysis (Figure 5) revealed that the degree of protonation is 100% meaning that all potassium ions were successively substituted with protons. Also, the sample contained about 0.2 intercalated water molecules per formula unit, which are irreversible liberated at T > 150°C. Thus, its true composition can be expressed as H2Nd2Ti3O10·0.2H2O.
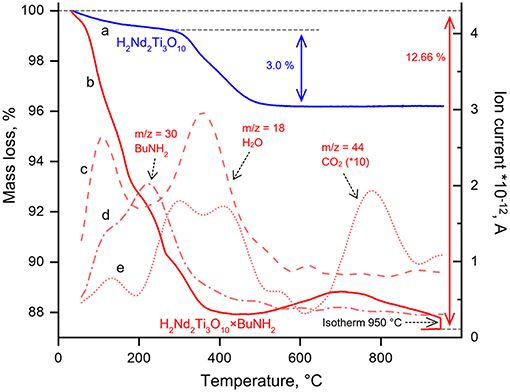
Figure 5. Thermogravimetric curves for H2Nd2Ti3O10 (a), H2Nd2Ti3O10×BuNH2 (b) and ion currents recorded during simultaneous thermal analysis coupled with mass spectrometry at m/z = 18 (c), m/z = 30 (d), m/z = 44 (e).
The treatment of the H2Nd2Ti3O10 sample with methylamine solution led to a significant increase in the interlayer distance, indicating the intercalation of methylamine molecules into the interlayer space, and further exchange reaction with n-butylamine led to even greater expansion of the interlayer gallery (Figure 4). The final product H2Nd2Ti3O10×BuNH2 was obtained as a single phase with tetragonal symmetry, which is perfectly indexed in the simple P4/mmm space group with parameters a = 3.802 Å, c = 24.70 Å.
Thermogravimetric analysis was carried out in order to determine the content of n-butylamine in the sample. The TG curve (Figure 5) demonstrates a significant mass loss from room temperature to 400°C followed by a mass increase up to 700°C and finally the loss of mass at higher temperatures. In order to explain such complex behavior, STA-MS was carried out. Mass spectrometry showed that at the first step (T < 200°C) butylamine and water are the main components which are liberated into the gas phase. Apparently, at this step we observe only the deintercalation of both compounds. At the second step (200°C < T < 500°C) not only water but also carbon dioxide is liberated, whereas the n-butylamine content in the gas phase gradually decreases. This may be associated with the beginning of the n-butylamine burning accompanied by the subsequent decomposition of the protonated H2Nd2Ti3O10 compound. At temperature about 500°C the mass of the sample begins to rise, that may be explained by the oxidation of either the remained carbon-containing species or the titanium cations, which might be reduced at lower temperatures by the reaction with organics. However, subsequent liberation of CO2 during the mass loss after 700°C clearly indicates, that some carbon species still remain in the sample after 500°C, when all butylamine is apparently gone. After an isotherm segment at 950°C for 20 min the mass of the sample stabilizes and an overall mass loss of 12.66% is reached. If we reasonably assume, that the final composition of the sample is described by the formula Nd2Ti3O9, then this mass loss gives us the upper estimate of the n-butylamine content in the sample, which is 0.9 per formula unit. While calculating this value, we also take into account the amount of H2O formed during H2Nd2Ti3O10 decomposition. However, the sample may also contain some unknown amount of intercalated water, which contributes to the mass loss at the first step. Thus, another independent method is needed to determine the composition of the sample more precisely. The use of CHN analysis data allowed to calculate the content of the organic component in the sample, which, together with thermogravimetric data, gave us following formula of the sample: H2Nd2Ti3O10·0.8BuNH2·0.4H2O. Also, CHN analysis showed that the molar ratio of C/N in this sample is close to 4, which indicates complete substitution of methylamine by n-butylamine.
Raman spectra of the protonated form and its n-butylamine derivative are presented in Figure 6. The hybrids formation is indicated by appearance of characteristic bands relating to latitudinal vibrations of C–C–H/C–N–H (1,100, 1,330 cm−1), methylene (1,460 cm−1) and amino fragments (1,575 cm−1) as well as stretching of C–N (1,055 cm−1) and C–H fragments (2,860–3,000 cm−1). Intercalation of n-butylamine is accompanied by redistribution of some bands' intensities (265–280 and 320 cm−1) and suppression of vibrations at 160–250 cm−1. The band relating to the symmetric stretching mode (ν1) of axial Ti–O bonds (810 cm−1 for the protonated forms) splits into two bands (765, 895 cm−1) during n-butylamine intercalation that points at the existence of two types of octahedra with unequal axial Ti–O distances.
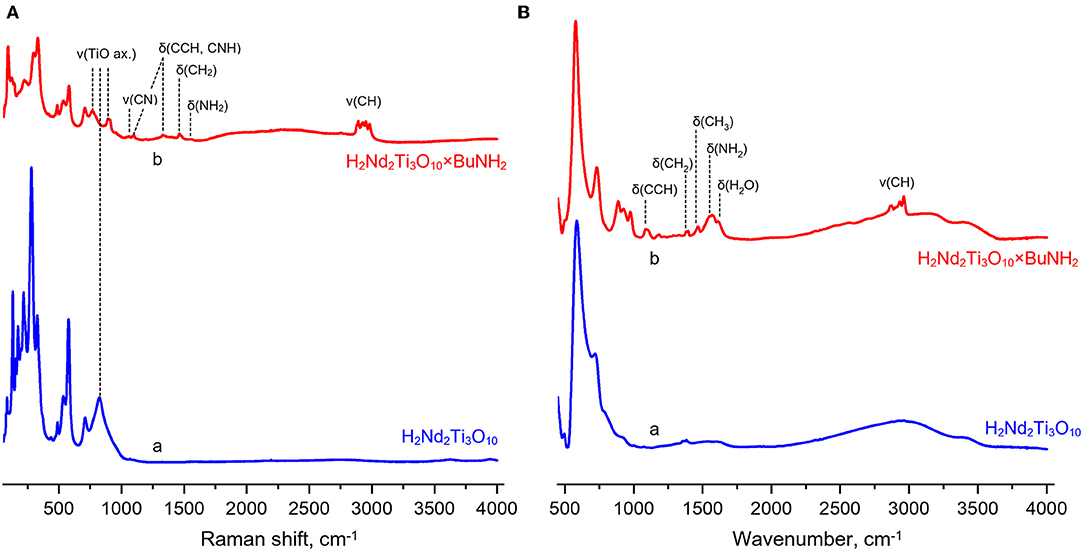
Figure 6. Raman (A) and infrared (B) spectra of the protonated titanate H2Nd2Ti3O10 (a) and its butylamine-intercalated form H2Nd2Ti3O10×BuNH2 (b).
IR spectra of the samples Figure 6 also indicate successful n-butylamine intercalation, the presence of water in the interlayer space (1,625 cm−1) and stretching of its O–H fragments (wide band at 2,800–3,500 cm−1). Thereby, IR spectroscopy indicates joint intercalation of n-butylamine and water. The absence of characteristic bands at 3,500–3,300 cm−1 relating to unprotonated amino groups –NH2 shows that n-butylamine is presented in the interlayer space in the form of n-butylammonium ions. This fact is well consistent with data from the earlier report (Tahara et al., 2007) where the presence of interlayer n-butylamine in the cationic form was confirmed using NMR spectroscopy.
The bandgap energy of the butylamine-intercalated titanate (3.58 eV) slightly exceeds that of the initial H2Nd2Ti3O10 (3.46 eV) as can be seen from the Tauc plot of the Kubelka-Munk function (Figure 7). This is quite expected, because the bandgap usually increases with the increase of the interlayer distance in the absence of additional factors (Rodionov et al., 2017d). It is important, that due to the close values of band gap energy, both catalysts absorb the same peaks in the lamp emission spectrum (Figure 2), and thus there is no factor of different amount of available light, which could otherwise contribute to the difference in observed photocatalytic activity.
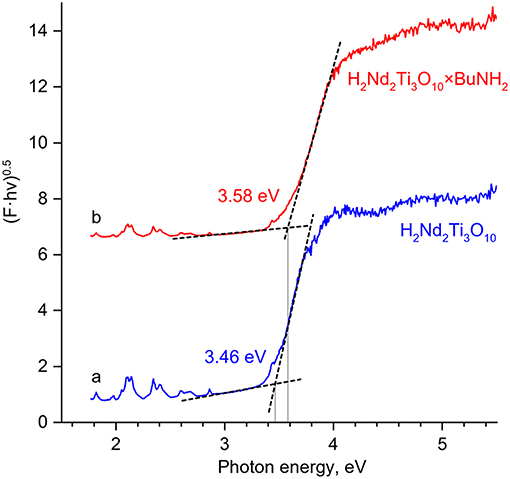
Figure 7. The Tauc plots for H2Nd2Ti3O10 (a) and H2Nd2Ti3O10×BuNH2 (b) based on diffuse reflectance measurements. The Kubelka-Munk function F is plot against the photon energy hν in the coordinates (F·hν)0.5 – hν. The bandgap energy is determined from the cross point of linear sections.
SEM investigation showed that the particle morphology of H2Nd2Ti3O10×BuNH2 is almost the same as for the initial H2Nd2Ti3O10 (Figure 8). We observe plate-like particles with an irregular size in the range of 100–1,000 nm that is typical for layered oxides prepared by the solid-state method. The morphology also does not considerably change after the photocatalytic experiment with platinization. The platinum nanoparticles can be observed at the SEM image as light dots with an average diameter of 4–6 nm. The EDX analysis also confirms the presence of 1 ± 0.4 wt.% platinum in this sample, which is consistent with the expected value.
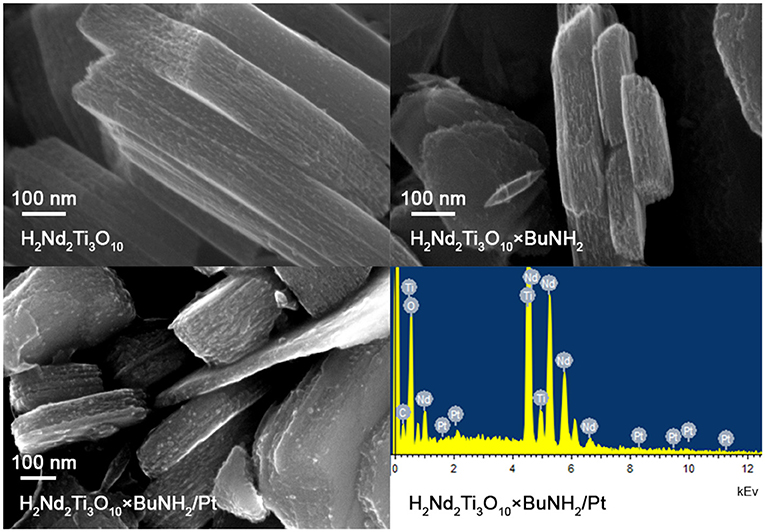
Figure 8. SEM images of initial H2Nd2Ti3O10 and H2Nd2Ti3O10×BuNH2 samples, SEM image and EDX spectrum for H2Nd2Ti3O10×BuNH2/Pt sample after the photocatalytic experiment in 1% methanol solution.
The BET surface area of the H2Nd2Ti3O10 sample was found to be 21 m2/g. Unfortunately, we were unable to measure the surface area for amine-intercalated samples due to the fact that they are unstable under vacuum conditions. However, the preservation of the particle morphology allows to assume that at least the outside surface area of the samples does not change much as a result of intercalation.
Photocatalytic Activity
The results of photocatalytic experiments are presented in Figure 9. In each case the hydrogen evolution rate ω was calculated from the slope of the kinetic curve. In case when the curve is not linear, the initial rate was calculated from the first points by linear approximation. These data, as well as the calculated values of apparent quantum efficiency ϕ, are summarized in Table 1. The standard error of hydrogen evolution rate determined in this way is estimated as 7%.
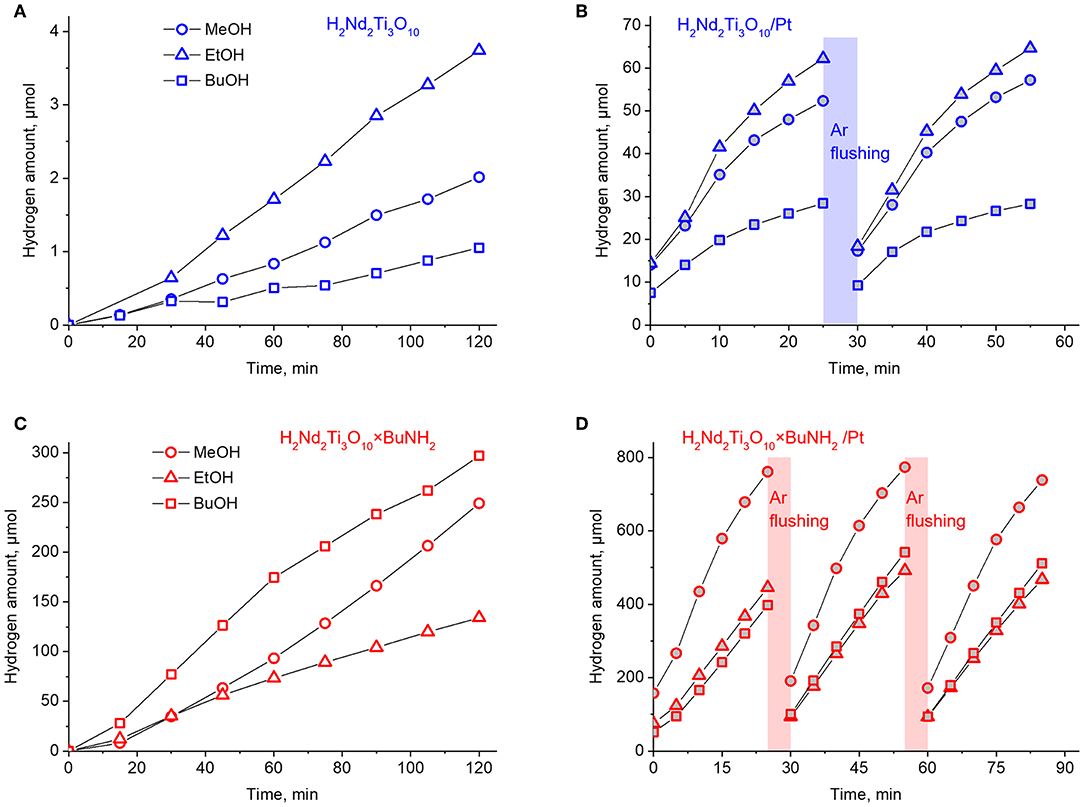
Figure 9. Kinetic curves of photocatalytic hydrogen evolution over H2Nd2Ti3O10 (A), H2Nd2Ti3O10/Pt (B), H2Nd2Ti3O10×BuNH2 (C), and H2Nd2Ti3O10×BuNH2/Pt (D).
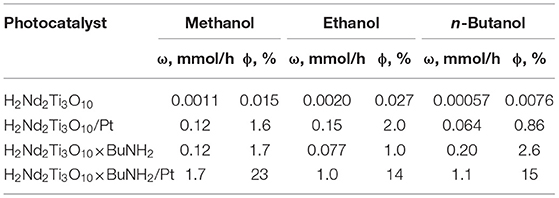
Table 1. Rate of photocatalytic hydrogen evolution (ω) and its apparent quantum efficiency at 220–340 nm (ϕ) from 1 mol.% aqueous methanol, ethanol and n-butanol solutions over H2Nd2Ti3O10, H2Nd2Ti3O10/Pt, H2Nd2Ti3O10×BuNH2 and H2Nd2Ti3O10×BuNH2/Pt.
The protonated titanate H2Nd2Ti3O10 demonstrates a very low photocatalytic activity with a quantum efficiency of hydrogen generation in the range of 0.01–0.03% depending on the alcohol nature. After 1 wt.% platinum was loaded on it as a co-catalyst, the hydrogen evolution rate significantly increased and the quantum efficiency reached 2% in the case of ethanol. This is quite expected because platinum nanoparticles serve both for the improved charge separation and as active hydrogen evolution sites. Note that both the platinized and non-platinized H2Nd2Ti3O10 showed the maximum hydrogen evolution rate for ethanol rather than methanol. Probably, this is due to the fact that ethanol is a slightly stronger reducing agent.
For the platinized sample the hydrogen evolution rate decreases with time, but restores its original value after flushing the gas system with argon. Thus, we can conclude that the deceleration is associated with the accumulation of hydrogen in the gas phase which may give rise to some backward reactions involving hydrogen and alcohol oxidation products (i.e., aldehydes) as reactants.
After n-butylamine was introduced into the interlayer space, the photocatalytic activity of the bare titanate increased to almost the same level as for the platinized non-intercalated sample in the case of methanol. It is known, that the interlayer space plays an important role in the reaction of water splitting by alkaline forms of triple-layered titanates A2Ln2Ti3O10 (A = alkaline metal, Ln = La, Nd). Remarkable activity is observed only for K- and Rb-containing compounds, which are capable of spontaneous water intercalation, whereas Li- and Na-containing compounds do not undergo water intercalation and show almost no activity. A similar relationship is also noticed for triple-layered Dion-Jacobson phases. Thus, the increased photocatalytic activity may be explained by the expansion of the interlayer space by n-butylamine, which allows the alcohol substrate molecules to intercalate and undergo oxidation between the perovskite layers. Surprisingly, in this case the maximum quantum efficiency of 2.6% was observed for n-butanol, which showed the worst performance for initial H2Nd2Ti3O10. The reason is not quite clear yet, but it may be due to the similarity of n-butanol and n-butylamine molecules, which facilitates the incorporation of alcohol molecules in the interlayer space during the reaction.
Finally, after platinization in situ the photocatalytic activity of H2Nd2Ti3O10×BuNH2 increased by one order of magnitude. This time the best quantum efficiency (23%) was achieved in the case of methanol, whereas ethanol and n-butanol gave 14–15%. The effect of reaction rate decay with time is also noted here, but it is not as significant as in the previous case. Thus, the effects of n-butylamine intercalation and platinization together gave rise to a much higher efficiency, than any of them separately. The reason is probably that these two modification methods affect different parts of the photocatalytic system and thus different reaction steps. While n-butylamine is likely to promote the alcohol oxidation in the interlayer space, the platinum co-catalyst particles facilitate the hydrogen reduction on the surface. Since during photocatalysis the oxidation and reduction reactions should proceed at the same rate, if only one of them is promoted, then the other may become rate-limiting. But if both are promoted, the rate of the whole process increases much more efficiently.
During the photocatalytic experiment with H2Nd2Ti3O10×BuNH2/Pt in 1 mol.% methanol solution hydrogen was formed with a total amount of ca. 2.5 mmol. The mass of photocatalyst in each experiment was 25 mg. Thus, even if we assume the content of n-butylamine in the sample to be equal to its upper limit according to TGA data (0.9 per formula unit), the total amount of n-butylamine in the reaction system is only 0.034 mmol. That means a more than 70-fold excess of hydrogen was generated without significant loss of reaction rate. Therefore, we can conclude that the reaction proceeds catalytically and that hydrogen is formed from the reaction solution rather than from the intercalated amine.
Since the maximum efficiency was observed for H2Nd2Ti3O10×BuNH2/Pt in methanol solution, we decided to investigate this photocatalytic system more extensive. Particularly, we studied the effect of different operating conditions, such as methanol concentration, amount of platinum loaded and the catalyst amount on the hydrogen evolution rate. We stated 1 mol.% methanol concentration, 1 wt.% platinum loading and 25 mg photocatalyst mass as standard conditions and varied one of these parameters in each experiment series. Unlike in previous cases, this time we added H2PtCl6 straight at the beginning of the experiment to obtain the platinized photocatalyst immediately. The results are presented at Figure 10.
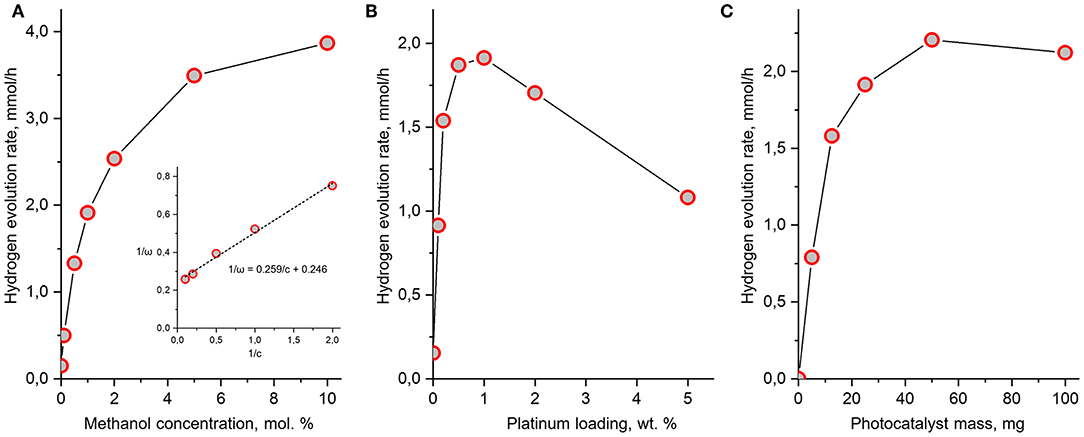
Figure 10. Dependence of hydrogen evolution rate over H2Nd2Ti3O10×BuNH2/Pt on methanol concentration (A), platinum loading (B), and photocatalyst mass (C). Unless varied, the methanol concentration is 1 mol.%, the platinum content is 1 wt.% and the mass of photocatalyst is 25 mg in every experiment.
Usually, the dependence of photocatalytic reaction rate ω on the substrate concentration c is described by the Langmuir-Hinshelwood equation
where k is the apparent rate constant, K is the adsorption constant of the substrate by the catalyst. If this is the case, a linear relation between ω−1 and c−1 should be present. As can be seen from Figure 10, such a relation between the hydrogen evolution rate and methanol concentration is indeed observed. Like usual, the reaction order with respect to methanol gradually decreases with the increase of its concentration. However, in our case the Langmuir-Hinshelwood equation is obeyed only at methanol concentrations higher than 0.5 mol.%. At lower concentrations there are deviations caused by the fact that the hydrogen evolution rate from water without methanol is not zero, but has a significant value of 0.15 mmol/h (ϕ = 2%). Since methanol is not the only reactant which can act as a source of hydrogen, but there is also water, the kinetics of the overall reaction cannot be fully described by the simple Equation (8).
At standard conditions (1 mol.% methanol) the measured hydrogen evolution rate was 1.9 mmol/h that is somewhat greater, than in the first experimental series (1.7 mmol/h). This is due to the fact that the platinization was performed immediately at the start of the experiment thus preventing any possible undesired processes, which may occur with the bare catalyst during the first 2 h of experiment, such as partial degradation n-butylamine or sedimentation.
The maximum hydrogen evolution rate (3.9 mmol/h) was observed at the maximum methanol concentration (10 mol.%) and corresponds to an apparent quantum efficiency as high as 52%.
The dependence of photocatalytic activity on the amount of loaded platinum co-catalyst represents a curve with a maximum around 1 wt.%. Such type of dependence has been reported earlier for many photocatalytic systems, including layered oxides (Kudo et al., 1988; Cui et al., 2006; Rodionov et al., 2014), but the position of the maximum always depends on the nature of the photocatalyst and co-catalyst. Thus, our standard platinum content of 1 wt.% was found to be optimal accidentally. The slow decay of hydrogen evolution rate at higher platinum content is explained by the increased light shielding of the catalyst by the platinum particles.
Finally, the observed dependence of the hydrogen evolution rate on the catalyst amount is also consistent with known examples (Minero and Vione, 2006). At low catalyst concentrations a linear dependence is observed due to the light absorption being proportional to the suspension turbidity. When the catalyst concentration is so high that almost no light transmits through the suspension, the dependence reaches saturation and the change of catalyst amount does not considerably affect the reaction rate. If the catalyst concentration is set even higher, a slow decay of reaction rate is observed due to increased light scattering. In the conditions of our experiment, the maximum efficiency is observed around 50 mg of catalyst and it is only 15% higher than at the standard amount of 25 mg. The optimal catalyst concentration is therefore 1 g/L, which is consistent with literature data for other systems.
Conclusions
We have discovered, that the intercalation of n-butylamine is an efficient method to improve the photocatalytic activity of the protonated triple-layered titanate H2Nd2Ti3O10 in the reaction of hydrogen production from aqueous solutions of methanol, ethanol and n-butanol. The obtained hybrid photocatalyst H2Nd2Ti3O10×BuNH2/Pt demonstrated an apparent quantum efficiency of 52% in the wavelength range of 220–340 nm. The hydrogen evolution rate was stable with time and the amount of generated hydrogen exceeded the amount of intercalated n-butylamine more than 70 times, indicating that hydrogen is produced from the alcohol solution rather than from the intercalated amine. The dependences of photocatalytic activity on operating conditions, such as alcohol concentration, platinum co-catalyst content and catalyst loading are well-described by the usual theoretical approaches for heterogeneous photocatalysis. We propose that the intercalation of amines is an effective strategy to improve photocatalytic properties of protonated layered oxides that can be applied to other related compounds as well. At the present moment we investigate carefully the composition, structure and properties of the samples which are obtained after photocatalytic experiments, including the platinized samples. It is important to explore what changes occur in the interlayer space during the reaction. Also, further studies involving different amines and different layered oxides are in progress.
Data Availability Statement
The datasets generated for this study are available on request to the corresponding author.
Author Contributions
IR, OS, and IZ contributed the conception and design of the study. Experimental work was carried out by EM (photocatalytic experiments, quantum efficiency), SK and AP (synthesis, characterization) under supervision of IR and OS. IR wrote the manuscript and prepared images with contributions of OS, EM, and IZ in certain sections. All authors participated in the analysis and discussion of obtained results.
Funding
This study was financially supported by the Russian Science Foundation (Project No. 19-13-00184).
Conflict of Interest
The authors declare that the research was conducted in the absence of any commercial or financial relationships that could be construed as a potential conflict of interest.
Acknowledgments
The study was technically supported by the Saint Petersburg State University Research Park. The authors were grateful to the Center of Thermal Analysis and Calorimetry (TG and STA studies), Research Centre for X-ray Diffraction Studies (XRD studies), Chemistry Educational Centre (GC for photocatalytic experiments, DRS), Centre for Chemical Analysis and Materials Research (IR spectroscopy, CHN analysis), Interdisciplinary Resource Centre for Nanotechnology (SEM), Centre for Optical and Laser Materials Research (Raman spectroscopy), Centre for Diagnostics of Functional Materials for Medicine, Pharmacology and Nanoelectronics (BET).
References
Akbarian-Tefaghi, S., and Wiley, J. B. (2018). Microwave-assisted routes for rapid and efficient modification of layered perovskites. Dalt. Trans. 47, 2917–2924. doi: 10.1039/C7DT03865H
Boykin, J. R., and Smith, L. J. (2015). Rapid microwave-assisted grafting of layered perovskites with n-alcohols. Inorg. Chem. 54, 4177–4179. doi: 10.1021/ic503001w
Chen, W., Li, C., Gao, H., Yuan, J., Shangguan, W., Su, J., et al. (2012). Photocatalytic water splitting on protonated form of layered perovskites K0.5La0.5Bi2M2O9 (M = Ta; Nb) by ion-exchange. Int. J. Hydrogen Energy 37, 12846–12851. doi: 10.1016/j.ijhydene.2012.05.090
Compton, O. C., Carroll, E. C., Kim, J. Y., Larsen, D. S., and Osterloh, F. E. (2007). Calcium niobate semiconductor nanosheets as catalysts for photochemical hydrogen evolution from water. J. Phys. Chem. C 111, 14589–14592. doi: 10.1021/jp0751155
Cui, W., Guo, D., Liu, L., Hu, J., Rana, D., and Liang, Y. (2014). Preparation of ZnIn2S4/K2La2Ti3O10 composites and their photocatalytic H2 evolution from aqueous Na2S/Na2SO3 under visible light irradiation. Catal. Commun. 48, 55–59. doi: 10.1016/j.catcom.2014.01.026
Cui, W., Liu, L., Feng, L., Xu, C., Li, Z., Lü, S., et al. (2006). Preparation of Pt/K2La2Ti3O10 and its photo-catalytic activity for hydrogen evolution from methanol water solution. Sci. China Ser. B Chem. 49, 162–168. doi: 10.1007/s11426-006-0162-6
Cui, W., Liu, L., Ma, S., Liang, Y., and Zhang, Z. (2013). CdS-sensitized K2La2Ti3O10 composite: a new photocatalyst for hydrogen evolution under visible light irradiation. Catal. Today 207, 44–49. doi: 10.1016/j.cattod.2012.05.009
Cui, W., Qi, Y., Liu, L., Rana, D., Hu, J., and Liang, Y. (2012). Synthesis of PbS–K2La2Ti3O10 composite and its photocatalytic activity for hydrogen production. Prog. Nat. Sci. Mater. Int. 22, 120–125. doi: 10.1016/j.pnsc.2012.03.002
Gómez-Romero, P., and Sanchez, C. (2006). Functional Hybrid Materials. Weinheim: Wiley-VCH Verlag GmbH & Co. KGaA.
Gopalakrishnan, J., and Bhat, V. (1987). A2Ln2Ti3O10 (A = potassium or rubidium; Ln = lanthanum or rare earth): a new series of layered perovskites exhibiting ion exchange. Inorg. Chem. 26, 4299–4301. doi: 10.1021/ic00273a001
Guo, T., Wang, L., Evans, D. G., and Yang, W. (2010). Synthesis and photocatalytic properties of a polyaniline-intercalated layered protonic titanate nanocomposite with a p–n heterojunction structure. J. Phys. Chem. C 114, 4765–4772. doi: 10.1021/jp9055413
Han, Y.-S., Park, I., and Choy, J.-H. (2001). Exfoliation of layered perovskite, KCa2Nb3O10, into colloidal nanosheets by a novel chemical process. J. Mater. Chem. 11, 1277–1282. doi: 10.1039/b006045n
Hong, Y., and Kim, S.-J. (1996). Intercalation of primary diamines in the layered perovskite oxides, HSr2Nb3O10. Bull. Korean Chem. Soc. 17, 730–735.
Huang, Y., Li, J., Wei, Y., Li, Y., Lin, J., and Wu, J. (2009). Fabrication and photocatalytic property of Pt-intercalated layered perovskite niobates H1−xLaNb2−xMoxO7 (x = 0–0.15). J. Hazard. Mater. 166, 103–108. doi: 10.1016/j.jhazmat.2008.11.040
Huang, Y., Li, Y., Wei, Y., Huang, M., and Wu, J. (2011). Photocatalytic property of partially substituted Pt-intercalated layered perovskite, ASr2TaxNb3−xO10 (A = K, H; x = 0, 1, 1.5, 2 and 3). Sol. Energy Mater. Sol. Cells 95, 1019–1027. doi: 10.1016/j.solmat.2010.12.017
Huang, Y., Wu, J., Li, T., Hao, S., and Lin, J. (2006). Synthesis and photocatalytic properties of H2La2Ti3O10/TiO2 intercalated nanomaterial. J. Porous Mater. 13, 55–59. doi: 10.1007/s10934-006-5490-6
Ida, S., Ogata, C., Eguchi, M., Youngblood, W. J., Mallouk, T. E., Matsumoto, Y., et al. (2008). Photoluminescence of perovskite nanosheets prepared by exfoliation of layered oxides, K2Ln2Ti3O10, KLnNb2O7, and RbLnTa2O7 (Ln: lanthanide ion). J. Am. Chem. Soc. 130, 7052–7059. doi: 10.1021/ja7114772
Jacobson, A. J., Johnson, J. W., and Lewandowski, J. (1987). Intercalation of the layered solid acid HCa2Nb3O10 by organic amines. Mater. Res. Bull. 22, 45–51. doi: 10.1016/0025-5408(87)90148-6
Kawashima, K., Hojamberdiev, M., Chen, S., Yubuta, K., Wagata, H., Domen, K., et al. (2017a). Understanding the effect of partial N3−-to-O2− substitution and H+-to-K+ exchange on photocatalytic water reduction activity of Ruddlesden-Popper layered perovskite KLaTiO4. Mol. Catal. 432, 250–258. doi: 10.1016/j.mcat.2017.01.004
Kawashima, K., Hojamberdiev, M., Wagata, H., Yubuta, K., Domen, K., and Teshima, K. (2017b). Protonated oxide, nitrided, and reoxidized K2La2Ti3O10 crystals: visible-light-induced photocatalytic water oxidation and fabrication of their nanosheets. ACS Sustain. Chem. Eng. 5, 232–240. doi: 10.1021/acssuschemeng.6b01344
Kickelbick, G. (2007). Hybrid Materials: Synthesis, Characterization, and Applications. Weinheim: Wiley-VCH Verlag GmbH & Co. KGaA.
Kudo, A., Tanaka, A., Domen, K., Maruya, K., Aika, K., and Onishi, T. (1988). Photocatalytic decomposition of water over NiO-K4Nb6O17 catalyst. J. Catal. 111, 67–76. doi: 10.1016/0021-9517(88)90066-8
Kumar, V., Govind, and Uma, S. (2011). Investigation of cation (Sn2+) and anion (N3−) substitution in favor of visible light photocatalytic activity in the layered perovskite K2La2Ti3O10. J. Hazard. Mater. 189, 502–508. doi: 10.1016/j.jhazmat.2011.02.064
Liu, C., Wu, L., Chen, J., Liang, J. Y., Li, C. S., Ji, H. M., et al. (2014). The nanocomposite of polyaniline and nitrogen-doped layered HTiNbO5with excellent visible-light photocatalytic performance. Phys. Chem. Chem. Phys. 16, 13409–13417. doi: 10.1039/C4CP01423E
Liu, Y., Zhou, Y., Lv, C., Zhang, C., Jin, X., Meng, Q., et al. (2018). Construction of 2D-composite HCa2Nb3O10/CaNb2O6 heterostructured photocatalysts with enhanced hydrogen production performance. New J. Chem. 42, 681–687. doi: 10.1039/C7NJ03707D
Machida, M., Mitsuyama, T., Ikeue, K., Matsushima, S., and Arai, M. (2005). Photocatalytic property and electronic structure of triple-layered perovskite tantalates, MCa2Ta3O10 (M = Cs, Na, H, and C6H13NH3). J. Phys. Chem. B 109, 7801–7806. doi: 10.1021/jp044833d
Maeda, K. (2011). Photocatalytic water splitting using semiconductor particles: history and recent developments. J. Photochem. Photobiol. C Photochem. Rev. 12, 237–268. doi: 10.1016/j.jphotochemrev.2011.07.001
Minero, C., and Vione, D. (2006). A quantitative evalution of the photocatalytic performance of TiO2 slurries. Appl. Catal. B Environ. 67, 257–269. doi: 10.1016/j.apcatb.2006.05.011
Reddy, V., Hwang, D., and Lee, J. (2003). Effect of Zr substitution for Ti in KLaTiO4 for photocatalytic water splitting. Catal. Lett. 90, 39–44. doi: 10.1023/A:1025812125852
Richard, M., Brohan, L., and Tournoux, M. (1994). Synthesis, characterization, and acid exchange of the layered perovskites: A2Nd2Ti3O10 (A–Na, K). J. Solid State Chem. 112, 345–354. doi: 10.1006/jssc.1994.1315
Rodionov, I. A., Fateev, S. A., and Zvereva, I. A. (2017a). Effect of protonation on the photocatalytic activity of the layered titanate Rb2Nd2Ti3O10. Russ. J. Gen. Chem. 87, 2728–2729. doi: 10.1134/S1070363217110317
Rodionov, I. A., Fateev, S. A., and Zvereva, I. A. (2017b). Synthesis of a new layered Rb2Nd2Ti3O10 oxide, its hydration and protonation. Glas. Phys. Chem. 43, 593–596. doi: 10.1134/S1087659617060128
Rodionov, I. A., Mechtaeva, E. V., Burovikhina, A. A., Silyukov, O. I., Toikka, M. A., and Zvereva, I. A. (2017c). Effect of protonation on the photocatalytic activity of the K2La2Ti3O10 layered oxide in the reaction of hydrogen production. Mon. Chem. 149, 475–482. doi: 10.1007/s00706-017-2105-7
Rodionov, I. A., Mechtaeva, E. V., and Zvereva, I. A. (2014). Photocatalytic activity of TiO2-MOx composites in the reaction of hydrogen generation from aqueous isopropanol solution. Russ. J. Gen. Chem. 84, 611–616. doi: 10.1134/S107036321404001X
Rodionov, I. A., Silyukov, O. I., Utkina, T. D., Chislov, M. V., Sokolova, Y. P., and Zvereva, I. A. (2012). Photocatalytic properties and hydration of perovskite-type layered titanates A2Ln2Ti3O10 (A = Li, Na, K; Ln = La, Nd). Russ. J. Gen. Chem. 82, 1191–1196. doi: 10.1134/S1070363212070018
Rodionov, I. A., Sokolova, I. P., Silyukov, O. I., Burovikhina, A. A., Fateev, S. A., and Zvereva, I. A. (2017d). Protonation and photocatalytic activity of the Rb2La2Ti3O10 layered oxide in the reaction of hydrogen production. Int. J. Photoenergy 2017, 1–8. doi: 10.1155/2017/9628146
Rodionov, I. A., and Zvereva, I. A. (2016). Photocatalytic activity of layered perovskite-like oxides in practically valuable chemical reactions. Russ. Chem. Rev. 85, 248–279. doi: 10.1070/RCR4547
Sabio, E. M., Chamousis, R. L., Browning, N. D., and Osterloh, F. E. (2012). Photocatalytic water splitting with suspended calcium niobium oxides: why nanoscale is better than bulk–a kinetic analysis. J. Phys. Chem. C 116, 3161–3170. doi: 10.1021/jp209006n
Sato, S., Shintani, K., Idota, N., Nishino, T., and Sugahara, Y. (2017). Effect of the graft density of cellulose diacetate-modified layered perovskite nanosheets on mechanical properties of the transparent organic–inorganic hybrids bearing covalent bonds at the interface. Cellulose 24, 5463–5473. doi: 10.1007/s10570-017-1475-7
Schneider, J., and Bahnemann, D. W. (2013). Undesired role of sacrificial reagents in photocatalysis. J. Phys. Chem. Lett. 4, 3479–3483. doi: 10.1021/jz4018199
Shelyapina, M. G., Nefedov, D. Y., Kostromin, A. V., Silyukov, O. I., and Zvereva, I. A. (2019). Proton mobility in Ruddlesden–Popper phase H2La2Ti3O10 studied by 1H NMR. Ceram. Int. 45, 5788–5795. doi: 10.1016/j.ceramint.2018.12.045
Shimizu, K., Itoh, S., Hatamachi, T., Kitayama, Y., and Kodama, T. (2006). Pillaring of Ruddlesden–Popper perovskite tantalates, H2ATa2O7 (A = Sr or La2/3), with n-alkylamines and oxide nanoparticles. J. Mater. Chem. 16:773–779. doi: 10.1039/B514066H
Shori, S., Pellechia, P. J., zur Loye, H.-C., and Ploehn, H. J. (2015). Covalent grafting of phenylphosphonate on calcium niobate platelets. J. Colloid Interface Sci. 437, 97–110. doi: 10.1016/j.jcis.2014.09.024
Silyukov, O. I., Abdulaeva, L. D., Burovikhina, A. A., Rodionov, I. A., and Zvereva, I. A. (2015). Phase transformations during HLnTiO4 (Ln = La, Nd) thermolysis and photocatalytic activity of obtained compounds. J. Solid State Chem. 226, 101–106. doi: 10.1016/j.jssc.2015.02.008
Silyukov, O. I., Kurnosenko, S. A., and Zvereva, I. A. (2018). Intercalation of methylamine into the protonated forms of layered perovskite-like oxides HLnTiO4 (Ln = La and Nd). Glas. Phys. Chem. 44, 428–432. doi: 10.1134/S1087659618050176
Tahara, S. (2007). Preparation of inorganic-organic hybrids via intercalation and grafting reactions of protonated forms of ion-exchangeable layered perovskites (Dissertation thesis), Waseda University, Tokyo, Japan.
Tahara, S., Ichikawa, T., Kajiwara, G., and Sugahara, Y. (2007). Reactivity of the Ruddlesden–Popper phase H2La2Ti3O10 with organic compounds: intercalation and grafting reactions. Chem. Mater. 19, 2352–2358. doi: 10.1021/cm0623662
Takahashi, S., Nakato, T., Hayashi, S., Sugahara, Y., and Kuroda, K. (1995). Formation of a methoxy-modified interlayer surface via the reaction between methanol and layered perovskite HLaNb2O7xH2O. Inorg. Chem. 34, 5065–5069. doi: 10.1021/ic00124a023
Takata, T., Furumi, Y., Shinohara, K., Tanaka, A., Hara, M., Kondo, J. N., et al. (1997a). Photocatalytic decomposition of water on spontaneously hydrated layered perovskites. Chem. Mater. 9, 1063–1064. doi: 10.1021/cm960612b
Takata, T., Shinohara, K., Tanaka, A., Hara, M., Kondo, J. N., and Domen, K. (1997b). A highly active photocatalyst for overall water splitting with a hydrated layered perovskite structure. J. Photochem. Photobiol. A Chem. 106, 45–49. doi: 10.1016/S1010-6030(97)00037-3
Takeda, Y., Momma, T., Osaka, T., Kuroda, K., and Sugahara, Y. (2008). Organic derivatives of the layered perovskite HLaNb2O7·xH2O with polyether chains on the interlayer surface: characterization, intercalation of LiClO4, and ionic conductivity. J. Mater. Chem. 18:3581–3587. doi: 10.1039/b802003e
Takeda, Y., Suzuki, H., Notsu, K., Sugimoto, W., and Sugahara, Y. (2006). Preparation of a novel organic derivative of the layered perovskite bearing HLaNb2O7·nH2O interlayer surface trifluoroacetate groups. Mater. Res. Bull. 41, 834–841. doi: 10.1016/j.materresbull.2005.10.004
Tong, Z., Zhang, G., Takagi, S., Shimada, T., Tachibana, H., and Inoue, H. (2005). Preparation and characterization of a transparent thin film of the layered perovskite, K2La2Ti3O10, intercalated with an ionic porphyrin. Chem. Lett. 34, 632–633. doi: 10.1246/cl.2005.632
Tsunoda, Y., Sugimoto, W., and Sugahara, Y. (2003). Intercalation behavior of n-alkylamines into a protonated form of a layered perovskite derived from aurivillius phase Bi2SrTa2O9. Chem. Mater. 15, 632–635. doi: 10.1021/cm0200893
Uma, S., and Gopalakrishnan, J. (1994). Synthesis of anion-deficient layered perovskites, ACa2Nb3−xMxO10−x (A = Rb, Cs; M = Al, Fe), exhibiting ion-exchange and intercalation. evidence for the formation of layered brownmillerites, ACa2Nb2AlO9 (A = Cs, H). Chem. Mater. 6, 907–912. doi: 10.1021/cm00043a008
Uma, S., and Gopalakrishnan, J. (1995). Polymerization of aniline in layered perovskites. Mater. Sci. 34, 175–179. doi: 10.1016/0921-5107(95)01235-4
Utkina, T., Chislov, M., Myshenkov, M., Rodionov, I., and Zvereva, I. (2018). Water sorption by the perovskite-like layered titanate K2Nd2Ti3O10 in humid atmosphere. J. Therm. Anal. Calorim. 134, 323–331. doi: 10.1007/s10973-018-7017-1
Wang, B., Dong, X., Pan, Q., Cheng, Z., and Yang, Y. (2007). Intercalation behavior of n-alkylamines into an A-site defective layered perovskite H2W2O7. J. Solid State Chem. 180, 1125–1129. doi: 10.1016/j.jssc.2007.01.009
Wang, C., Tang, K., Wang, D., Liu, Z., Wang, L., Zhu, Y., et al. (2012). A new carbon intercalated compound of Dion–Jacobson phase HLaNb2O7. J. Mater. Chem. 22:11086. doi: 10.1039/c2jm14902h
Wang, Y., Nikolopoulou, M., Delahaye, E., Leuvrey, C., Leroux, F., Rabu, P., et al. (2018). Microwave-assisted functionalization of the Aurivillius phase Bi2SrTa2O9: diol grafting and amine insertion vs. alcohol grafting. Chem. Sci. 9, 7104–7114. doi: 10.1039/C8SC01754A
Wang, Y., Wang, C., Wang, L., Hao, Q., Zhu, X., Chen, X., et al. (2014). Preparation of interlayer surface tailored protonated double-layered perovskite H2CaTa2O7 with n-alcohols, and their photocatalytic activity. RSC Adv. 4, 4047–4054. doi: 10.1039/C3RA44623A
Wu, J., Huang, Y., Li, T., Lin, J., Huang, M., and Wei, Y. (2006). Synthesis and photocatalytic properties of layered nanocomposite H2La2Ti3O10/Fe2O3. Scr. Mater. 54, 1357–1362. doi: 10.1016/j.scriptamat.2005.12.008
Youngblood, W. J., Lee, S.-H. A., Maeda, K., and Mallouk, T. E. (2009). Visible light water splitting using dye-sensitized oxide semiconductors. Acc. Chem. Res. 42, 1966–1973. doi: 10.1021/ar9002398
Zhou, Y., Wen, T., Guo, Y., Yang, B., and Wang, Y. (2016). Controllable doping of nitrogen and tetravalent niobium affords yellow and black calcium niobate nanosheets for enhanced photocatalytic hydrogen evolution. RSC Adv. 6, 64930–64936. doi: 10.1039/C6RA11407E
Zhu, H., Yao, X., and Hua, S. (2013). Nanocomposite of polyaniline and a layered niobate acid host: synthesis, electrochemical studies, and photocatalytic properties. Polym. Compos. 34, 834–841. doi: 10.1002/pc.22485
Zou, Z., Ye, J., and Arakawa, H. (2001). Substitution effects of In3+ by Fe3+ on photocatalytic and structural properties of Bi2InNbO7 photocatalysts. J. Mol. Catal. 168, 289–297. doi: 10.1016/S1381-1169(00)00545-8
Zvereva, I., and Rodionov, I. (2013). “Photocatalytic properties of perovskite-type layered oxides,” in Perovskite: Crystallography, Chemistry and Catalytic Performance, eds J. Zhang and H. Li (New York, NY: Nova Science Publishers), 181–198.
Keywords: photocatalysis, hydrogen production, layered titanate, perovskite-type structure, intercalation, amine, hybrid compounds
Citation: Rodionov IA, Maksimova EA, Pozhidaev AY, Kurnosenko SA, Silyukov OI and Zvereva IA (2019) Layered Titanate H2Nd2Ti3O10 Intercalated With n-Butylamine: A New Highly Efficient Hybrid Photocatalyst for Hydrogen Production From Aqueous Solutions of Alcohols. Front. Chem. 7:863. doi: 10.3389/fchem.2019.00863
Received: 10 October 2019; Accepted: 28 November 2019;
Published: 12 December 2019.
Edited by:
Eugene A. Goodilin, Lomonosov Moscow State University, RussiaReviewed by:
Van-Huy Nguyen, Duy Tan University, VietnamFernando Fresno, IMDEA Energy Institute, Spain
Kirill Sergeevich Napolskii, Lomonosov Moscow State University, Russia
Copyright © 2019 Rodionov, Maksimova, Pozhidaev, Kurnosenko, Silyukov and Zvereva. This is an open-access article distributed under the terms of the Creative Commons Attribution License (CC BY). The use, distribution or reproduction in other forums is permitted, provided the original author(s) and the copyright owner(s) are credited and that the original publication in this journal is cited, in accordance with accepted academic practice. No use, distribution or reproduction is permitted which does not comply with these terms.
*Correspondence: Ivan A. Rodionov, aS5yb2Rpb25vdkBzcGJ1LnJ1