- 1Institute of Materials Research and Engineering, A*STAR (Agency for Science, Technology and Research), Singapore, Singapore
- 2Institute of High Performance Computing, A*STAR (Agency for Science, Technology and Research), Singapore, Singapore
- 3Department of Chemistry, National University of Singapore, Singapore, Singapore
Dimethylsulfone (DMSO2), a small organic molecule, was observed to induce the alignment of poly(3,4-ethylenedioxythiophene): poly(4-styrenesulfonate) (PEDOT:PSS) via in-situ crystallization in PEDOT:PSS mixture, which was verified by field emission scanning electron microscopy (FESEM), X-ray diffraction (XRD) and atomic force microscopy (AFM). A chemically stable dopant, DMSO2, remarkably raised the electrical conductivity of the PEDOT:PSS film, which was fabricated from pre-mixed solution of PEDOT:PSS and DMSO2, up to 1080 S/cm, and more importantly, such a PEDOT:PSS film showed a long-term humidity stability and it retained near 90% electric conductivity after 60 days, suggesting DMSO2 is promising for an eco-friendly alternative to replace dimethyl sulfoxide (DMSO), ethylene glycol (EG) and various acids dopants that have been widely employed to dope and post-treat PEDOT:PSS. Pairwise interaction energies and free energy of solvation between PEDOT:PSS and DMSO2 were calculated by first-principles and molecular mechanics, respectively, revealing the mechanism of DMSO2 in enhancing the electrical conductivity.
Introduction
In the past few decades, we have witnessed great progress on thermoelectric devices due to its capability to directly convert waste heat into electricity. The efficiency of the thermoelectric materials is evaluated via the dimensionless figure-of-merit: ZT = S2σT/κ, where S, σ, T, and κ are Seebeck coefficient, electrical conductivity, thermal conductivity and absolute temperature, respectively. Previously, inorganic materials such as Bi2Te3, BiSb, SiGe, and other metal alloys (Han et al., 2014; Zhao and Tan, 2014) were extensively studied, achieving a commendable ZT of about 2 (Culebras et al., 2014b). These materials were fabricated into various devices, which were used in niche applications including refrigeration and power generation. Nevertheless, these inorganic materials are intrinsically disadvantaged because of their scarcity on earth, non-flexibility and toxicity, thus limiting their wide application in thermoelectric modules (Hu et al., 2015a). To overcome such drawbacks, conducting polymers (CPs) are currently studied as alternative TE materials, given their advantages, such as tunable electric conductivity and low thermal conductivity (Choy, 1977; Kim et al., 2013; Park et al., 2013).
The typical CPs that have been widely studied to date include polyacetylene (Kaneko et al., 1993), polypyrrole (PPy) (Kemp et al., 1999), polyaniline (PANI) (Yoon et al., 1995; Mateeva et al., 1998), polythiophene (PTH) (Hu et al., 2013) and poly(3,4-ethylenedioxythiophene):poly(styrenesulfonate) (PEDOT:PSS) (Zhang et al., 2010). The TE performance of CPs are still much lower than their inorganic counterparts due to their intrinsically low electrical conductivity and relatively low Seebeck coefficient (Culebras et al., 2014b; McGrail et al., 2015). Though the electrical conductivity can be enhanced, the Seebeck coefficient usually decreases correspondingly due to the trade-off relationship between them, resulting in a low power factor, termed as P = S2σ (Yao et al., 2010). Several approaches have been widely investigated to improve TE performance of the CPs, including (1): nanostructuring CPs, (2): hybridization with other nanostructures, including metallic and carbon-based materials and (3): surface modifications or post-treatment. In the first approach, various CP nanostructures, such as PEDOT nanostructures including nanorods, nanofibers, nanotubes (Hu et al., 2015b), and PPy nanotubes (Wu et al., 2014) have been systematically studied. A significant improvement in TE performance has been observed for these CPs-nanostructure composites as compared to the corresponding bulky CPs. However, the synthesis and fabrication of such nanostructures sometimes involve complicated synthesis and disproportionate scale-up, which limits their applications. The second approach has been widely investigated, which takes advantages of each respective component of the hybrid, high electric conductivity or high Seebeck coefficient, to “balance” the composite materials' properties (Zhang et al., 2010; Moriarty et al., 2013; Xu et al., 2013; Park et al., 2014a). Thus, an optimum power factor can be obtained via higher energy-filtering efficiency through the joint adjunct between nanoparticles and CPs (Choi et al., 2016). The highest ZT obtained so far is about <0.5 and mechanism for the hybrid materials is unfortunately not fully understood due to many challenging factors involved in the complicated system (Zhang et al., 2010; Kumar et al., 2018). The third approach is to tune the surface morphology through various doping approaches or post-treatments. In this approach, PEDOT:PSS has been the dominant CP to be extensively studied with various post-treatments (McGrail et al., 2015; Wei et al., 2015). The main aim for process or treatment is to remove the insulating polymer PSS from PEDOT:PSS. Various organic solvents (DMSO, EG and other chemicals) and inorganic salts have also been widely used to increase its electrical conductivity in a few magnitudes so that the power factor can be significantly increased (Zhang et al., 2010; Culebras et al., 2014a). Post-treatment by immersing the PEDOT:PSS films into EG or acid solution was also studied (Culebras et al., 2014a; Park et al., 2014b). The mechanism is well-studied and the phase separation of PEDOT is observed in several studies (Timpanaro et al., 2004; Crispin et al., 2006). Our previous modeling study also demonstrated that PSS-DMSO interaction was stronger than these in the PEDOT-PSS, PEDOT-DMSO and PSS-PSS interactions. Thus, dissolution of insulating PSS shells to release PEDOT conducting cores for self-aggregation is the main mechanism for the enhancement of electrical conductivity (Yildirim et al., 2018).
Aligning conducting polymers is a good method to tune carrier transport properties, and thus improve the thermoelectric properties. In order to align polymers, both common template synthesis and post-treatment methods have been investigated. Feng et al. reported the use of electrospinning and oxidative chemical polymerization to feasibly synthesize PEDOT fibers and tubes (Feng et al., 2013). Aligned PEDOT structures can be obtained through the template synthesis, but the whole process of the template synthesis is quite complicated and the template polymer has to be employed and removed in the fabrication. Another example is vapor-phase polymerization, in which an oxidant is used as a template and EDOT monomers stack via a bottom-up approach for well-ordered PEDOT crystals (Kim et al., 2007; Laforgue and Robitaille, 2010). Lee also proposed to post-treat a pre-fabricated PEDOT:PSS film with sulfuric acid. High-angle annular dark-field scanning transmission electron microscopy (HAADF-STEM) and X-ray powder diffraction (XRD) studies revealed that the PEDOT fibril structure was observed, resulting in a remarkably high electrical conductivity (4,380 S/cm). (Kim et al., 2014) Toward this goal, there seems no report using small molecules to induce the PEDOT:PSS polymer alignment. The major challenge of this is that the most commonly used molecules, such as DMSO, EG, acids and hydrazine are liquid which is unable to shape the polymer in a well-oriented way.
In this paper, we first identified a small molecule, dimethylsulfone (DMSO2), which is water-soluble and miscible with PEDOT:PSS well. DMSO2 is able to crystallize from the PEDOT:PSS system and indirectly align PEDOT:PSS through the hydrogen bonding interactions. Such alignment significantly increases electrical conductivity of PEDOT to 1080 S/cm, comparable to DMSO and EG, suggesting that DMSO2 is a green alternative to replace widely used DMSO and EG for solvent treatment of PEDOT:PSS. Furthermore, DMSO2 has several advantageous properties in term of non-toxicity, solid and high solubility in water. These properties make DMSO2 appropriate for wearable electronics devices that are required to direct contact with human skins.
Experiment Section
Materials
Microscopic glass slides (25 mm by 75 mm), dimethylsulfone (purity ≥98%) were purchased from Sigma Aldrich. PEDOT:PSS aqueous solution (PH1000, Heraeus Clevios) was purchased from Heraeus, Germany. All other solvents, such as EG, ethanol and distilled water were used as received without further purification.
Sample Preparation
Glass slides were first pre-treated by immersing in aqua regia for 2 days at ambient temperature to enhance its hydrophilic property. The glass slides were the further washed consecutively with distilled water, isopropanol, and acetone prior to being dried under air flow. Preparation of 1% DMSO2 in PEDOT:PSS is used as an example. Dimethylsulfone (4.0 mg) was added into a 2-mL sample vial containing 400.0 mg of PEDOT:PSS aqueous solution at ambient temperature. The pre-mixed solution was stirred under vortex for 5 min to ensure that DMSO2 is fully dissolved in PEDOT:PSS prior to ultrasonication for 20 min. The as-prepared solution was loaded onto the 2.5 × 2.5 cm2 pre-treated glass slide and left to stand for 5 min at ambient temperature. It was then further cured at 80°C for 40 min to obtain a thin film. In some cases, shrinkage was observed during the curing stage at 80°C; a glass pipette can be used to spread the solution so that a uniform film can be prepared.
Post-treatment
The as-prepared doped PEDOT:PSS film annealed on the glass substrate was immersed in solvents, such as water, methanol and ethanol respectively for 1 h at ambient temperature. The glass substrate together with the film was then carefully taken out and dried over the hotplate at 80°C for 30 min. The corresponding films obtained are then for the electrical conductivity measurements.
Characterization
The morphologies of the PEDOT:PSS films were studied using field emission scanning electron microscope (FESEM) on JSM6700F and atomic force microscopy (AFM) on Nanoscope IIIa instrument, Digital Instrument. XRD Measurement was performed on PANalytical X'Pert PRO High Resolution XRD. The electrical conductivity of PEDOT:PSS films were measured by Loresta-GP MCP-T600 (Mitsubishi Low Resistivity Meter) at room temperature. The thicknesses of the films were determined with a KLA Tencor P-16+ Surface Profiler. The Seebeck coefficient was measured by the custom-made measurement system equipped with an SA Peltier cooler (298 K - ΔT) and a Peltier heater (298 K + ΔT). Two microthermocouples (TCs) of 0.20 mm diameter were placed on the sample abreast of two electrodes connected to a Keithley 2400 source meter. The Seebeck coefficient was estimated by a linear fit to the measured ΔV vs. ΔT at different electrode spaces.
Calculation of Interaction Energies
Pairwise interaction energies between PEDOT:PSS components and solvents are critical for elaborating molecular mechanism of solvent treatment in PEDOT:PSS. Pairwise interactions are calculated between solvents and components of PEDOT:PSS. The solvents include DMSO2, DMSO, EG and N-methyl pyrrolidone (NMP), while the components of PEDOT:PSS are neutral PEDOT trimer, polystyrene sulfonic acid trimer (PSSH), negatively charged PSS− trimer with one deprotonated PSS monomer, and positively charged radical PEDOT+ trimer in the polaron state. Trimer structures were used for the PSS and the PEDOT, since it was experimentally determined that three PEDOT monomers possess one positive charge (Volkov et al., 2017). Initial structure pairs for density functional theory (DFT) calculations were determined by generating a large number of molecular configurations with excluded-volume constraints to determine energetically favorable configurations by employing statistical mechanics techniques (Fan et al., 1992). At least 12 lowest energy structures were determined for each of binary interactions between PEDOT and DMSO2. DFT calculations were performed by using M06-2X (Zhao and Truhlar, 2008) functional with 6-31+g(d) basis set in Gaussian16.A.03 software (Frisch et al., 2019). Both vacuum and implicit solvent methods for water are considered during the calculations. IEF-PCM implicit water continuum model is used to represent water solution (Tomasi et al., 2005).
Free Energy of Solvation (ΔGsol) Calculations
Free energies of solvation (ΔGsol) were calculated for DMSO2, and solutes in DMSO, EG, tetrahydrofuran (THF), acetone, N,N-dimethyl formamide (DMF), NMP, CH2Cl2, hexane, MeOH and water solvents under periodic boundary conditions based on the coupling parameter method and thermodynamic integration algorithm (Figure S3) (Steinbrecher et al., 2011) The free energy of solvation was determined in three steps. Firstly, we computed the ideal free of energy of solvation (ΔGid) by determining the free energy change associated with the discharge of the solute (DMSO2, , and ) in vacuum. Then the neutral solute molecule was brought into contact with the solvent molecules; the free energy change for this step is called vdW free energy of solvation (ΔGvdw). Lastly, the solvated and discharged solute molecules are charged again in solvent to determine the electrostatic free energy of solvation (ΔGel) (Biovia, 2017). Total free energy of solvation (ΔGsol) was calculated as the sum of the free energy contributions from ideal, vdW and electrostatic free energies of solvation (Equation 1), noting that experimental densities were used for the solvents in free energy calculations.
COMPASS (Condensed-Phase Optimized Molecular Potentials for Atomistic Simulation Studies) force field was used to validate solubility and phase properties of polymers in solution (Sun, 1998; Yildirim et al., 2015).
Results and Discussions
Figure 1 shows some common additives including DMSO, EG, NMP, and DMSO2 used in the PEDOT:PSS for electrical conductivity enhancement. DMSO, EG, and NMP are in liquid forms at room temperature with high boiling point. During the process of film curing, high temperature has to be employed to vaporize these additives into ambient environment. To overcome this disadvantage, DMSO2 is screened as a potential dopant. Unlike DMSO, EG and NMP, DMSO2 is a solid and the sulfur atom in the molecule is at its highest oxidation state, making it more chemically stable. The fabrication process for PEDOT:PSS with DMSO2 via drop-cast is shown in Figure 2, which is very similar to that of the commonly used DMSO, EG (Zhang et al., 2010) and other inorganic additives (Culebras et al., 2014a). It includes mixing PEDOT:PSS with additives, drop-cast of the solution onto the glass substrate and curing at a given temperature. When DMSO and inorganic acids are used to dope PEDOT:PSS, a closed encasement or a fume-hood is required for the film preparation and curing process due to toxic and hazardous vapor caused by these volatile solvents. However, the processing and fabrication of solid DMSO2 doped PEDOT films do not require a confined environment as no hazardous solvent vapor is produced. This opens up a new means to fabricate PEDOT:PSS films, which reduces the potential damage to human health and also lowers the fabrication cost. Similarly, electronic devices employing PEDOT:PSS and DMSO2 as an additive would be much safer to consumers, particularly in the event that demands the stringent safety regulations such as wearable devices.
The thermoelectric performance in terms of electrical conductivity with respect to DMSO2 concentration was measured and results are shown in Figure 3. The pristine PEDOT:PSS gave an electrical conductivity of 0.2 S/cm. With the increase in DMSO2 loading, the electrical conductivity gradually increased and reached close to 1,100 S/cm. When over 3% additive loading was added, the decrease in electrical conductivity was observed, possibly due to the un-evenness of as-prepared film at high additive loading. Another plausible reason is that DMSO2 play a somewhat insulation role if more loading of it applies, leading to the reduction in the electrical conductivity. This trend of electrical conductivity with respect to the amount of DMSO2 added is very similar to known DMSO, EG, NMP and others dopants. Interestingly, the electrical conductivity of PEDOT:PSS remained almost constant despite curing temperatures between 60-120°C, showing more advantages than processes using NMP or EG, which require a higher temperature and a much longer curing time to vaporize out the high boiling-point additives. The Seebeck coefficient was also measured and data are summarized in Figure 3. The Seebeck coefficient shows an overall decreasing trend with the increase of loading of DMSO2, and a highest Seebeck coefficient of 23 μV/K was achieved at 1% loading of DMSO2. The carrier concentration usually leads to a proportional increment of electrical conductivity. However, the Seebeck coefficient varies inversely due to the trade-off relationship between these two parameters. (Yao et al., 2010) The power factor increased sharply at a DMSO2 loading of <3%. At a higher DMSO2 loading, the power factor dropped. A highest power factor of 32 μW/mK2 was obtained at 3% loading of DMSO2. The figure of merit (ZT) was approximately estimated to be 0.02 assuming that the thermal conductivity of PEDOT is 0.54 Wm−1K−1 (Kyaw et al., 2017). The current ZT value is smaller than those reported (Table S1), but it is believed that it could be greatly improved if the further post-treatment that is able to reduce the doping level is performed to increase its Seebeck coefficient.
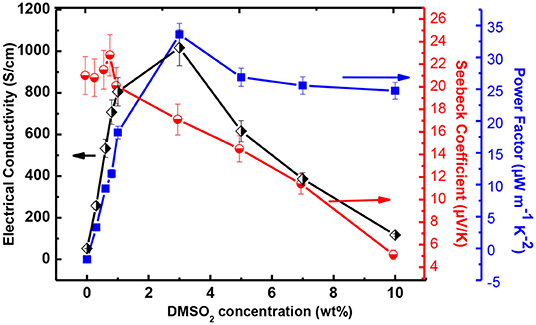
Figure 3. The electrical conductivity, Seebeck coefficient and power factor at ambient temperature for DMSO2 doped PEDOT:PSS at different loading.
AFM and FESEM were used to understand the interaction between sulfone and PEDOT:PSS. AFM images were taken to observe the roughness for pristine and DMSO2-doped PEDOT:PSS films (Figure 4). Similar to the observation by Alshareef (Kumar et al., 2016), the rms roughness for pristine PEDOT:PSS is 1.8 nm (Figure 4A), showing very smooth and uniform film as prepared. Upon adding DMSO2 into PEDOT:PSS at 1, 3, and 5% loading (Figures 4C,E,G), film roughness is ascending with the similar trend. At 1% loading (Figure 4C), the roughness was 4 nm with tiny fibrous structures observed by topography and phase images. Accordingly, 5.0 and 5.3 nm and 8.1 nm roughness were observed by careful examination on AFM images, showing that tiny crystal-like bright rods with regular shape was observed in their phase images (Figures 4B,D,F,H). The bright rods could be the uniform crystals which were formed by DMSO2 during the curing process. Unlike conventional additives, such as liquid DMSO, EG, and NMP, DMSO2 is in a solid form, and more chemically stable as the sulfur atom in DMSO2 is fully oxidized.
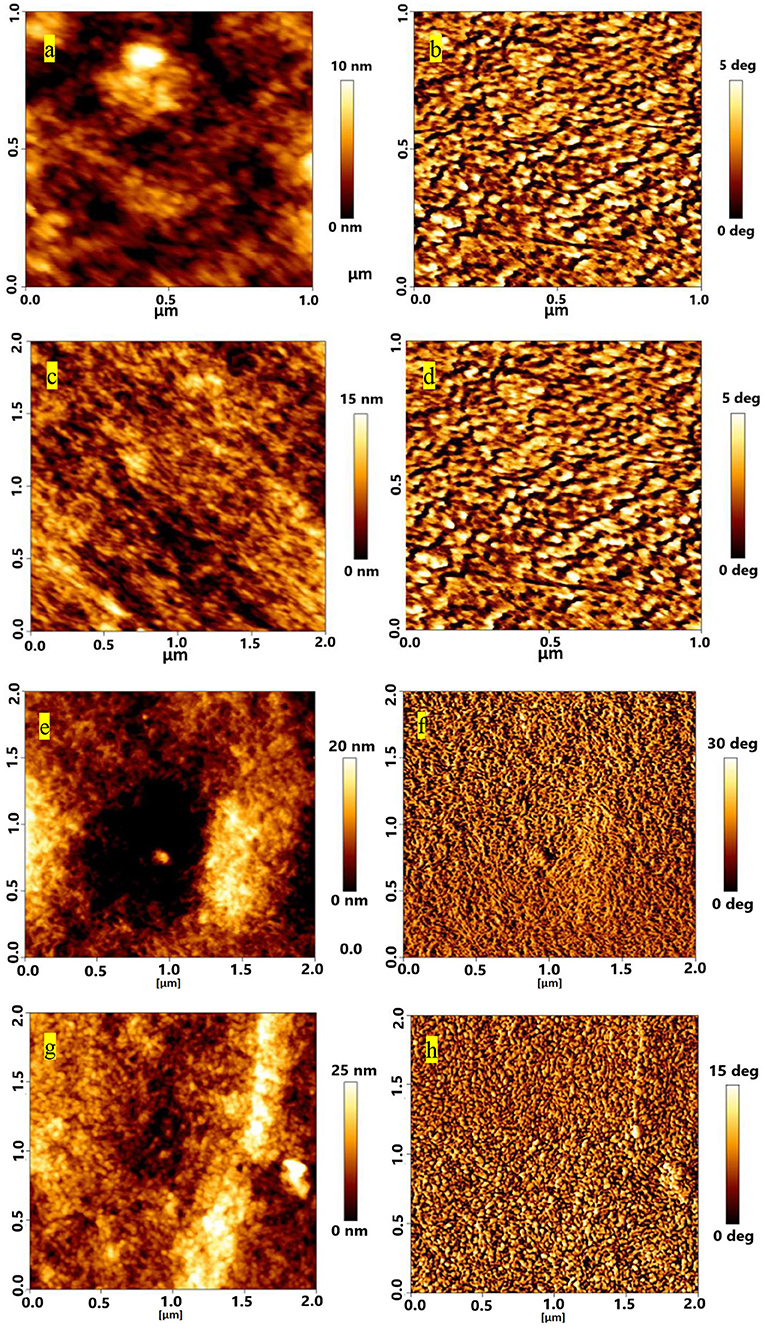
Figure 4. AFM topography images of different loading of DMSO2 in PEDOT:PSS films for (a) pristine (c) 1% (e) 3% and (g) 5%; corresponding phase images are shown on right panels (b,d,f,h).
FESEM images were used to examine the surface morphology for all the films doped with sulfone (Figure 5). Interestingly, a clear long fibrous structure embedded in the surface was found in the film prepared by 3% DMSO2 in PEDOT:PSS. Such a long crystal structure helped well-orientation of the PEDOT:PSS backbone so that carriers can move in a more efficient way, resulting in higher electrical conductivity. In literature, three approaches have been used to enhance electrical conductivity of PEDOT:PSS. The first was the de-doping process through tuning the charge carrier concentration along the polymer chains. Bubnova et al. reported the use of tetrakis(dimethylamino)ethylene to tune the electron distribution along PEDOT:p-toluenesulfonate (PEDOT:Tos) to enhance electrical conductivity significantly (Bubnova et al., 2011; Tomlinson et al., 2015). The second approach is the secondary doping with organic solvents or counter-ions so as to tune the polymer chain conformation. For example, when DMSO or EG is employed in PEDOT:PSS solution, the strong Van der Waals interactions between the polar group of DMSO/EG and PEDOT:PSS chains can lead to a structural change from a benzoid to a quinoid structure in the polymer chains, resulting in a few magnitude of electrical conductivity enhancement (Jiang et al., 2008; Yue et al., 2012). The third approach is to remove the excess of insulation polymer, PSS, in the PEDOT:PSS system through post-treatment with DMSO, EG or acids (Kim et al., 2013). In contrast, in our DMSO2 doped PEDOT:PSS system, the electrical conductivity enhancement is similarly due to the polymer alignment but induced by in-situ crystallization of solid DMSO2 dopant as observed by microscopy images.
To further verify the polymer alignment of PEDOT:PSS induced by DMSO2 crystallization, the X-ray diffraction (XRD) is used to check the diffraction of the pristine, DMSO2-doped PEDOT:PSS and pure DMSO2 crystals (Figure 6). Four characteristic peaks: 2θ = 3.7°, 6.6°, 17.7°, and 26.0° were observed on pristine sample which is consistent with the recent work published by Lee et al. (Kim et al., 2014) The low diffraction at 3.7°, 6.6° and high diffraction at 17.7°, and 26.0° was attributed to the lamella stacking between two distinct alternate ordering PEDOT/PSS and inter-chain ring stacking between PEDOT:PSS, respectively. Due to the random orientation of polymer chains in the pristine PEDOT:PSS, it can be observed that much stronger diffraction at 6.6° than at 3.7° is observed, and the intensity ratio between the diffractions of 6.6 and 3.7° is 1.50. XRD shows that the diffraction at 6.6° is significantly reduced when DMSO2 was loaded into PEDOT:PSS, while the diffraction at 3.7, 17.7, and 26.0° remained relatively unchanged. More importantly, the intensity ratio between the diffraction of 6.6 and 3.7° become 0.49. This observation demonstrates that the polymers orient in a more ordered way toward one particular lamella stacking between the two distinct alternate ordering of PEDOT:PSS.
Meantime, the XRD diffraction patterns for pure DMSO2 crystal are also measured for comparison. Characteristic diffraction peaks at 17.2°, 20.5°, 24.0, 26.1 and 32.5° were observed. However, when doped with PEDOT:PSS, no diffraction signals are observed indicating that the DMSO2 crystal is wrapped by polymer chains, which is consistent with AFM studies (Figure 4). In other words, PEDOT:PSS is aligned in a more ordered manner, which is indirectly induced by DMSO2 crystals wrapped by PEDOT:PSS.
Pairwise interaction energies were analyzed to explain observed enhancement in thermoelectric properties. Optimized structures for the PSSH with DMSO2, DMSO, EG and NMP are given in Figures 7A–D, where interactions with the PSSH are found to follow the order of DMSO > DMSO2 > NMP > EG. Hydrogen bonding is the main interactions, e.g., oxygen atoms in both DMSO and DMSO2 would form hydrogen bonds with the –S-O-H in sulfonic acid group of the PSSH. In addition to strong hydrogen bonding, methyl hydrogens in DMSO and DMSO2 interact and surround the two other sulfonate oxygen atoms in the PSSH, leading to the –SO3H groups in the PSSH to diminish interactions with other PSS and PEDOT chains. Therefore, separation of the excess insulating PSSH shell from the PSS− doped PEDOT+. chains is believed to be a main mechanism. On the other hand, stronger hydrogen bonding interaction is not possible between the deprotonated PSS− anion and DMSO and DMSO2, which consist mainly of relatively weaker –CH…O− interaction. Hence, we found that the PSSH had much stronger interaction with all solvents compared to the PSS− except for EG; EG solvent can form two strong hydrogen bonds with the deprotonated PSS−. The difference in the solvation mechanism between EG and DMSO explains why big electrical conductivity enhancement was observed by co-solvent treatment in previous studies (Shi et al., 2015).
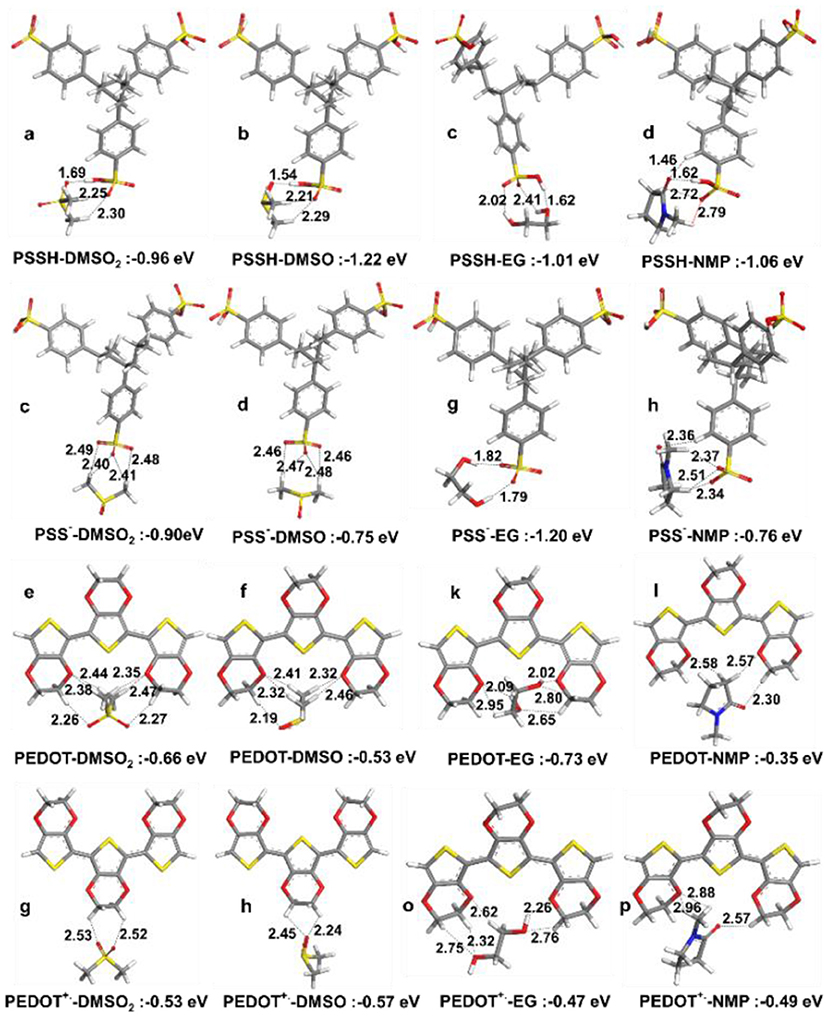
Figure 7. Optimized lowest energy structures and interaction energies in vacuum for (a–d) PSSH trimer with DMSO2, DMSO, EG, and NMP, (e–h) PSS− trimer with DMSO2, DMSO, EG and NMP, (i–l) PEDOT trimer with DMSO2, DMSO, EG, and NMP, (m–p) PEDOT+. trimer with DMSO, DMSO2, EG, and NMP.
Neither PEDOT nor PEDOT+. in the polaron state can form any hydrogen bonds with solvent molecules, and therefore their interactions with solvents are generally much weaker, where solvent molecules prefer to interact with oxygen atoms in the dioxyethylene group (-OCH2CH2O-) and solvent oxygen atoms weakly interact with the –CH moieties of PEDOT. This implies that solvents are mainly interacting with the PSS shell, leaving the conductive PEDOT core to remain intact. The most important consequence here is that the lowest energy structures suggest similar enhancement mechanism and comparable performance for DMSO and DMSO2. The interactions are weaker than under water solvation effect, showing the advantage of post treatment compared to solution treatment.
For solvent interactions with single PSSH, PSSH-DMSO has stronger interaction compared to DMSO2. However, DMSO2 with two oxygen atoms is bifunctional and thus is able to coordinate with two PSSH monomers (see Figure 8 for solvent interactions). DMSO and DMSO2 have higher interaction energies with the PSSH compared to EG and NMP. The difference among solvents is lowered under implicit water solvation effect for ternary interaction. According to the calculated interaction energies, the PSSH-solvent interaction can have two effects on the system, i.e., (i) chain expansion and phase separation of the PSSH shell as a result of decreasing PSS-PSS interactions, and (ii) charge screening and phase separation of PSS-PEDOT. Partial reduction of the excess PSSH shell that covers the conducting PEDOT components enhances the connectivity between PEDOT phases, directly resulting in the enhancement of electrical conductivity.
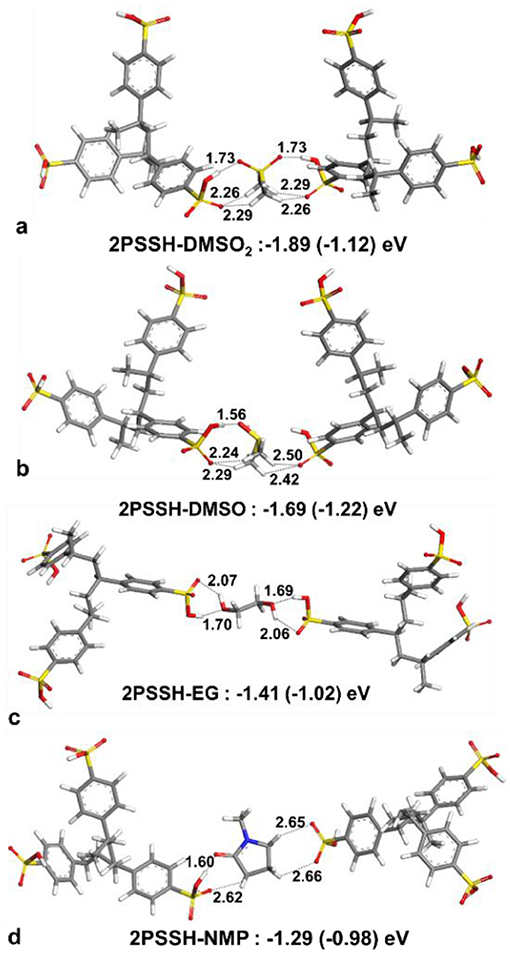
Figure 8. Optimized lowest energy structures and interaction energies of two PSSH trimers with (a) DMSO2, (b) DMSO, (c) EG, and (d) NMP. Interaction energies calculated with water solvation effect are given in parenthesis.
Based on AFM, FESEM, and XRD and theoretical studies, we propose a mechanism for electrical conductivity enhancement by DMSO2 in Figure 9, illustrating a schematic for the role of DMSO2 in doping PEDOT:PSS and polymer orientation arising from the crystallization. When water molecules slowly evaporate upon heating, the DMSO2 concentration increases and thus its self-interaction leads to the formation of fibrous crystals. Such a fibrous structure triggers the alignment of long PSS chains resulting in well-orientation of PEDOT and better connection between PEDOT units absorbed to PSS chain to improve the electrical conductivity. In literature, it has well-reported that the PSS insulating polymer can be separated through DMSO, EG, or acid treatment (Culebras et al., 2014a; Park et al., 2014b).
Free energy of solvation calculations were performed in periodic cells for the PSS, PEDOT, and DMSO2 solvation by organic solvents to understand the observed variation in structural and electrical conductivity properties (Figure 10). Since all the calculated solvation free energies are negative, mixing is expected in all cases. The highest |ΔGsol| is observed for the solvation of the PSS chain (across almost all solvents), proving that the mechanism of conductivity enhancement via the PSS phase separation. Hexane, which has the lowest |ΔGsol| around the PSS chain and DMSO2 molecules, does not have any effect on the electrical conductivity and film thickness. THF and CH2Cl2 solvents, which have high |ΔGsol| for PEDOT chains and good miscibility with the PSS chains, have low electrical conductivity and big thickness due to polymer expansion. The solvents that enhance electrical conductivity of PEDOT:PSS films treated with DMSO2 should thus have high values of |ΔGsol| for either PSS solvation or DMSO2 solvation. Besides, ideal solvent should have lower solvation free energy for PEDOT as a solute. As an example, DMSO can wash away insulating DMSO2 crystals in PEDOT:PSS, decrease thickness of films and enhance electrical conductivity. DMSO has similar electrical conductivity enhancement mechanism with DMSO2. That is the reason why further electrical conductivity enhancement is limited. Acetone also have similar effect with strong solvation of DMSO2, decreasing thickness and slightly enhances the conductivity. Solvents that have strong solvation of both DMSO2 and the PSS chains, such as EG and DMF should simultaneously decrease film thickness and enhance electrical conductivity. The mechanism of solvent interaction with the PSS chains is important so that secondary solvent with different interaction mechanism with the PSS chains compared to DMSO2 can separate the remaining PSS chains to enhance conductivity further.
In order to obtain the electric properties of PEDOT:PSS films with different concentration of DMSO2, the Van der Pauw–Hall measurements were performed. Table 1 lists the carrier mobility (μ), concentration (n) and conductivity of PEDOT:PSS with DMSO2. The carrier mobility improved significantly with the increase of the concentration of additive in PEDOT:PSS film. It is reasonable to conclude that the enhancement in electric conductivity of PEDOT:PSS is due to the incorporation of DMSO2. The carrier concentration increases with the increase of additive concentrations but slightly decrease when the concentration of DMSO2 is over 3%. As DMSO2 does not behave like a reducing agent to cause the change in the oxidation level of PEDOT. The increase in carrier concentration is probably due to the release of trapped carriers by polymer chains. DMSO2 treatment induces the polymer alignment along a particular direction and thus pushes out the carriers from the polymer chains entangled with the PSSH. On the other hand, DMSO2 with two oxygen atoms is bifunctional and thus is able to coordinate with two PSSH monomers. Based on the modeling (vide supra) partial reduction of the excess PSSH shell that covers the conducting PEDOT components enhances the connectivity between PEDOT phases, as a result, perhaps freeing more carriers and increasing the electric conductivity.
In addition, the long-term stability for our pre-mixed PEDOT:PSS and DMSO2 solution is also investigated. Chemical de-doping with tetrakis(dimethylamino)ethylene, secondary doping with polar organic solvents like DMSO, EG and NMP have been used to obtain high electrical conductivity. However, it is known that most mixtures of PEDOT:PSS and solvent dopants cannot be stored for a long duration. Figure 11 shows that pre-mixed solution of DMSO2 and PEDOT:PSS can be stored at 2–6°C for up to 2 months without significant change of the electrical conductivity, benefiting many applications.
Conclusion
As an alternative to conventional additives, DMSO2 was identified as a dopant for the PEDOT: PSS to raise the electrical conductivity from 0.2 to 1080 S/cm with 3% loading. The enhancement in electrical conductivity is due to polymer alignment induced by crystallization of DMSO2 in the PEDOT:PSS system. The modeling study revealed that the interactions at the molecular level demonstrated that DMSO2 had comparable interaction energies with conventional solvents, which is responsible for conductivity enhancement. This new additive has an edge over other traditional dopants/additives as it is environmentally friendly, non-toxic, and has an easy doping process without additional treatment. Furthermore, PEDOT:PSS doped with DMSO2 is stable and “green,” making it highly desired for industrial applications. DMSO2 is unable to contribute to improvement in the Seebeck coefficient due to the inability of DMSO2 to change the oxidation level of PEDOT. Our next step would be to introduce an additional post-treatment that enables to enhance the Seebeck coefficient, and thus leads to the high power factor of PEDOT.
Data Availability Statement
All datasets generated for this study are included in the article/Supplementary Material.
Author Contributions
QZ, and JX conceived and designed the experiments. QZ, YZ, XW, and XS performed the experiments and contributed to the film fabrication, measurement, and data analysis. EY, TT, GW, and S-WY contributed to modeling and analysis. QZ, EY, and JX wrote and revised the paper.
Funding
This work was financially supported by the Agency for Science, Technology and Research (A*STAR), Industry Alignment Fund, Pharos Hybrid thermoelectric materials for ambient applications Program (Grant Nos.: 1527200019, 1527200021, and 1527200024).
Conflict of Interest
The authors declare that the research was conducted in the absence of any commercial or financial relationships that could be construed as a potential conflict of interest.
Acknowledgments
The authors would like to acknowledge the support from the Institute of Materials Research and Engineering (IMRE), Agency for Science, Technology and Research (A*STAR).
Supplementary Material
The Supplementary Material for this article can be found online at: https://www.frontiersin.org/articles/10.3389/fchem.2019.00783/full#supplementary-material
References
Biovia, D. S. (2017). BIOVIA Discovery Studio 2017 R2: A Comprehensive Predictive Science Application for the Life Sciences. San Diego, CA. Available online at: http://accelrys.com/products/collaborative-science/biovia-discovery-studio
Bubnova, O., Khan, Z. U., Malti, A., Braun, S., Fahlman, M., Berggren, M., et al. (2011). Optimization of the thermoelectric figure of merit in the conducting polymer poly (3, 4-ethylenedioxythiophene). Nat. Mater. 10, 429–433. doi: 10.1038/nmat3012
Choi, J., Lee, J. Y., Lee, S. S., Park, C. R., and Kim, H. (2016). High-performance thermoelectric paper based on double carrier-filtering processes at nanowire heterojunctions. Adv. Energy Mater. 6:1502181. doi: 10.1002/aenm.201502181
Choy, C. L. (1977). Thermal conductivity of polymers. Polymer 18, 984–1004. doi: 10.1016/0032-3861,90002-7
Crispin, X., Jakobsson, F., Crispin, A., Grim, P., Andersson, P., Volodin, A., et al. (2006). The origin of the high conductivity of poly (3, 4-ethylenedioxythiophene)-poly (styrenesulfonate)(PEDOT-PSS) plastic electrodes. Chem. Mater. 18, 4354–4360. doi: 10.1021/cm061032+
Culebras, M., Gomez, C., and Cantarero, A. (2014a). Enhanced thermoelectric performance of PEDOT with different counter-ions optimized by chemical reduction. J. Mater. Chem. A 2, 10109–10115. doi: 10.1039/C4TA01012D
Culebras, M., Gómez, C. M., and Cantarero, A. (2014b). Review on polymers for thermoelectric applications. Materials 7, 6701–6732. doi: 10.3390/ma7096701
Fan, C. F., Olafson, B. D., Blanco, M., and Hsu, S. L. (1992). Application of molecular simulation to derive phase diagrams of binary mixtures. Macromolecules 25, 3667–3676. doi: 10.1021/ma00040a010
Feng, Z.-Q., Wu, J., Cho, W., Leach, M. K., Franz, E. W., Naim, Y. I., et al. (2013). Highly aligned poly (3, 4-ethylene dioxythiophene)(PEDOT) nano-and microscale fibers and tubes. Polymer 54, 702–708. doi: 10.1016/j.polymer.2012.10.057
Frisch, M., Trucks, G., Schlegel, H., Scuseria, G., Robb, M., Cheeseman, J., et al. (2019). Gaussian 16, Revision A. Wallingford, CT: Gaussian, Inc.
Han, S., Zhai, W., Chen, G., and Wang, X. (2014). Morphology and thermoelectric properties of graphene nanosheets enwrapped with polypyrrole. RSC Adv. 4, 29281–29285. doi: 10.1039/C4RA04003A
Hu, F., Cai, Q., Liao, F., Shao, M., and Lee, S.-T. (2015a). Recent advancements in nanogenerators for energy harvesting. Small 11, 5611–5628. doi: 10.1002/smll.201501011
Hu, X., Chen, G., Wang, X., and Wang, H. (2015b). Tuning thermoelectric performance by nanostructure evolution of a conducting polymer. J. Mater. Chem. A 3, 20896–20902. doi: 10.1039/C5TA07381B
Hu, Y., Shi, H., Song, H., Liu, C., Xu, J., Zhang, L., et al. (2013). Effects of a proton scavenger on the thermoelectric performance of free-standing polythiophene and its derivative films. Synth. Met. 181, 23–26. doi: 10.1016/j.synthmet.2013.08.006
Jiang, F.-X., Xu, J.-K., Lu, B.-Y., Xie, Y., Huang, R.-J., and Li, L.-F. (2008). Condensed matter: electronic structure, electrical, magnetic, and optical properties: thermoelectric performance of poly (3, 4-ethylenedioxythiophene): poly (styrenesulfonate). Chin. Phys. Lett. 25, 2202–2205. doi: 10.1088/0256-307X/25/6/076
Kaneko, H., Ishiguro, T., Takahashi, A., and Tsukamoto, J. (1993). Magnetoresistance and thermoelectric power studies of metal-nonmetal transition in iodine-doped polyacetylene. Synth. Met. 57, 4900–4905. doi: 10.1016/0379-6779(93)90836-L
Kemp, N., Kaiser, A., Liu, C. J., Chapman, B., Mercier, O., Carr, A., et al. (1999). Thermoelectric power and conductivity of different types of polypyrrole. J. Polym. Sci. Part B: Polym. Phys. 37, 953–960.
Kim, G. H., Shao, L., Zhang, K., and Pipe, K. P. (2013). Engineered doping of organic semiconductors for enhanced thermoelectric efficiency. Nat. Mater. 12, 719–723. doi: 10.1038/nmat3635
Kim, J. Y., Kwon, M. H., Min, Y. K., Kwon, S., and Ihm, D. W. (2007). Self-Assembly And Crystalline Growth Of Poly (3, 4-Ethylenedioxythiophene) Nanofilms. Adv. Mater. Weinheim. 19, 3501–3506. doi: 10.1002/adma.200602163
Kim, N., Kee, S., Lee, S. H., Lee, B. H., Kahng, Y. H., Jo, Y. R., et al. (2014). Highly Conductive PEDOT: PSS Nanofibrils Induced by Solution-Processed Crystallization. Adv. Mater. Weinheim. 26, 2268–2272. doi: 10.1002/adma.201304611
Kumar, P., Zaia, E. W., Yildirim, E., Repaka, D. V. M., Yang, S.-W., Urban, J. J., et al. (2018). Polymer morphology and interfacial charge transfer dominate over energy-dependent scattering in organic-inorganic thermoelectrics. Nat. Commun. 9:5347. doi: 10.1038/s41467-018-07435-z
Kumar, S. R. S., Kurra, N., and Alshareef, H. N. (2016). Enhanced high temperature thermoelectric response of sulphuric acid treated conducting polymer thin films. J. Mater. Chem. C 4, 215–221. doi: 10.1039/C5TC03145A
Kyaw, A. K. K., Yemata, T. A., Wang, X., Lim, S. L., Chin, W. S., Hippalgaonkar, K., et al. (2017). Enhanced thermoelectric performance of PEDOT:PSS films by sequential post-treatment with formamide. Macromol. Mater. Eng. 303:1700429. doi: 10.1002/mame.201700429
Laforgue, A., and Robitaille, L. (2010). Production of conductive PEDOT nanofibers by the combination of electrospinning and vapor-phase polymerization. Macromolecules 43, 4194–4200. doi: 10.1021/ma9027678
Mateeva, N., Niculescu, H., Schlenoff, J., and Testardi, L. (1998). Correlation of Seebeck coefficient and electric conductivity in polyaniline and polypyrrole. J. Appl. Phys. 83, 3111–3117. doi: 10.1063/1.367119
McGrail, B. T., Sehirlioglu, A., and Pentzer, E. (2015). Polymer composites for thermoelectric applications. Angew. Chem. Int. Ed. 54, 1710–1723. doi: 10.1002/anie.201408431
Moriarty, G. P., Briggs, K., Stevens, B., Yu, C., and Grunlan, J. C. (2013). Fully organic nanocomposites with high thermoelectric power factors by using a dual-stabilizer preparation. Energy Technol. 1, 265–272. doi: 10.1002/ente.201300018
Park, G. O., Roh, J. W., Kim, J., Lee, K. Y., Jang, B., Lee, K. H., et al. (2014a). Enhanced thermoelectric properties of germanium powder/poly (3, 4-ethylenedioxythiophene): poly (4-styrenesulfonate) composites. Thin Solid Films 566, 14–18. doi: 10.1016/j.tsf.2014.07.011
Park, H., Lee, S. H., Kim, F. S., Choi, H. H., Cheong, I. W., and Kim, J. H. (2014b). Enhanced thermoelectric properties of PEDOT: PSS nanofilms by a chemical dedoping process. J. Mater. Chem. A 2, 6532–6539. doi: 10.1039/C3TA14960A
Park, T., Park, C., Kim, B., Shin, H., and Kim, E. (2013). Flexible PEDOT electrodes with large thermoelectric power factors to generate electricity by the touch of fingertips. Energy Environ. Sci. 6, 788–792. doi: 10.1039/c3ee23729j
Shi, H., Liu, C., Jiang, Q., and Xu, J. (2015). Effective approaches to improve the electrical conductivity of PEDOT: PSS: a review. Adv. Electron. Mater. 1:1500017. doi: 10.1002/aelm.201500017
Steinbrecher, T., Joung, I., and Case, D. A. (2011). Soft-core potentials in thermodynamic integration: comparing one-and two-step transformations. J. Comput. Chem. 32, 3253–3263. doi: 10.1002/jcc.21909
Sun, H. (1998). COMPASS: An ab initio force-field optimized for condensed-phase applications overview with details on alkane and benzene compounds. J. Phys. Chem. B 102, 7338–7364. doi: 10.1021/jp980939v
Timpanaro, S., Kemerink, M., Touwslager, F., De Kok, M., and Schrader, S. (2004). Morphology and conductivity of PEDOT/PSS films studied by scanning–tunneling microscopy. Chem. Phys. Lett. 394, 339–343. doi: 10.1016/j.cplett.2004.07.035
Tomasi, J., Mennucci, B., and Cammi, R. (2005). Quantum mechanical continuum solvation models. Chem. Rev. 105, 2999–3094. doi: 10.1021/cr9904009
Tomlinson, E. P., Willmore, M. J., Zhu, X., Hilsmier, S. W., and Boudouris, B. W. (2015). Tuning the thermoelectric properties of a conducting polymer through blending with open-shell molecular dopants. ACS Appl. Mater. Interfaces 7, 18195–18200. doi: 10.1021/acsami.5b05860
Volkov, A. V., Wijeratne, K., Mitraka, E., Ail, U., Zhao, D., Tybrandt, K., et al. (2017). Understanding the capacitance of PEDOT:PSS. Adv. Funct. Mater. 27:1700329. doi: 10.1002/adfm.201700329
Wei, Q., Mukaida, M., Kirihara, K., Naitoh, Y., and Ishida, T. (2015). Recent progress on PEDOT-based thermoelectric materials. Materials 8, 732–750. doi: 10.3390/ma8020732
Wu, J., Sun, Y., Pei, W.-B., Huang, L., Xu, W., and Zhang, Q. (2014). Polypyrrole nanotube film for flexible thermoelectric application. Synth. Met. 196, 173–177. doi: 10.1016/j.synthmet.2014.08.001
Xu, K., Chen, G., and Qiu, D. (2013). Convenient construction of poly (3, 4-ethylenedioxythiophene)–graphene pie-like structure with enhanced thermoelectric performance. J. Mater. Chem. A 1, 12395–12399. doi: 10.1039/c3ta12691a
Yao, Q., Chen, L., Zhang, W., Liufu, S., and Chen, X. (2010). Enhanced thermoelectric performance of single-walled carbon nanotubes/polyaniline hybrid nanocomposites. ACS Nano 4, 2445–2451. doi: 10.1021/nn1002562
Yildirim, E., Wu, G., Yong, X., Tan, T. L., Zhu, Q., Xu, J., et al. (2018). A theoretical mechanistic study on electrical conductivity enhancement of DMSO treated PEDOT:PSS. J. Mater. Chem. C 6, 5122–5131. doi: 10.1039/C8TC00917A
Yildirim, E., Zhang, Y., Lutkenhaus, J. L., and Sammalkorpi, M. (2015). Thermal transitions in polyelectrolyte assemblies occur via a dehydration mechanism. ACS Macro Lett. 4, 1017–1021. doi: 10.1021/acsmacrolett.5b00351
Yoon, C., Reghu, M., Moses, D., Cao, Y., and Heeger, A. (1995). Transports in blends of conducting polymers. Synth. Met. 69, 255–258. doi: 10.1016/0379-6779(94)02439-6
Yue, R., Chen, S., Liu, C., Lu, B., Xu, J., Wang, J., et al. (2012). Synthesis, characterization, and thermoelectric properties of a conducting copolymer of 1, 12-bis (carbazolyl) dodecane and thieno [3, 2-b] thiophene. J. Solid State Electrochem. 16, 117–126. doi: 10.1007/s10008-011-1292-0
Zhang, B., Sun, J., Katz, H., Fang, F., and Opila, R. (2010). Promising thermoelectric properties of commercial PEDOT: PSS materials and their Bi2Te3 powder composites. ACS Appl. Mater. Interfaces 2, 3170–3178. doi: 10.1021/am100654p
Zhao, D., and Tan, G. (2014). A review of thermoelectric cooling: materials, modeling and applications. Appl. Therm. Eng. 66, 15–24. doi: 10.1016/j.applthermaleng.2014.01.074
Zhao, Y., and Truhlar, D. G. (2008). The M06 suite of density functionals for main group thermochemistry, thermochemical kinetics, noncovalent interactions, excited states, and transition elements: two new functionals and systematic testing of four M06-class functionals and 12 other functionals. Theor. Chem. Acc. 120, 215–241. doi: 10.1007/s00214-007-0310-x
Keywords: dimethylsulfone, PEDOT:PSS, thermoelectric, polymer alignment, crystallization
Citation: Zhu Q, Yildirim E, Wang X, Soo XYD, Zheng Y, Tan TL, Wu G, Yang S-W and Xu J (2019) Improved Alignment of PEDOT:PSS Induced by in-situ Crystallization of “Green” Dimethylsulfone Molecules to Enhance the Polymer Thermoelectric Performance. Front. Chem. 7:783. doi: 10.3389/fchem.2019.00783
Received: 14 July 2019; Accepted: 30 October 2019;
Published: 15 November 2019.
Edited by:
Sumeet Walia, RMIT University, AustraliaReviewed by:
Ayaskanta Sahu, New York University, United StatesSyed Mubeen Jawahar Hussaini, The University of Iowa, United States
Copyright © 2019 Zhu, Yildirim, Wang, Soo, Zheng, Tan, Wu, Yang and Xu. This is an open-access article distributed under the terms of the Creative Commons Attribution License (CC BY). The use, distribution or reproduction in other forums is permitted, provided the original author(s) and the copyright owner(s) are credited and that the original publication in this journal is cited, in accordance with accepted academic practice. No use, distribution or reproduction is permitted which does not comply with these terms.
*Correspondence: Shuo-Wang Yang, eWFuZ3N3JiN4MDAwNDA7aWhwYy5hLXN0YXIuZWR1LnNn; Jianwei Xu, ancteHUmI3gwMDA0MDtpbXJlLmEtc3Rhci5lZHUuc2c=