- École Polytechnique Fédérale de Lausanne, Lausanne, Switzerland
Optical sensors based on single-walled carbon nanotubes (SWCNTs) demonstrate tradeoffs that limit their use in in vivo and in vitro environments. Sensor characteristics are primarily governed by the non-covalent wrapping used to suspend the hydrophobic SWCNTs in aqueous solutions, and we herein review the advantages and disadvantages of several of these different wrappings. Sensors based on surfactant wrappings can show enhanced quantum efficiency, high stability, scalability, and diminished selectivity. Conversely, sensors based on synthetic and bio-polymer wrappings tend to show lower quantum efficiency, stability, and scalability, while demonstrating improved selectivity. Major efforts have focused on optimizing sensors based on DNA wrappings, which have intermediate properties that can be improved through synthetic modifications. Although SWCNT sensors have, to date, been mainly engineered using empirical approaches, herein we highlight alternative techniques based on iterative screening that offer a more guided approach to tuning sensor properties. These more rational techniques can yield new combinations that incorporate the advantages of the diverse nanotube wrappings available to create high performance optical sensors.
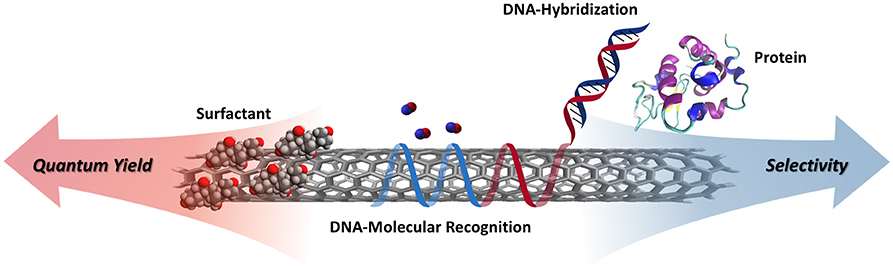
Graphical Abstract. Schematic illustrating the tradeoffs between quantum yield and selectivity for various non-covalent surface functionalizations.
1. Introduction
Optical sensors use light as a means of contactless detection for real-time sensing. Distinct optical signals from a single device enables multimodal detection of several analytes simultaneously, a feature that is especially advantageous for remote in vivo biosensing applications. Fluorescence-based optical sensors require two elements for operation: a molecular recognition element that selectively interacts with the analyte of interest and an optical transducer, such as a fluorophore, that converts this interaction into a measurable optical signal.
As described in several reviews (Boghossian et al., 2011; Liu et al., 2011; Kruss et al., 2013; Pan et al., 2017), single-walled carbon nanotubes (SWCNTs) are among the most promising fluorescence-based transducers for biosensing applications. They are one-dimensional nanostructures with optoelectronic properties that are tuned by tube diameter as a result of quantum confinement. Conceptualized as cylindrically rolled sheets of graphene, SWCNTs exist with various diameters, and they can be either metallic, semi-metallic, or semiconducting, depending on the direction the sheet is rolled. In 2002, O'Connell et al. demonstrated that semiconducting forms of SWCNTs dispersed in aqueous solutions emit photoluminescence at near-infrared (near-IR) wavelengths (O'Connell et al., 2002). This emission lies within the optical transparency window for biological material (Boghossian et al., 2011) which, when coupled with the nanotube's indefinite photostability and capabilities for single-molecule detection, makes SWCNTs attractive for in vivo continuous monitoring applications.
The use of SWCNTs as fluorescent transducers requires surface functionalization to impart optical stability and molecular recognition. Non-functionalized SWCNTs are inherently hydrophobic and exhibit a strong tendency to aggregate into bundles in aqueous solutions. Since most SWCNT preparations contain metallic nanotubes, these bundles contribute to the fluorescence quenching of semiconducting SWCNTs through the non-radiative exciton decay channels within the bundle (O'Connell et al., 2002; Maeda et al., 2004). Therefore, the bundles need to be exfoliated to generate individually suspended nanotubes in a liquid phase for most practical applications (Coleman, 2009). Specifically, this suspension allows the semi-conducting nanotubes to fluoresce in the near-IR. In addition to enabling solubilization and fluorescence, surface functionalization can also modify the nanotube surface to promote selective interactions with particular analytes of interest (Figure 1). The underlying mechanism for this selectivity depends on the wrapping and remains an ongoing area of research for many wrappings (Jeng et al., 2006; Hertel et al., 2010; Bisker et al., 2016; Polo and Kruss, 2016; Antonucci et al., 2017; Kruss et al., 2017; Mann et al., 2017; Zubkovs et al., 2017; Gillen et al., 2018; Wu et al., 2018; Lambert et al., 2019).
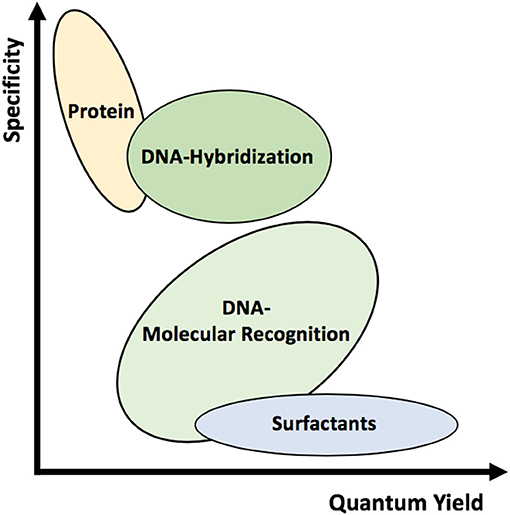
Figure 1. Schematic representation of the various wrappings used to suspend SWCNTs. Different wrappings can alter the quantum yield and specificity of the complexes.
Since covalent functionalization of the nanotube surface is known to strongly affect, or even diminish, the nanotube fluorescence, non-covalent modifications are typically used for creating optical sensors. The most common approach for non-covalently separating SWCNT bundles is liquid-phase exfoliation and stabilization (Coleman, 2009). This approach typically involves using forced dispersion (with sonication, for example) in the presence of wrappings, such as surfactants, synthetic polymers, oligonucleotides, and proteins that can stabilize the suspended SWCNTs (Figure 2). In addition to improving the solubility of the SWCNTs, these wrappings can also impart secondary characteristics, such as enhanced bio-compatibility and improved molecular sensitivity, overcoming problems associated with the chemical inertness of raw SWCNTs (Saifuddin et al., 2013).
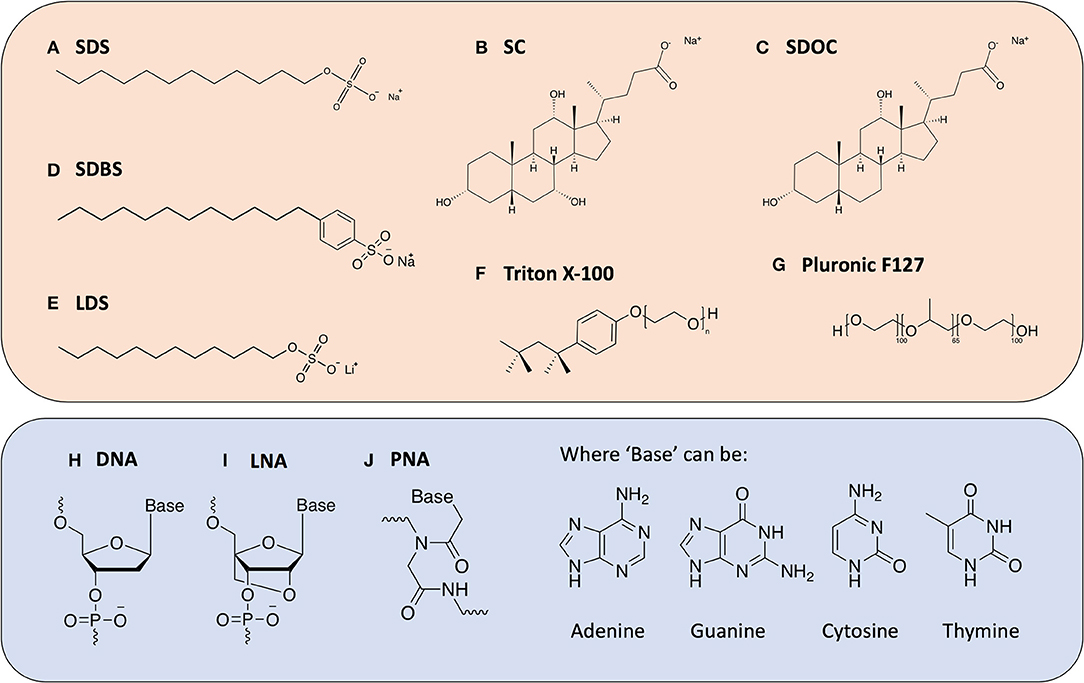
Figure 2. Chemical structures of the various dispersants discussed in this review. Surfactant molecules (top box) (A) Sodium Dodecylsulfate (SDS); (B) Sodium Cholate (SC); (C) Sodium Deoxycholate (SDOC); (D) Sodium Dodecylbenzenesulfonate (SDBS); (E) Lithium Dodecylsulfate (LDS); (F) Triton X-100; (G) Pluronic F127; and oligonucleotide-derived molecules (bottom box); (H) Deoxyribo Nucleic Acid (DNA); (I) Locked Nucleic Acid (LNA); (J) Peptide Nucleic Acid (PNA).
In this review, we present an overview of several key methods used for the non-covalent functionalization of SWCNTs. Beginning with surfactant-coated SWCNTs, we progress toward the use of biomolecules to suspend nanotubes, highlighting key advantages and disadvantages associated with each wrapping. Finally, we conclude with a consideration of new approaches aimed at overcoming some of the limitations of both surfactant- and biomolecule-suspended SWCNTs. These examples highlight emerging methods to selectively engineer improved SWCNT-based optical sensors in complex environments.
2. Surfactant-Coated SWCNTs
Surfactant-coated SWCNTs represent a standard comparative benchmark for nanotube suspensions, particularly with respect to achieving scalable wrapping procedures and the high quantum yields necessary for optical sensing. Historically, the first reported suspensions of individual SWCNTs were achieved using an aqueous surfactant, sodium dodecylsulfate (SDS) (O'Connell et al., 2001, 2002; Bachilo et al., 2002). The resulting isolation of the nanotubes from surrounding bundles greatly improved the optical resolution of the absorbance spectra. Additionally, the authors were able to characterize the direct band gap of individual semiconducting SWCNTs with fluorescence spectroscopy (Bachilo et al., 2002; O'Connell et al., 2002), which was first hypothesized in the early 1990s (Dresselhaus et al., 1992; Hamada et al., 1992; Saito et al., 1992) and previously detected using Raman and STEM (Wildoer et al., 1998; Kataura et al., 1999).
To prevent re-bundling and obtain a thermodynamically stable suspension, the strong cohesion energy of the isolated tubes (~120 kT nm−1) must be compensated by either tube-solvent, or in the case of surfactant-suspended SWCNTs, tube-surfactant interactions (Angelikopoulos and Bock, 2012). However, SWCNT suspensions often exist in a kinetically meta-stable state. Kinetic stabilization does not fully overcome the cohesion energy of the tubes; instead the surfactant creates a repulsive force between the tubes that reduces the likelihood of forming tube-tube contacts, hence slowing aggregation (Angelikopoulos and Bock, 2012). Similar to the interactions in the kinetic stabilization of colloids (Tummala and Striolo, 2008, 2009; Angelikopoulos and Bock, 2012; Kato et al., 2012; Oh et al., 2013). Previous studies hypothesized that the individual nanotubes are encased in the hydrophobic interiors of the micelle. The hydrophilic part of the surfactant molecules is believed to face outwards, creating a cylindrical micelle and a repulsive force between the nanotubes that renders a thermodynamically meta-stable suspension (Figure 3) (Angelikopoulos and Bock, 2008, 2012).
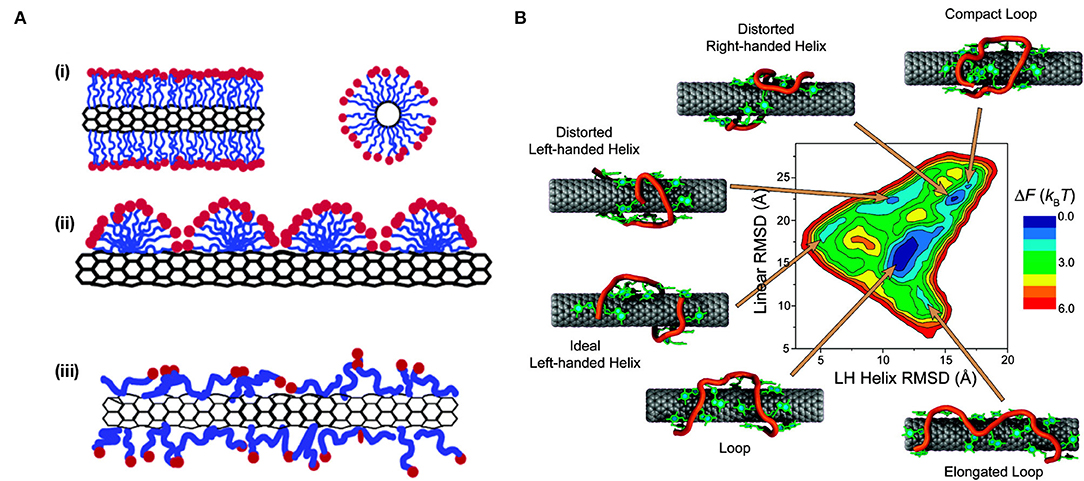
Figure 3. Conformations of non-selective wrappings on SWCNT surface. (A) A schematic representation of the various mechanisms by which surfactant molecules interact and disperse SWCNTs. (i) Cylindrical micelle encapsulation; (ii) Hemimicellar adsorption; and (iii) Random adsorption. Reprinted with permission from Yurekli et al. (2004). Copyright © 2004, American Chemical Society. (B) Free energy landscape of (GT)7-SWCNT hybrid at room temperature. Representative conformations for each local minimum are displayed. The sugar-phosphate backbone is depicted in orange, and bases are shown in green. Reprinted with permission from Johnson et al. (2009). Copyright © 2009, American Chemical Society.
The use of surfactants to suspend SWCNTs has since expanded to include other anionic, cationic, and non-ionic surfactants (Hirsch, 2002; Wenseleers et al., 2004; Crochet et al., 2007; Haggenmueller et al., 2008; Blanch et al., 2010; Bergler et al., 2016; Nonoguchi et al., 2018), such as sodium cholate (SC), sodium deoxycholate (SDOC), sodium dodecylbenzenesulfonate (SDBS), lithium dodecyl sulfate (LDS), Triton X-100, and pluronic F127. Depending on the surfactant, high-quality dispersions can be achieved with large populations of individualized nanotubes (Coleman, 2009) and SWCNT concentrations >1 mg/mL. However, different surfactants have been found to vary greatly in the degree of dispersion and stability of the resulting suspensions. This variation is, in part, attributed to the interactions between the surfactant and nanotube, which result in the formation of different structures with varying degrees of surface coverage (Matarredona et al., 2003). In addition to cylindrical micelle SWCNT encapsulation, as was proposed for the SDS-suspended SWCNTs, two additional configurations include (Angelikopoulos and Bock, 2012; Xin et al., 2013) (i) Langmuir-type (random molecular adsorption) layers and (ii) adsorbed spherical and hemispherical micelles (Islam et al., 2003; Vo et al., 2016; Vo and Papavassiliou, 2017) (Figure 3A). The latter, spherical and hemispherical micelle formation, is adopted only by strong amphiphiles that prefer higher curvature aggregates. This formation of hemimicellar aggregates on the surface of the SWCNTs typically involves adsorption of the surfactant onto the nanotube followed by the self-assembly of the molecules, which is enabled by diffusion along the nanotube surface (Vo et al., 2016). In contrast, the former, random adsorption of the surfactant on the SWCNT surface, is adopted by weakly amphiphilic molecules [such as flavin mononucleotides (FMN)] and bile acid surfactants (including SC and SDOC) where adsorption is competitive, i.e., follows a Langmuir isotherm (Angelikopoulos and Bock, 2010, 2012; Tummala et al., 2010; Bergler et al., 2016; Xu et al., 2017).
According to both experiment and simulation, the degree of exposed SWCNT surface coverage following adsorption of surfactant molecules under all three regimes is largely dependent on surfactant concentration (Matarredona et al., 2003). Indeed, Wang et al. (2004) have shown that for Triton-X, the optimal surfactant dispersion concentration is different from the critical micelle concentration (CMC) and results from competition between maximizing surfactant adsorption on the nanotube surface and micelle-mediated depletion interactions between adjacent SWCNT bundles. Moreover, previous reports have shown vast differences in the maximum relative solubility of SWCNT complexes using the same surfactant, with an apparent dependence on the processing method and conditions. For example, suspensions made with SDBS can have maximum SWCNT concentrations of 20 mg/mL (Islam et al., 2003) or no more than ~0.01 mg/mL (Moore et al., 2003), depending on the dispersion approach.
Another factor believed to impact the stability of surfactant-dispersed SWCNTs is the ζ-potential. When surfactant molecules adsorb onto the surface of SWCNTs, the surfactant counter-ion (commonly Na+ or Li+) is dissociated from the hydrophilic head group of the surfactant. These counter-ions are spatially separated from the tail group of the molecular ions, arranging in a diffuse cloud that acts as a multi-pole. As a result, surfactant-suspended nanotubes appear, from a distance, to carry an effective charge associated with this double layer, which is denoted as the ζ-potential (Coleman, 2009). This potential is equivalent to the electrostatic potential measured at the edge of the layer at the bound surfactant tail groups, and it acts as a repulsive interaction potential between neighboring SWCNTs. In a study by Sun et al. (2008), the dispersion quality of six surfactant molecules was tested. Each of the dispersion-quality metrics were found to scale well with the measured ζ-potential of the dispersion, with SDS suspending better than both SDBS and SC, corresponding to ζ-potential values of −70.0, −68.8, and −48.8 mV, respectively. These findings indicate that the dispersion quality of surfactant-SWCNTs may be controlled by the magnitude of the electrostatic repulsive forces between the coated SWCNTs (White et al., 2007; Sun et al., 2008), a property that can be tuned in order to improve the long-term stability of these solutions.
Given the dependence of maximum dispersion concentration and stability on surfactant type, we focus the remainder of our discussion on the four most commonly used surfactants for SWCNT suspension, SC, SDOC, SDS, and SDBS. These surfactants have been shown to achieve stable dispersions with suspension efficiencies above 40% (Haggenmueller et al., 2008). Despite the similar structures of SC and SDOC, which only differ by a hydroxy group, SDOC shows a marked increase in suspension yield (+17%). In addition to dispersion efficiency, the resolution of the optical absorption spectrum can be used to determine differences in the quality of SWCNT suspensions. Distinct absorption peaks are observed for both SC and SDOC, while SDS and SDBS show much broader peaks. In instances where SWCNTs are not effectively exfoliated, the van der Waals interactions between aggregated nanotubes result in broad, weak absorption peaks (Antonucci et al., 2017). This observation therefore suggests that SC and SDOC can generally yield more monodisperse SWCNTs under the studied preparation conditions. On the other hand, the broader peaks observed for SDS and SDBS indicate that these surfactants do not effectively de-bundle the nanotubes, resulting in a poorer dispersion quality despite the apparently high suspension yields.
In addition to their high dispersion efficiencies, these surfactants also benefit from a number of additional advantages. Compared to most biopolymers, these wrappings yield SWCNT suspensions that are relatively cheap and stable, and the preparation procedures are scalable enough to produce large volumes of monodisperse SWCNTs, which is an important consideration for the industrialization of nanotube sensors. Furthermore, surfactant-suspended SWCNTs typically exhibit much larger suspension (Coleman, 2009) and quantum yield values (Haggenmueller et al., 2008) compared to both protein- and DNA-suspended SWCNTs. The increased fluorescence intensity is attributed, in part, to the increased surface coverage of the surfactant on the SWCNT surface. The increased coverage results in higher levels of oxygen and water shielding, which has been shown to decrease nanotube fluorescence (Zheng et al., 2017), thereby leading to brighter SWCNT complexes. This increase in brightness is particularly important for biosensing applications, where penetration depth and sensor sensitivity have been linked to quantum yield (Yum et al., 2013; Bonis-O'Donnell et al., 2017, 2019; Beyene et al., 2018).
Toxicity is an additional metric when considering surfactant-suspended SWCNTs for in vitro and in vivo biosensing applications. Surfactants allow SWCNTs to disperse in water, the universal and biological solvent, permitting researchers to flexibly carry out a variety of environmental, biocompatibility, and safety analyses (Coleman, 2009). However, certain surfactants, such as SDS and SDBS, are known to be cytotoxic to cells even at concentrations as low as 0.05 mg ml−1 (Dong et al., 2008), and similar effects have been observed for nanotubes suspended with these surfactants (Dong et al., 2008, 2009). In studies performed by Dong et al. (2008) and Dong et al. (2009), neither the proliferation nor viability of the cells was affected by pristine SWCNTs in the absence of surfactant. Furthermore, conjugates of SWCNTs suspended with various concentrations of SC also showed no negative impact on cell viability and growth, and proliferation was comparable to that of untreated cells. The observed cytotoxicity of the nanotube-surfactant conjugates was therefore believed to be driven by the inherent cytotoxicity of the surfactant in the suspension (Dong et al., 2008, 2009). These studies illustrate the importance surfactant selection in overcoming challenges in toxicity. Although issues such as toxicity can be mitigated through appropriate selection of surfactant type and concentration, surfactant-suspended SWCNTs are limited for biosensing applications due to their lack of inherent selectivity. As a result, current efforts focus on the use of alternative wrappings to suspend SWCNTs, including biopolymers, such as single-stranded DNA (ssDNA) and proteins.
3. Biopolymer-Suspended SWCNTs
DNA is one of the most extensively studied wrappings for optical sensing applications based on Raman, fluorescence, and absorption spectroscopies (Zheng et al., 2003a; Heller et al., 2006; Enyashin et al., 2007; Zhang et al., 2011; Bansal et al., 2013; Kupis-Rozmysłowicz et al., 2016; Wu et al., 2018). The non-covalent functionalization of ssDNA is based on π-stacking of the aromatic nucleotide bases with the sp2-hybridized side-wall of carbon nanotubes (Zheng et al., 2003a). This arrangement exposes the negatively charged sugar-phosphate backbone of the DNA, which is hydrophilic and easily solvated, toward the water, enabling suspension of the DNA-SWCNT complexes in aqueous solutions (Zheng et al., 2003a). These favorable side-wall-DNA and DNA-water interactions yield suspensions that are facile and stable at room temperature for several months (Zheng et al., 2003a). Work carried out by Zheng et al. (2003a) showed that almost any ssDNA sequence could be used to successfully suspend SWCNTs in the presence of a denaturant and mild sonication. Although atomic force microscopy (AFM) measurements and simulations show DNA to helically self-assemble around the SWCNT (Zheng et al., 2003a,b), the final binding structure has been shown to be sequence-dependent, and short ssDNA strands may also assume other configurations on the nanotube surface (Zheng et al., 2003a; Johnson et al., 2008, 2009) (Figure 3B). The sparser surface coverage of the DNA compared to surfactants such as SC exposes a larger carbon surface to water, resulting in a decrease in the intensity and emission energy of the SWCNT fluorescence. For example, the (7,5) chirality undergoes a bathochromic shift of 17.6 meV (15.6 nm) when wrapped in ssDNA instead of SC due to the greater water accessibility of the DNA wrapping and the resulting increase in the local dielectric constant at the nanotube surface (Jeng et al., 2006; Li and Shi, 2014). Such changes in the local dielectric have been shown to yield an expected fluorescence shift in SWCNT emission peaks (Choi and Strano, 2007).
In addition to the facile suspension procedure and stable assembly, ssDNA benefits from additional features ideal for scale-up sensor design. DNA-wrapped SWCNT suspensions can be further concentrated to achieve dispersion yields as high as 4 mg ml−1 (Zheng et al., 2003a). Additionally, the nearly limitless variability in sequence length and composition, as well as the well-established chemistries available for DNA functionalization, make ssDNA an incredibly malleable polymer for tuning the characteristics of the suspended SWCNTs. For example, Zheng et al. modified DNA-SWCNTs at one end with biotin that was used for immobilization onto streptavidin-coated beads (Zheng et al., 2003a). This study demonstrates one of many biochemical approaches for controlling DNA-SWCNT behavior by specifically engineering DNA-SWCNT complexes. Furthermore, both sequence length and base composition has been shown to impact the interaction potential of ssDNA with the surface of SWCNTs (Zheng et al., 2003a; Safaee et al., 2019), which has also recently been shown to vary with SWCNT chirality (Jena et al., 2017; Safaee et al., 2019).
The ability of DNA to form chirality-specific interactions has been exploited for a variety of applications, most notably, chirality separation. Chirality separation is key for multi-modal sensing applications where each chirality selectively responds to a distinct analyte in a solution mixture. Following separation, the individual chiralities can each be functionalized with a wrapping that selectively responds to a particular analyte of interest, and the analyte is detected by monitoring the corresponding wavelength. Many separation mechanisms have been devised (Chattopadhyay et al., 2003; Krupke et al., 2003, 2004; Zheng et al., 2003a,b; Heller et al., 2004; Strano et al., 2004; Huang et al., 2005; Lustig et al., 2005; Arnold et al., 2006; Peng et al., 2006; Zheng and Semke, 2007; Tu and Zheng, 2008; Tu et al., 2009; Zhang et al., 2015) with varying degrees of success; however, a facile approach for scalable, complete and total fractionation into all the single chiralities remains elusive. Aqueous two-phase polymer (ATP) separation (Khripin et al., 2013; Ao et al., 2014, 2016; Ao and Zheng, 2015; Subbaiyan et al., 2015) has emerged at the forefront of methods currently employed in chirality separation. Briefly, an ATP system consists of two separate, but permeable, water phases of slightly different compositions that is created by polymer phase separation (Ao et al., 2016). Studies have shown that the partitioning of DNA-SWCNT complexes has a strong dependence on both the DNA sequence and SWCNT structure (i.e., chirality) (Ao et al., 2014). Moreover, this partitioning can be modulated by changing the polymer compositions of the two phases in order to rescale the hydration energies. For example, the addition of dextran (DX) to a poly-(ethylene glycol)/polyacrylamide (PEG/PAM) system pulls down DNA-SWCNTs from the top to the bottom phase (due to increased hydrophilicity) while the addition of poly(vinylpyrrolidone) (PVP) has the opposite effect. The effectiveness of this method was demonstrated in work carried out by Ao et al. (Khripin et al., 2013; Ao et al., 2014, 2016; Ao and Zheng, 2015), where over 300 DNA sequences were screened using ATP separation techniques, resulting in the isolation of 23 different chiralities.
Aside from their chirality specificity and selectivity, different DNA lengths and sequences have also shown preferences toward molecular recognition with certain analytes (Figure 4). Small nucleotide and microRNA sequences are promising biomarkers for a variety of pathologies, including cancer (Harvey et al., 2017). However, current methods of detection are complex and time-consuming, leading to difficulties in their implementation for point-of-care diagnostics. An advantage of DNA-SWCNT optical sensors is the ability to engineer selectivity toward target oligonucleotides by taking advantage of DNA's natural preference for specific complementary base pairing. Many studies have demonstrated the use of DNA-SWCNTs to quantitatively detect a range of both microRNA and DNA sequences (Jeng et al., 2006, 2010; Bansal et al., 2013; Harvey et al., 2017). Work carried out by Jeng et al. and Harvey et al. have shown that these fluorescence-based sensors are even capable of detecting single nucleotide polymorphisms (SNPs) (Jeng et al., 2010) and can be multiplexed to detect several sequences simultaneously (Harvey et al., 2017). In the study by Jeng et al., the addition of complementary DNA is believed to increase the surface coverage of the SWCNT upon hybridization, resulting in a decrease in the effective dielectric constant of the surrounding SWCNT environment and a shifting of the SWCNT fluorescence peak. Similarly, Harvey et al. propose an underlying mechanism based on changes in dielectric constant and electrostatic charge, which can modulate SWCNT emission wavelengths upon hybridization. The fluorescence shifting observed in both studies in response to complementary hybridization is particularly advantageous for detecting diseases, such as heart and kidney disease, as well as various cancers, which can be associated with different combinations of specific microRNA sequences (Etheridge et al., 2011; Hayes et al., 2014; Mishra, 2014; Bertoli et al., 2015; Wang et al., 2016; Hamam et al., 2017).
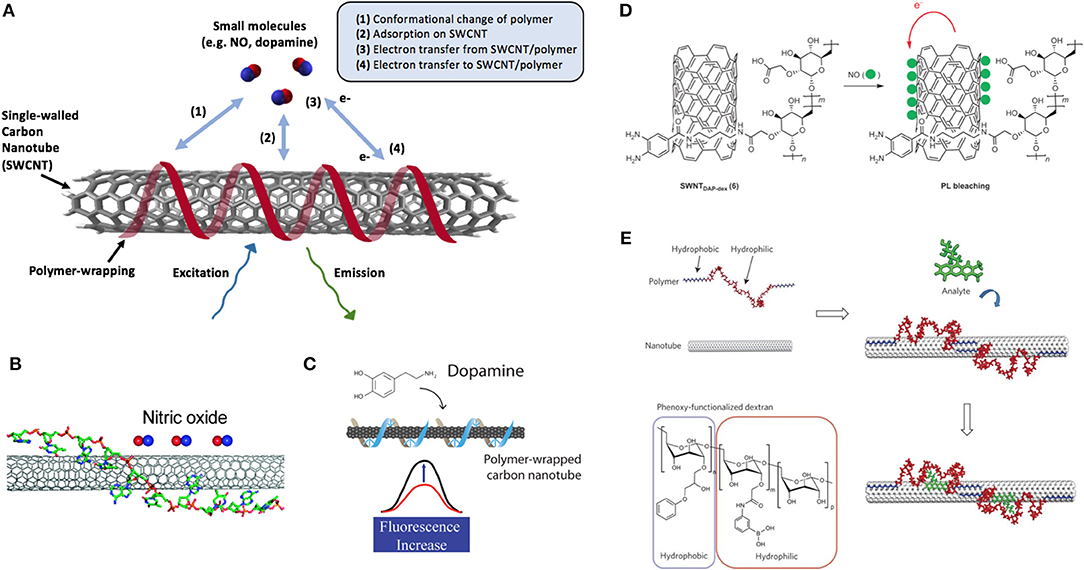
Figure 4. Non-specific interactions of polymer wrappings on SWCNT surface. (A) Common mechanisms of interaction between small molecules and polymer-wrapped SWCNTs. (B) The optical response of (AT)15 DNA oligonucleotide-wrapped SWCNT [(AT)15-SWCNT] upon exposure to NO. A side schematic view of one of the several binding structures of (AT)15-SCWNT simulated from a HyperChem simulation package. Bases of the DNA stack on the sidewall of the SWCNT, and the sugar-phosphate backbone extends away from the surface. Adapted with permission from Zhang et al. (2011). Copyright © 2011, American Chemical Society. (C) Schematic of the fluorescent turn-on sensor for dopamine. Adapted with permission from Kruss et al. (2014). Copyright © 2006, American Chemical Society. (D) Schematic illustration for NO detection using SWCNT-polymer hybrids, highlighting the mechanism for near-IR fluorescence bleaching by NO with SWCNT-DAP-dextran complexes. PL = photoluminescence. Reprinted with permission from Kim et al. (2009). Copyright © 2009, Nature Publishing Group. (E) A synthetic polymer with an alternating hydrophobic-and-hydrophilic sequence adopting a specific conformation when adsorbed to the nanotube. The polymer is pinned in place to create a favored recognition site for the molecules of interest, leading to either a wavelength or intensity change in SWCNT fluorescence. Adapted with permission from Zhang et al. (2013). Copyright © 2013, Nature Publishing Group.
In addition to detecting hybridization, DNA-SWCNTs can also be engineered to detect a variety of other molecules, including neurotransmitters, sugars, and peptides (Xu et al., 2007; Kruss et al., 2017; Landry et al., 2017; Bisker et al., 2018), though the underlying mechanism of these sensors remains an ongoing area of research (Bisker et al., 2015; Ulissi et al., 2015). While certain DNA-SWCNT sensors are based on oligonucleotides that act as molecular sieves, like the (AT)15-SWCNT sensors designed to detect NO (Zhang et al., 2011), an alternative approach is based on displacement or conformational changes of the DNA wrapping (Heller et al., 2006; Landry et al., 2014, 2017; Salem et al., 2017; Beyene et al., 2018; Gillen et al., 2018). Early studies in this area screened libraries of molecules of interest against SWCNTs suspended using several different DNA sequences by monitoring the changes in the fluorescence emission of these sensors upon addition of the analyte. Through this approach, researchers were able to identify particular sequences with an enhanced affinity to certain chemicals, such as (AT)15 toward nitric oxide (NO) (Zhang et al., 2011) (Figure 4B) and (GT)15 toward catecholamines (Zhang et al., 2011; Kruss et al., 2017; Mann et al., 2017) (Figure 4C). Further studies have demonstrated that DNA length can also be used to tune the fluorescence properties of DNA-SWCNT hybrids, offering a new approach to controlling the behavior of these sensors (Jena et al., 2017; Beyene et al., 2018).
Recent studies carried out by Landry et al. and Lee et al. have shown that DNA aptamers on SWCNT scaffolds can be used to detect certain biologically relevant proteins (Landry et al., 2017; Lee et al., 2018). This label-free fluorescence detection offers many advantages over conventional immunological analytical methods, such as enzyme-linked immunosorbent assays (ELISA) or mass spectroscopy, which lack temporal resolution for real-time quantification of protein levels. Furthermore, this method obviates cumbersome purification and labeling steps typically required by more classical approaches. Both RAP1 and HIV-1 (Landry et al., 2017) and platelet-derived growth factor (PDGF) (Lee et al., 2018) were successfully detected using DNA aptamer-SWCNT complexes. Moreover, the RAP1 and HIV-1 sensors were also reported to selectively respond to their target proteins in molecularly complex environments, such as crude, unpurified cell lysates (Landry et al., 2017).
Although DNA-SWCNTs have shown improved selectivity toward small molecules compared to surfactant-SWCNTs (Zhang et al., 2013), they still lag behind their protein- and peptide-based counterparts, which offer exceptional molecular recognition. Proteins are capable of not only differentiating between molecularly similar targets, but also different chiralities of the same molecule. For example, whereas proteins, such as glucose oxidase (GOX) selectively interact with D-glucose (Zubkovs et al., 2017), sensors based on the (GT)15 DNA wrapping interact with a family of catecholamines (Kruss et al., 2014, 2017; Mann et al., 2017). Although glucose sensors based on DNA-SWCNTs have also been developed, these sensors ultimately require the addition of GOX for specificity due to the structural similarities of competing sugar molecules. Furthermore, the underlying sensing mechanisms for protein-based sensors are often more clearly identifiable. Their sensing mechanisms are quite diverse, varying from protein charge-transfer (Barone and Strano, 2006; Zubkovs et al., 2017) to exciton quenching due to protein conformational changes (Yoon et al., 2011), both of which have been shown to alter the SWCNT fluorescence intensity.
Protein-based wrappings, however, suffer from their own disadvantages; a lack of precise control during the protein immobilization process, for example, can result in unfavorable orientations that limit access to the active site (Mohamad et al., 2015). Similarly, structural rearrangements may occur that inhibit, or in some cases destroy, the bioactivity of these molecules (Saifuddin et al., 2013; Antonucci et al., 2017). In addition, protein-based wrappings exhibit limited dispersion efficiency, which has been shown to depend on the protein and is generally less efficient than DNA- and surfactant-based suspensions. Several methods of functionalization have been proposed (Huang et al., 2002; Jiang et al., 2004; Gao and Kyratzis, 2008; Saifuddin et al., 2013; Antonucci et al., 2017) and used to create sensors based on Luciferase-suspended SWCNTs (Kim et al., 2010), AnnexinV-suspended SWCNTs (Neves et al., 2013), and anti-uPA-suspended SWCNTs (Williams et al., 2018), for example. However, these sensors require an intermediate linker or wrapping for stability, as opposed to the non-specific adsorption possible with GOX. In fact, other protein-based glucose sensors, such as those based on glucose-binding protein (Yoon et al., 2011; Yum et al., 2013), typically require more complex conjugative chemistries compared to GOX, highlighting the importance of understanding the underlying protein mechanism when determining the most appropriate method for functionalization. Although these intermediate wrappings can improve solubilization and help maintain protein structure and function on the SWCNT surface, more complex functionalization procedures with multiple conjugation steps could limit the scalability of the sensors.
Irrespective of the improved selectivity offered by DNA and especially protein-suspended SWCNTs, these sensors suffer from relatively low quantum yields compared to surfactant-suspended nanotubes (Haggenmueller et al., 2008). The low intensities restrict the depth at which these biosensors can be implanted for use in vivo and in vitro. Moreover, studies have shown that both protein- and DNA-SWCNTs are sensitive to local variations in pH (Nepal and Geckeler, 2006; Antonucci et al., 2017) and ionic strength (Heller et al., 2006; Holt et al., 2012; Salem et al., 2017; Gillen et al., 2018). The latter poses additional challenges for biosensing applications, as these ions are often involved in biological signaling pathways (such as Ca2+ in dopamine regulation). Therefore, fluctuations in local concentrations throughout the day would compromise the sensing capabilities of the DNA-SWCNT complexes.
4. Polymer Engineering of SWCNT Sensor Specificity
The tradeoffs between surfactant-suspended SWCNTs and biopolymer-suspended SWCNTs have encouraged researchers to seek an alternative means of detection based on synthetic or bioengineered polymers. Xeno nucleic acids (XNAs), for example, have recently been engineered to improve the sensing capabilities of DNA-SWCNTs in ionically complex systems (Gillen et al., 2018). XNAs are synthetic alternatives to naturally occurring DNA and RNA that typically benefit from greater resistivity against nuclease degradation. Due to their modularity, nucleic acids can be readily adjusted using a variety of chemical modifications (Pinheiro and Holliger, 2012; Pinheiro et al., 2013; Ghosh and Chakrabarti, 2016; Ma et al., 2016), and XNAs can contain modifications to either the nucleobase, phosphate, or sugar in an otherwise native oligonucleotide sequence (Pinheiro et al., 2013; Pinheiro and Holliger, 2014; Anosova et al., 2016). Although XNAs were initially developed to emulate the DNA replication processes found in nature, these synthetic oligomers were quickly realized for their advantages in in vivo stability and specificity (Wang et al., 2005; Pinheiro and Holliger, 2014; Taylor et al., 2014; Ma et al., 2016). Larger base modifications can result in altered physico-chemical properties, such as a tendency to adopt non-standard helical conformations, but certain chemical modifications to the N7 (in purines) or C5 (in pyrimidines), sites that extend into the major DNA groove, can be reasonably tolerated without significant steric impact (Pinheiro and Holliger, 2012). Backbone modifications can also alter the physico-chemical properties of oligonucleotides. One example is peptide nucleic acid (PNA), where the sugar phosphate backbone is substituted with aminoethylgylcine. This substitution results in a charge-neutral polymer that is capable of strong canonical base pairing. The type and extent of the modification depends on the intended application. For example, locked nucleic acid (LNA) can greatly improve the stability of SWCNT sensors in the presence of high ionic concentrations (Gillen et al., 2018). Previous studies showed that salt cations can alter the DNA conformation on the nanotube surface, changing the emission wavelengths (Heller et al., 2006; Salem et al., 2017; Gillen et al., 2018). Since the added methyl bridge in the backbone of LNA increases the rigidity of the polymer, LNA exhibits increased conformation stability in the presence of fluctuating salt concentrations. By modifying 25% of the sequence with a “locked” base, bioengineered sensors based on LNA have been shown to withstand over two orders of magnitude higher salt concentrations without any perturbations in fluorescence. These complexes offer a strong promise for use in ionically complex media, such as blood or urine, without compromising the biocompatibility or nearly inexhaustible sequence space offered by oligonucleotide wrappings. The added chemical modifications also carry untapped potential for further narrowing selectivity through bio-conjugative chemistries that are specific to functional groups in the desired target.
Similarly, recent work by Chio et al. has employed the use of peptoids, N-substituted glycine polymers, to serve as protein molecular recognition elements for SWCNT-based sensors (Chio et al., 2019). These peptoids draw inspiration from biological peptides, with the benefit of greater resistivity against protease degradation (Anosova et al., 2016). The tunability of these sequence-defined synthetic polymers enables greater chemical diversity by providing a larger monomer space of primary amines (Sun and Zuckermann, 2013). Although the stability of the peptoid wrapping on the nanotube surface was shown to vary depending on composition, length, charge and polarity, Chio et al. demonstrated that these sensors could be used to engineer a selective sensor for the fluorescence detection of the lectin protein, wheat germ agglutinin (WGA) (Chio et al., 2019).
In addition to peptoids and oligonucleotide derivatives, purely synthetic heteropolymers have also been used to augment sensor properties. One such platform uses Corona Phase Molecular Recognition (CoPhMoRe) and relies on SWCNT-adsorbed heteropolymers to template preferential recognition sites for target analytes. The final structures adopted by the polymer on the surface control the selectivity of the sensor toward a target. Though the mechanism for modulating SWCNT fluorescence in response to binding is likely analyte- and polymer-specific, its precise characterization remains an area of active research (Bisker et al., 2015; Ulissi et al., 2015). Typically, the heteropolymers employed contain both hydrophobic and hydrophilic segments. The former interacts with the SWCNT surface, while the latter extends into solution to suspend the complex in aqueous solutions. CoPhMoRe-based sensors have been developed to detect neurotransmitters (Kruss et al., 2013; Zhang et al., 2013; Landry et al., 2014), vitamins (Zhang et al., 2013), and steroids (Zhang et al., 2013), as well as small molecules, such as NO and H2O2 (Kim et al., 2009; Iverson et al., 2013; Giraldo et al., 2015) (Figure 4).
Furthering the development of these sensors, Bisker et al. (2016) extended the capabilities of CoPhMoRe sensors to detect larger macromolecules, such as proteins. A variant of a CoPhMoRe screening approach was used to identify polymeric wrappings that could be used to create synthetic, non-biological antibody analogs capable of recognizing biological macromolecules. This approach yielded a selective sensor for fibrinogen based on dipalmitoyl-phosphatidylethanolamine (DPPE)-PEG (5 kDa)-suspended SWCNTs. This sensor was capable of detecting fibrinogen in a competitive binding assay in the presence of albumin, which can passivate the sensor by binding to non-specific binding sites (Bisker et al., 2016). This observation suggests that CoPhMoRe is more likely due to a combination of factors related to both the specific corona phase formed by the polymer-SWCNT complex and the unique elongated conformation of the fibrinogen protein, rather than sensing mechanisms based on aggregation, molecular weight, or protein hydrophobicity.
5. Conclusions and Future Perspective
Since the first reported aqueous suspension of individual SWCNTs with surfactant (O'Connell et al., 2001, 2002; Bachilo et al., 2002), SWCNTs have been suspended using a variety of natural and synthetic wrappings. Polymer wrappings in particular have served the dual purpose of both solubilizing SWCNTs and regulating the selectivity of SWCNTs toward specific analytes in biological media. As a result, polymers such as DNA have become standard wrappings for optical SWCNT-based biosensing, and recent efforts have focused on modifying these polymers to improve the quantum yield, stability, scalability, and selectivity of these sensors. However, with the exception of protein-based wrappings and complementary DNA-strand hybridization, the nature of the selectivity of polymer wrappings toward specific analytes remains unclear. As a result, most DNA and synthetic polymer-based SWCNT sensors are empirically engineered through random library screening and selection. These techniques evaluate the responsivity of several different polymer-wrapped SWCNTs against a variety of analytes, and the polymer-analyte combinations that yield relatively strong fluorescence responses are used to identify suitable polymer wrappings for SWCNT-based sensing (Zhang et al., 2011, 2013). Though this approach has been quite successful in identifying wrappings that can trigger a fluorescence response toward particular analytes, the sensors often show compromised selectivity. Moreover, the polymer wrappings also yield sensors with lower stability and brightness compared to surfactant wrappings.
Studies for new SWCNT optical sensors thus far screen, at most, tens of polymers at a given time (Zhang et al., 2011, 2013), meaning they have only explored a small fraction of the near-infinite polymer sequence space. One approach to overcoming the current limitations of SWCNT sensors is to screen larger polymer libraries in order to increase the chances of identifying a polymer-analyte combination with more favorable sensing properties. An alternative approach to addressing this challenge is to implement more guided techniques, such as directed evolution (Arnold, 1997). Directed evolution uses an iterative approach to improving the properties of materials that lack a defined structure-function relationship. Though the technique is conventionally used to engineer proteins, it was recently applied to engineer DNA wrappings that were shown to improve the quantum yield of an optical SWCNT-based sensor (Lambert et al., 2019). Combined with computational methods, such guided approaches can be used to identify trends between polymer sequence and sensor properties, with the goal of ultimately understanding the underlying mechanism for selectivity and designing molecular probes in a rational and predictive manner.
Author Contributions
AG and AB contributed to the research and writing of this manuscript. Both authors have agreed on the final version of the manuscript submitted.
Conflict of Interest Statement
The authors declare that the research was conducted in the absence of any commercial or financial relationships that could be construed as a potential conflict of interest.
References
Angelikopoulos, P., and Bock, H. (2008). Directed self-assembly of surfactants in carbon nanotube materials. J. Phys. Chem. B 112, 13793–13801. doi: 10.1021/jp804891a
Angelikopoulos, P., and Bock, H. (2010). The differences in surfactant adsorption on carbon nanotubes and their bundles. Langmuir 26, 899–907. doi: 10.1021/la902376b
Angelikopoulos, P., and Bock, H. (2012). The science of dispersing carbon nanotubes with surfactants. Phys. Chem. Chem. Phys. 14, 9546–9557. doi: 10.1039/c2cp23436j
Anosova, I., Kowal, E. A., Dunn, M. R., Chaput, J. C., Horn, W. D., and Egli, M. (2016). The structural diversity of artificial genetic polymers. Nucleic Acids Res. 44, 1007–1021. doi: 10.1093/nar/gkv1472
Antonucci, A., Kupis-Rozmysłowicz, J., and Boghossian, A. A. (2017). Noncovalent protein and peptide functionalization of single-walled carbon nanotubes for biodelivery and optical sensing applications. ACS Appl. Mater. Interfaces 9, 11321–11331. doi: 10.1021/acsami.7b00810
Ao, G., Khripin, C. Y., and Zheng, M. (2014). DNA-controlled partition of carbon nanotubes in polymer aqueous two-phase systems. J. Am. Chem. Soc. 136, 10383–10392. doi: 10.1021/ja504078b
Ao, G., Streit, J. K., Fagan, J. A., and Zheng, M. (2016). Differentiating left- and right-handed carbon nanotubes by DNA. J. Am. Chem. Soc. 138, 16677–16685. doi: 10.1021/jacs.6b09135
Ao, G., and Zheng, M. (2015). Current Protocols in Chemical Biology, Vol. 7. Hoboken, NJ: John Wiley & Sons, Inc., 43–51.
Arnold, M. S., Green, A. A., Hulvat, J. F., Stupp, S. I., and Hersam, M. C. (2006). Sorting carbon nanotubes by electronic structure using density differentiation. Nat. Nanotechnol. 1, 60–65. doi: 10.1038/nnano.2006.52
Bachilo, S. M., Strano, M. S., Kittrell, C., Hauge, R. H., Smalley, R. E., and Weisman, R. B. (2002). Structure-assigned optical spectra of single-walled carbon nanotubes. Science 298, 2361–2366. doi: 10.1126/science.1078727
Bansal, J., Singh, I., Bhatnagar, P. K., and Mathur, P. C. (2013). DNA sequence detection based on raman spectroscopy using single walled carbon nanotube. J. Biosci. Bioeng. 115, 438–441. doi: 10.1016/j.jbiosc.2012.11.002
Barone, P. W., and Strano, M. S. (2006). Reversible control of carbon nanotube aggregation for a glucose affinity sensor. Angew. Chem. Int. Ed. 45, 8138–8141. doi: 10.1002/anie.200603138
Bergler, F. F., Stahl, S., Goy, A., Schöppler, F., and Hertel, T. (2016). Substrate-mediated cooperative adsorption of sodium cholate on (6,5) single-wall carbon nanotubes. Langmuir 32, 9598–9603. doi: 10.1021/acs.langmuir.6b02759
Bertoli, G., Cava, C., and Castiglioni, I. (2015). Micrornas: new biomarkers for diagnosis, prognosis, therapy prediction and therapeutic tools for breast cancer. Theranostics 5, 1122–1143. doi: 10.7150/thno.11543
Beyene, A. G., Alizadehmojarad, A. A., Dorlhiac, G., Streets, A. M., Král, P., Vuković, L., et al. (2018). Ultralarge modulation of single wall carbon nanotube fluorescence mediated by neuromodulators adsorbed on arrays of oligonucleotide rings. Nano Lett. 18, 6995–7003. doi: 10.1021/acs.nanolett.8b02937
Bisker, G., Ahn, J., Kruss, S., Ulissi, Z. W., Salem, D. P., and Strano, M. S. (2015). A mathematical formulation and solution of the CoPhMoRe inverse problem for helically wrapping polymer corona phases on cylindrical substrates. J. Phys. Chem. C 119:24. doi: 10.1021/acs.jpcc.5b01705
Bisker, G., Bakh, N. A., Lee, M. A., Ahn, J., Park, M., O'Connell, E. B., et al. (2018). Insulin detection using a corona phase molecular recognition site on single-walled carbon nanotubes. ACS Sens. 3, 367–377. doi: 10.1021/acssensors.7b00788
Bisker, G., Dong, J., Park, H. D., Iverson, N. M., Ahn, J., Nelson, J. T., et al. (2016). Protein-targeted corona phase molecular recognition. Nat. Commun. 7, 1–14. doi: 10.1038/ncomms10241
Blanch, A. J., Lenehan, C. E., and Quinton, J. S. (2010). Optimizing surfactant concentrations for dispersion of single-walled carbon nanotubes in aqueous solution. J. Phys. Chem. B 114, 9805–9811. doi: 10.1021/jp104113d
Boghossian, A. A., Zhang, J., Barone, P. W., Reuel, N. F., Kim, J. H., Heller, D. A., et al. (2011). Near-infrared fluorescent sensors based on single-walled carbon nanotubes for life sciences applications. ChemSusChem 4, 848–863. doi: 10.1002/cssc.201100070
Bonis-O'Donnell, J. T., Page, R. H., Beyene, A. G., Tindall, E. G., McFarlane, I. R., and Landry, M. P. (2017). Dual near-infrared two-photon microscopy for deep-tissue dopamine nanosensor imaging. Adv. Funct. Mater. 27, 1–10. doi: 10.1002/adfm.201702112
Bonis-O'Donnell, J. T. D., Pinals, R., Jeong, S., Thakrar, A., Wolfinger, R., and Landry, M. P. (2019). Chemometric approaches for developing infrared nanosensors to image anthracyclines. Biochemistry 58, 54–64. doi: 10.1021/acs.biochem.8b00926
Chattopadhyay, D., Galeska, I., and Papadimitrakopoulos, F. (2003). A route for bulk separation of semiconducting from metallic single-wall carbon nanotubes. J. Am. Chem. Soc. 125, 3370–3375. doi: 10.1021/ja028599l
Chio, L., Del Bonis-O'Donnell, J. T., Kline, M. A., Kim, J. H., McFarlane, I. R., Zuckermann, R. N., et al. (2019). Electrostatic assemblies of single-walled carbon nanotubes and sequence-tunable peptoid polymers detect a lectin protein and its target sugars. Nano Lett. doi: 10.1021/acs.nanolett.8b04955. [Epub ahead of print].
Choi, J. H., and Strano, M. S. (2007). Solvatochromism in single-walled carbon nanotubes. Appl. Phys. Lett.90, 88–91. doi: 10.1063/1.2745228
Coleman, J. N. (2009). Liquid-phase exfoliation of nanotubes and graphene. Adv. Funct. Mater. 19, 3680–3695. doi: 10.1002/adfm.200901640
Crochet, J., Clemens, M., and Hertel, T. (2007). Quantum yield heterogeneities of aqueous single-wall carbon nanotube suspensions. J. Am. Chem. Soc. 129, 8058–8059. doi: 10.1021/ja071553d
Dong, L., Joseph, K. L., Witkowski, C. M., and Craig, M. M. (2008). Cytotoxicity of single-walled carbon nanotubes suspended in various surfactants. Nanotechnology 19:255702. doi: 10.1088/0957-4484/19/25/255702
Dong, L., Witkowski, C. M., Craig, M. M., Greenwade, M. M., and Joseph, K. L. (2009). Cytotoxicity effects of different surfactant molecules conjugated to carbon nanotubes on human astrocytoma cells. Nanoscale Res. Lett. 4, 1517–1523. doi: 10.1007/s11671-009-9429-0
Dresselhaus, M. S., Dresselhaus, G., and Saito, R. (1992). Carbon fibers based on C60 and their symmetry. Phys. Rev. B 45, 6234–6242. doi: 10.1103/PhysRevB.45.6234
Enyashin, A. N., Gemming, S., and Seifert, G. (2007). DNA-wrapped carbon nanotubes. Nanotechnology 18:24. doi: 10.1088/0957-4484/18/24/245702
Etheridge, A., Lee, I., Hood, L., Galas, D., and Wang, K. (2011). Extracellular microRNA: a new source of biomarkers. Mutat Res. 717, 85–90. doi: 10.1016/j.mrfmmm.2011.03.004
Gao, Y., and Kyratzis, I. (2008). Covalent immobilization of proteins on carbon nanotubes using the cross-linker 1-ethyl-3-(3-dimethylaminopropyl)carbodiimide–a critical assessment. Bioconjug. Chem. 19, 1945–50. doi: 10.1021/bc800051c
Ghosh, S., and Chakrabarti, R. (2016). Spontaneous unzipping of xylonucleic acid assisted by a single-walled carbon nanotube: a computational study. J. Phys. Chem. B 120, 3642–3652. doi: 10.1021/acs.jpcb.6b02035
Gillen, A. J., Kupis-Rozmyslowicz, J., Gigli, C., Schürgers, N., and Boghossian, A. A. (2018). Xeno nucleic acid nanosensors for enhanced stability against ion-induced perturbations. J. Phys. Chem. Lett. 2018, 4336–4343. doi: 10.1021/acs.jpclett.8b01879
Giraldo, J. P., Landry, M. P., Kwak, S. Y., Jain, R. M., Wong, M. H., Iverson, N. M., et al. (2015). A ratiometric sensor using single chirality near-infrared fluorescent carbon nanotubes: application to in vivo monitoring. Small 11, 3973–3984. doi: 10.1002/smll.201403276
Haggenmueller, R., Rahatekar, S. S., Fagan, J. A., Chun, J., Becker, M. L., Naik, R. R., et al. (2008). Comparison of the quality of aqueous dispersions of single wall carbon nanotubes using surfactants and biomolecules. Langmuir 24, 5070–5078. doi: 10.1021/la703008r
Hamada, N., Sawada, S. I., and Oshiyama, A. (1992). New one-dimensional conductors: graphitic microtubules. Phys. Rev. Lett. 68, 1579–1581. doi: 10.1103/PhysRevLett.68.1579
Hamam, R., Hamam, D., Alsaleh, K. A., Kassem, M., Zaher, W., Alfayez, M., et al. (2017). Circulating microRNAs in breast cancer : novel diagnostic and prognostic biomarkers. Cell Death Dis. 8:e3045. doi: 10.1038/cddis.2017.440
Harvey, J. D., Jena, P. V., Baker, H. A., Zerze, G. H., Williams, R. M., Galassi, T. V., et al. (2017). A carbon nanotube reporter of microRNA hybridization events in vivo. Nat. Biomed. Eng. 1, 1–11. doi: 10.1038/s41551-017-0041
Hayes, J., Peruzzi, P. P., and Lawler, S. (2014). MicroRNAs in cancer: biomarkers, functions and therapy. Trends Mol. Med. 20, 460–469. doi: 10.1016/j.molmed.2014.06.005
Heller, D. A., Jeng, E. S., Yeung, T.-K., Martinez, B. M., Moll, A. E., Gastala, J. B., et al. (2006). Optical detection of DNA conformational polymorphism on single-walled carbon nanotubes. Science 311, 508–511. doi: 10.1126/science.1120792
Heller, D. A., Mayrhofer, R. M., Baik, S., Grinkova, Y. V., Usrey, M. L., and Strano, M. S. (2004). Concomitant length and diameter separation of single-walled carbon nanotubes. J. Am. Chem. Soc. 126, 14567–14573. doi: 10.1021/ja046450z
Hertel, T., Himmelein, S., Ackermann, T., Stich, D., and Crochet, J. (2010). Diffusion limited photoluminescence quantum yields in 1-D semiconductors : single-wall carbon nanotubes. ACS Nano 4, 7161–7168. doi: 10.1021/nn101612b
Hirsch, A. (2002). Functionalization of single-walled carbon nanotubes. Angew. Chem. Int. Ed. 41, 1853–1859. doi: 10.1002/1521-3773(20020603)41:11<1853::AID-ANIE1853>3.0.CO;2-N
Holt, B. D., McCorry, M. C., Boyer, P. D., Dahl, K. N., and Islam, M. F. (2012). Not all protein-mediated single-wall carbon nanotube dispersions are equally bioactive. Nanoscale 4, 7425–7434. doi: 10.1039/C2NR31928D
Huang, W., Taylor, S., Fu, K., Lin, Y., Zhang, D., Hanks, T. W., et al. (2002). Attaching proteins to carbon nanotubes via diimide-activated amidation. Nano Lett. 2, 311–314. doi: 10.1021/nl010095i
Huang, X., Mclean, R. S., and Zheng, M. (2005). High-resolution length sorting and purification of DNA-wrapped carbon nanotubes by size-exclusion chromatography. Anal. Chem. 77, 6225–6228. doi: 10.1021/ac0508954
Islam, M. F., Rojas, E., Bergey, D. M., Johnson, A. T., and Yodh, A. G. (2003). High weight fraction surfactant solubilization of single-wall carbon nanotubes in water. Nano Lett. 3, 269–273. doi: 10.1021/nl025924u
Iverson, N. M., Barone, P. W., Shandell, M. A., Trudel, L. J., Sen, S., Sen, F., et al. (2013). In vivo biosensing via tissue localizable near infrared fluorescent single walled carbon nanotubes. Nat. Nanotechnol. 8, 873–880. doi: 10.1038/nnano.2013.222
Jena, P. V., Safaee, M. M., Heller, D. A., and Roxbury, D. (2017). DNA-carbon nanotube complexation affinity and photoluminescence modulation are independent. ACS Appl. Mater. Interfaces 9, 21397–21405. doi: 10.1021/acsami.7b05678
Jeng, E. S., Moll, A. E., Roy, A. C., Gastala, J. B., and Strano, M. S. (2006). Detection of DNA hybridization using the near-infrared band-gap fluorescence of single-walled carbon nanotubes. Nano Lett. 6, 371–375. doi: 10.1021/nl051829k
Jeng, E. S., Nelson, J. D., Prather, K. L., and Strano, M. S. (2010). Detection of a single nucleotide polymorphism using single-walled carbon-nanotube near-infrared fluorescence. Small 6, 40–43. doi: 10.1002/smll.20090094
Jiang, K., Schadler, L. S., Siegel, R. W., Zhang, X., Zhang, H., and Terrones, M. (2004). Protein immobilization on carbon nanotubes via a two-step process of diimide-activated amidation. J. Mater. Chem. 14, 37–39. doi: 10.1039/B310359E
Johnson, R. R., Johnson, A. T. C., and Klein, M. L. (2008). Probing the structure of DNA-carbon nanotube hybrids with molecular dynamics. Nano Lett. 8, 69–75. doi: 10.1021/nl071909j
Johnson, R. R., Kohlmeyer, A., Johnson, A. T. C., and Klein, M. L. (2009). Free energy landscape of a {DNA}–carbon nanotube hybrid using replica exchange molecular dynamics. Nano Lett. 9, 537–541. doi: 10.1021/nl802645d
Kataura, H., Kumazawa, Y., Maniwa, Y., Umezu, I., Suzuki, S., Ohtsuka, Y., et al. (1999). Optical properties of single-wall carbon nanotubes. Synth. Metals 103, 2555–2558. doi: 10.1016/S0379-6779(98)00278-1
Kato, Y., Inoue, A., Niidome, Y., and Nakashima, N. (2012). Thermodynamics on soluble carbon nanotubes: how do DNA molecules replace surfactants on carbon nanotubes? Sci. Rep. 2, 1–7. doi: 10.1038/srep00733
Khripin, C. Y., Fagan, J. A., and Zheng, M. (2013). Spontaneous partition of carbon nanotubes in polymer-modified aqueous phases. J. Am. Chem. Soc. 135, 6822–6825. doi: 10.1021/ja402762e
Kim, J. H., Ahn, J. H., Barone, P. W., Jin, H., Zhang, J., Heller, D. A., et al. (2010). A luciferase/single-walled carbon nanotube conjugate for nearInfrared fluorescent detection of cellular ATP. Angew. Chem. Int. Ed. 49, 1456–1459. doi: 10.1002/anie.200906251
Kim, J. H., Heller, D. A., Jin, H., Barone, P. W., Song, C., Zhang, J., et al. (2009). The rational design of nitric oxide selectivity in single-walled carbon nanotube near-infrared fluorescence sensors for biological detection. Nat. Chem. 1, 473–481. doi: 10.1038/nchem.332
Krupke, R., Hennrich, F., Kappes, M. M., and Löhneysen, H. V. (2004). Surface conductance induced dielectrophoresis of semiconducting single-walled carbon nanotubes. Nano Lett. 4, 1395–1399. doi: 10.1021/nl0493794
Krupke, R., Hennrich, F., Löhneysen, H. V., and Kappes, M. M. (2003). Separation of metallic from semiconducting single-walled carbon nanotubes. Science 301, 344–347. doi: 10.1126/science.1086534
Kruss, S., Hilmer, A. J., Zhang, J., Reuel, N. F., Mu, B., and Strano, M. S. (2013). Carbon nanotubes as optical biomedical sensors. Adv. Drug Deliv. Rev. 65, 1933–1950. doi: 10.1016/j.addr.2013.07.015
Kruss, S., Landry, M. P., Vander Ende, E., Lima, B. M. A., Reuel, N. F., Zhang, J., et al. (2014). Neurotransmitter detection using corona phase molecular recognition on fluorescent single-walled carbon nanotube sensors. J. Am. Chem. Soc. 136, 713–724. doi: 10.1021/ja410433b
Kruss, S., Salem, D. P., Vuković, L., Lima, B., Vander Ende, E., Boyden, E. S., et al. (2017). High-resolution imaging of cellular dopamine efflux using a fluorescent nanosensor array. Proc. Natl. Acad. Sci. U.S.A. 114, 1789–1794. doi: 10.1073/pnas.1613541114
Kupis-Rozmysłowicz, J., Antonucci, A., and Boghossian, A. A. (2016). Review - engineering the selectivity of the DNA-SWCNT sensor. ECS J. Solid State Sci. Technol. 5, M3067–M3074. doi: 10.1149/2.0111608jss
Lambert, B., Gillen, A. J., Schuergers, N., Wu, S. J., and Boghossian, A. A. (2019). Directed evolution of the optoelectronic properties of synthetic nanomaterials. Chem. Commun. 55, 3239–3242. doi: 10.1039/C8CC08670B
Landry, M. P., Ando, H., Chen, A. Y., Cao, J., Kottadiel, V. I., Chio, L., et al. (2017). Single-molecule detection of protein efflux from microorganisms using fluorescent single-walled carbon nanotube sensor arrays. Nat. Nanotechnol. 12, 368–377. doi: 10.1038/nnano.2016.284
Landry, M. P., Kruss, S., Nelson, J. T., Bisker, G., Iverson, N. M., Reuel, N. F., et al. (2014). Experimental tools to study molecular recognition within the nanoparticle corona. Sensors 14, 16196–16211. doi: 10.3390/s140916196
Lee, K., Nojoomi, A., Jeon, J., Lee, C. Y., and Yum, K. (2018). Near-infrared fluorescence modulation of refolded DNA aptamer-functionalized single-walled carbon nanotubes for optical sensing. ACS Appl. Nano Mater. 1:9. doi: 10.1021/acsanm.8b01377
Li, C., and Shi, G. (2014). Carbon nanotube-based fluorescence sensors. J. Photochem. Photobiol. C Photochem. Rev. 19, 20–34. doi: 10.1016/j.jphotochemrev.2013.10.005
Liu, Z., Yang, K., and Lee, S.-T. (2011). Single-walled carbon nanotubes in biomedical imaging. J. Mater. Chem. 2011, 586–598. doi: 10.1039/C0JM02020F
Lustig, S. R., Jagota, A., Khripin, C., and Zheng, M. (2005). Theory of structure-based carbon nanotube separations by Ion-exchange chromatography of DNA/CNT hybrids. J. Phys. Chem. B 109, 2559–2566. doi: 10.1021/jp0452913
Ma, Q., Lee, D., Tan, Y. Q., Wong, G., and Gao, Z. (2016). Synthetic genetic polymers: advances and applications. Polym. Chem. 7, 5199–5216. doi: 10.1039/C6PY01075J
Maeda, Y., Kimura, S. I., Hiroshima, Y., Kanda, M., Lian, Y., Wakahara, T., et al. (2004). Dispersion of single-walled carbon nanotube bundles in nonaqueous solution. J. Phys. Chem. B 108, 18395–18397. doi: 10.1021/jp0457242
Mann, F. A., Herrmann, N., Meyer, D., and Kruss, S. (2017). Tuning selectivity of fluorescent carbon nanotube-based neurotransmitter sensors. Sensors 17:E1521. doi: 10.3390/s17071521
Matarredona, O., Rhoads, H., Li, Z., Harwell, J. H., Balzano, L., and Resasco, D. E. (2003). Dispersion of single-walled carbon nanotubes in aqueous solutions of the anionic surfactant NaDDBS. J. Phys. Chem. B 107, 13357–13367. doi: 10.1021/jp0365099
Mishra, P. J. (2014). MicroRNAs as promising biomarkers in cancer diagnostics. Biomarker Res. 2:19. doi: 10.1186/2050-7771-2-19
Mohamad, N. R., Marzuki, N. H. C., Buang, N. A., Huyop, F., and Wahab, R. A. (2015). An overview of technologies for immobilization of enzymes and surface analysis techniques for immobilized enzymes. Biotechnol. Biotechnol. Equip. 29, 205–220. doi: 10.1080/13102818.2015.1008192
Moore, V. C., Strano, M. S., Haroz, E. H., Hauge, R. H., Smalley, R. E., Schmidt, J., et al. (2003). Individually suspended single-walled carbon nanotubes in various surfactants. Nano Lett. 3, 1379–1382. doi: 10.1021/nl034524j
Nepal, D., and Geckeler, K. E. (2006). pH-sensitive dispersion and debundling of single-walled carbon nanotubes: lysozyme as a tool. Small 2, 406–412. doi: 10.1002/smll.200500351
Neves, L. F. F., Krais, J. J., Van Rite, B. D., Ramesh, R., Resasco, D. E., and Harrison, R. G. (2013). Targeting single-walled carbon nanotubes for the treatment of breast cancer using photothermal therapy. Nanotechnology 24:375104. doi: 10.1088/0957-4484/24/37/375104
Nonoguchi, Y., Tani, A., Murayama, T., Uchida, H., and Kawai, T. (2018). Surfactant-driven amphoteric doping of carbon nanotubes. Chem. Asian J. 13, 3942–3946. doi: 10.1002/asia.201801490
O'Connell, M. J., Bachilo, S. M., Huffman, C. B., Rialon, K. L., Boul, P. J., and Noon, W. H. (2002). Band gap fluorescence from individual single-walled carbon nanotubes. Science 297, 593–597. doi: 10.1126/science.1072631
O'Connell, M. J., Boul, P., Ericson, L. M., Huffman, C., Wang, Y., Haroz, E., et al. (2001). Reversible water-solubilization of single-walled carbon nanotubes by polymer wrapping. Chem. Phys. Lett. 342, 265–271. doi: 10.1016/S0009-2614(01)00490-0
Oh, H., Sim, J., and Ju, S. Y. (2013). Binding affinities and thermodynamics of noncovalent functionalization of carbon nanotubes with surfactants. Langmuir 29, 11154–11162. doi: 10.1021/la4022933
Pan, J., Li, F., and Choi, J. H. (2017). Single-walled carbon nanotubes as optical probes for bio-sensing and imaging. J. Mater. Chem. B 2017, 6511–6522. doi: 10.1039/C7TB00748E
Peng, H., Alvarez, N. T., Kittrell, C., Hauge, R. H., and Schmidt, H. K. (2006). Dielectrophoresis field flow fractionation of single-walled carbon nanotubes. J. Am. Chem. Soc. 128, 8396–8397. doi: 10.1021/ja0621501
Pinheiro, V. B., and Holliger, P. (2012). The XNA world: progress towards replication and evolution of synthetic genetic polymers. Curr. Opin. Chem. Biol. 16, 245–252. doi: 10.1016/j.cbpa.2012.05.198
Pinheiro, V. B., and Holliger, P. (2014). Towards XNA nanotechnology: new materials from synthetic genetic polymers. Trends Biotechnol. 32, 321–328. doi: 10.1016/j.tibtech.2014.03.010
Pinheiro, V. B., Loakes, D., and Holliger, P. (2013). Synthetic polymers and their potential as genetic materials. Bioessays 35, 113–122. doi: 10.1002/bies.201200135
Polo, E., and Kruss, S. (2016). Impact of redox-active molecules on the fluorescence of polymer-wrapped carbon nanotubes. J. Phys. Chem. C 120, 3061–3070. doi: 10.1021/acs.jpcc.5b12183
Safaee, M. M., Gravely, M., Rocchio, C., Simmeth, M., and Roxbury, D. (2019). DNA sequence mediates apparent length distribution in single-walled carbon nanotubes. ACS Appl. Mater. Interfaces 11, 2225–2233. doi: 10.1021/acsami.8b16478
Saifuddin, N., Raziah, A. Z., and Junizah, A. R. (2013). Carbon nanotubes: a review on structure and their interaction with proteins. J. Chem. 2013:676815. doi: 10.1155/2013/676815
Saito, R., Dresselhaus, G., and Dresselhaus, M. S. (1992). Electronic structure of double-layer graphene tubules. J. Appl. Phys. 60, 2204–2206. doi: 10.1063/1.107080
Salem, D. P., Gong, X., Liu, A. T., Koman, V. B., Dong, J., and Strano, M. S. (2017). Ionic strength mediated phase transitions of surface adsorbed DNA on single-walled carbon nanotubes. J. Am. Chem. Soc. 139, 16791–16802. doi: 10.1021/jacs.7b09258
Strano, M. S., Zheng, M., Jagota, A., Onoa, G. B., Heller, D. A., Barone, P. W., et al. (2004). Understanding the nature of the DNA-assisted separation of single-walled carbon nanotubes using fluorescence and raman spectroscopy. Nano Lett. 4, 543–550. doi: 10.1021/nl034937k
Subbaiyan, N. K., Parra-Vasquez, A. N. G., Cambré, S., Cordoba, M. A., Yalcin, S. E., Hamilton, C. E., et al. (2015). Bench-top aqueous two-phase extraction of isolated individual single-walled carbon nanotubes. Nano Res. 8, 1755–1769. doi: 10.1007/s12274-014-0680-z
Sun, J., and Zuckermann, R. N. (2013). Peptoid polymers: A highly designable bioinspired material. ACS Nano 7, 4715–4732. doi: 10.1021/nn4015714
Sun, Z., Nicolosi, V., Rickard, D., Bergin, S. D., Aherne, D., and Coleman, J. N. (2008). Quantitative evaluation of surfactant-stabilized single-walled carbon nanotubes: Dispersion quality and its correlation with zeta potential. J. Phys. Chem. C 112, 10692–10699. doi: 10.1021/jp8021634
Taylor, A. I., Arangundy-Franklin, S., and Holliger, P. (2014). Towards applications of synthetic genetic polymers in diagnosis and therapy. Curr. Opin. Chem. Biol. 22, 79–84. doi: 10.1016/j.cbpa.2014.09.022
Tu, X., Manohar, S., Jagota, A., and Zheng, M. (2009). DNA sequence motifs for structure-specific recognition and separation of carbon nanotubes. Nature 460, 250–253. doi: 10.1038/nature08116
Tu, X., and Zheng, M. (2008). A DNA-based approach to the carbon nanotube sorting problem. Nano Res. 1, 185–194. doi: 10.1007/s12274-008-8022-7
Tummala, N. R., Morrow, B. H., Resasco, D. E., and Striolo, A. (2010). Stabilization of aqueous carbon nanotube dispersions using surfactants: insights from molecular dynamics simulations. ACS Nano 4, 7193–7204. doi: 10.1021/nn101929f
Tummala, N. R., and Striolo, A. (2008). Role of counterion condensation in the self-assembly of SDS surfactants at the water-graphite interface. J. Phys. Chem. B 112, 1987–2000. doi: 10.1021/jp077678m
Tummala, N. R., and Striolo, A. (2009). SDS surfactants on carbon nanotubes. ACS Nano 3, 595–602. doi: 10.1021/nn8007756
Ulissi, Z. W., Zhang, J., Sresht, V., Blankschtein, D., and Strano, M. S. (2015). 2D equation-of-state model for corona phase molecular recognition on single-walled carbon nanotube and graphene surfaces. Langmuir 31, 628–636. doi: 10.1021/la503899e
Vo, M. D., and Papavassiliou, D. V. (2017). Effects of temperature and shear on the adsorption of surfactants on carbon nanotubes. J. Phys. Chem. C 121, 14339–14348. doi: 10.1021/acs.jpcc.7b03904
Vo, M. D., Shiau, B., Harwell, J. H., and Papavassiliou, D. V. (2016). Adsorption of anionic and non-ionic surfactants on carbon nanotubes in water with dissipative particle dynamics simulation. J. Chem. Phys. 144:204701. doi: 10.1063/1.4949364
Wang, H., Zhou, W., Ho, D. L., Winey, K. I., Fischer, J. E., Glinka, C. J., et al. (2004). Dispersing single-walled carbon nanotubes with surfactants: a small angle neutron scattering study. Nano Lett. 4, 1789–1793. doi: 10.1021/nl048969z
Wang, J., Chen, J., and Sen, S. (2016). MicroRNA as biomarkers and diagnostics. J. Cell. Physiol. 231, 25–30. doi: 10.1002/jcp.25056
Wang, L., Yang, C. J., Medley, C. D., Benner, S. A., and Tan, W. (2005). Locked nucleic acid molecular beacons. J. Am. Chem. Soc. 127, 15664–15665. doi: 10.1021/ja052498g
Wenseleers, W., Vlasov, I. L., Goovaerts, E., Obraztsova, E. D., Lobach, A. S., and Bouwen, A. (2004). Efficient isolation and solubilization of pristine single-walled nanotubes in bile salt micelles. Adv. Funct. Mater. 14, 1105–1112. doi: 10.1002/adfm.200400130
White, B., Banerjee, S., O'Brien, S., Turro, N. J., and Herman, I. P. (2007). Zeta-potential measurements of surfactant-wrapped individual single-walled carbon nanotubes. J. Phys. Chem. C 111, 13684–13690. doi: 10.1021/jp070853e
Wildoer, J. W. G., Venema, L. C., Rinzler, A. G., Smalley, R. E., and Dekker, C. (1998). Electronic structure of atomically resolved carbonnanotubes. Nature 391, 59-62. doi: 10.1038/34139
Williams, R. M., Lee, C., and Heller, D. A. (2018). A fluorescent carbon nanotube sensor detects the metastatic prostate cancer biomarker uPA. ACS Sens. 3, 1838–1845. doi: 10.1021/acssensors.8b00631
Wu, S.-J., Schürgers, N., Lin, K.-H., Gillen, A. J., Corminboeuf, C., and Boghossian, A. A. (2018). Restriction enzyme analysis of double-stranded DNA on pristine single-walled carbon nanotubes. ACS Appl. Mater. Interfaces 10, 37386–37395. doi: 10.1021/acsami.8b12287
Xin, X., Xu, G., and Li, H. (2013). “Dispersion and property manipulation of carbon nanotubes by self-assemblies of amphiphilic molecules,” in Physical and Chemical Properties of Carbon Nanotubes, ed S. Suzuki (IntechOpen).
Xu, J., Mueller, R., Hazelbaker, E., Zhao, Y., Bonzongo, J. C. J., Clar, J. G., et al. (2017). Strongly bound sodium dodecyl sulfate surrounding single-wall carbon nanotubes. Langmuir 33, 5006–5014. doi: 10.1021/acs.langmuir.7b00758
Xu, Y., Pehrsson, P. E., Chen, L., Zhang, R., and Zhao, W. (2007). Double-stranded DNA single-walled carbon nanotube hybrids for optical hydrogen peroxide and glucose sensing. J. Phys. Chem. C 111, 8638–8643. doi: 10.1021/jp0709611
Yoon, H., Ahn, J. H., Barone, P. W., Yum, K., Sharma, R., Boghossian, A. A., et al. (2011). Periplasmic binding proteins as optical modulators of single-walled carbon nanotube fluorescence: amplifying a nanoscale actuator. Angew. Chem. Int. Ed. 50, 1828–1831. doi: 10.1002/anie.201006167
Yum, K., McNicholas, T. P., Mu, B., and Strano, M. S. (2013). Single-walled carbon nanotube-based near-infrared optical glucose sensors toward in vivo continuous glucose monitoring. J. Diabetes Sci. Technol. 7, 72–87. doi: 10.1177/193229681300700109
Yurekli, K., Mitchell, C. A., and Krishnamoorti, R. (2004). Small-angle neutron scattering from surfactant-assisted aqueous dispersions of carbon nanotubes. J. Am. Chem. Soc.126, 9902–9903. doi: 10.1021/ja047451u
Zhang, J., Boghossian, A. A., Barone, P. W., Rwei, A., Kim, J. H., Lin, D., et al. (2011). Single molecule detection of nitric oxide enabled by d(AT)15DNA adsorbed to near infrared fluorescent single-walled carbon nanotubes. J. Am. Chem. Soc. 133, 567–581. doi: 10.1021/ja1084942
Zhang, J., Landry, M. P., Barone, P. W., Kim, J.-H., Lin, S., Ulissi, Z. W., et al. (2013). Molecular recognition using corona phase complexes made of synthetic polymers adsorbed on carbon nanotubes. Nat. Nanotechnol. 8, 959–968. doi: 10.1038/nnano.2013.236
Zhang, M., Khripin, C. Y., Fagan, J. A., McPhie, P., Ito, Y., and Zheng, M. (2015). Single-step total fractionation of single-wall carbon nanotubes by countercurrent chromatography. Anal. Chem. 86, 3980–3984. doi: 10.1021/ac5003189
Zheng, M., Jagota, A., Semke, E. D., Diner, B. A., McLean, R. S., Lustig, S. R., et al. (2003a). DNA-assisted dispersion and separation of carbon nanotubes. Nat. Mater. 2, 338–342. doi: 10.1038/nmat877
Zheng, M., Jagota, A., Strano, M. S., Santos, A. P., Barone, P. W., Chou, S. G., et al. (2003b). Structure-based carbon nanotube sorting by sequence-dependent DNA assembly. Science 302, 1545–1548. doi: 10.1126/science.1091911
Zheng, M., and Semke, E. D. (2007). Enrichment of single chirality carbon nanotubes. J. Am. Chem. Soc. 129, 6084–6085. doi: 10.1021/ja071577k
Zheng, Y., Bachilo, S. M., and Weisman, R. B. (2017). Quenching of single-walled carbon nanotube fluorescence by dissolved oxygen reveals selective single-stranded DNA affinities. J. Phys. Chem. Lett. 8, 1952–1955. doi: 10.1021/acs.jpclett.7b00583
Keywords: optical biosensing, near-infrared sensors, single-walled carbon nanotubes (SWCNTs or SWNTs), molecular recognition, selectivity, fluorescence brightness, non-covalent solubilization
Citation: Gillen AJ and Boghossian AA (2019) Non-covalent Methods of Engineering Optical Sensors Based on Single-Walled Carbon Nanotubes. Front. Chem. 7:612. doi: 10.3389/fchem.2019.00612
Received: 29 January 2019; Accepted: 21 August 2019;
Published: 19 September 2019.
Edited by:
Fan Zhang, Fudan University, ChinaReviewed by:
Renren Deng, Zhejiang University, ChinaVictor Alexeevich Karachevtsev, B. Verkin Institute for Low Temperature Physics and Engineering (National Academy of Sciences Ukraine), Ukraine
Feng Long, Renmin University of China, China
Copyright © 2019 Gillen and Boghossian. This is an open-access article distributed under the terms of the Creative Commons Attribution License (CC BY). The use, distribution or reproduction in other forums is permitted, provided the original author(s) and the copyright owner(s) are credited and that the original publication in this journal is cited, in accordance with accepted academic practice. No use, distribution or reproduction is permitted which does not comply with these terms.
*Correspondence: Ardemis A. Boghossian, YXJkZW1pcy5ib2dob3NzaWFuQGVwZmwuY2g=