- 1Jiangsu Key Laboratory of Materials and Technology for Energy Conversion, College of Materials Science and Technology, Nanjing University of Aeronautics and Astronautics, Nanjing, China
- 2Photofunctional Materials Research Platform, College of Materials Science and Engineering, North China University of Science and Technology, Tangshan, China
- 3Testing Center, Yangzhou University, Yangzhou, China
The design of mesoporous or hollow transition metal oxide/carbon hybrid catalysts is very important for rechargeable Li-O2 batteries. Here, spindle-like Fe2O3 with hollow mesoporous structure on CNTs backbones (Fe2O3-HMNS@CNT) are prepared by a facile hydrolysis process combined with low temperature calcination. Within this hybrid structure, the hollow interior and mesoporous shell of the Fe2O3 nanospindles provide high specific surface area and abundant catalytical active sites, which is also beneficial to facilitating the electrolyte infiltration and oxygen diffusion. Furthermore, the crisscrossed CNTs form a three-dimensional (3D) conductive network to accelerate and stabilize the electron transport, which leads to the decreasing internal resistance of electrode. As a cathodic catalyst for Li-O2 batteries, the Fe2O3-HMNS@CNT composite exhibits high specific capacity and excellent cycling stability (more than 100 cycles).
Introduction
To meet the global energy demand, the development of the clean and sustainable energy storage or conversion devices is very important (Tarascon and Armand, 2011; Lu et al., 2014; Wang et al., 2017; Zhang et al., 2018; Gao et al., 2019). Rechargeable Li-O2 battery has attracted wide attention as a new energy storage device, due to its high theoretical energy density (~3,500 Wh kg−1) (Tarascon and Armand, 2011; Lu et al., 2014). However, the practical application of Li-O2 batteries still suffer a series of problems, including high overpotentials, low rate capacity and poor cycle stability, which primarily originates from its sluggish kinetics for oxygen reduction reaction (ORR) and oxygen evolution reaction (OER) (Bruce et al., 2012; Wang et al., 2014). At the cathode (air electrode), the gradual formation of the insoluble discharge products Li2O2 may block the inward oxygen diffusion of and electrolyte infiltration, which results in the rapid decline of battery performance (Zhao et al., 2015; Wang et al., 2016). To overcome these challenges, the design and development of the high-performance catalysts for oxygen-involved reactions are highly desired for the Li-O2 batteries.
In recent years, a lot of the efforts have been devoted to investigate the highly active and stable catalysts for the Li-O2 batteries, such as carbons, precious metals, and transition metal oxides. As the common catalysts, the inexpensive carbon materials have high surface area, nevertheless, their limited catalytic activity for both OER and ORR restricts the battery performance of the Li-O2 batteries (Girishkumar et al., 2010; Shui et al., 2013). Since the nanoporous gold (NPG) was used as a cathode catalyst, various precious metals (e.g., Ru, RuO, and Pd) have been adopted in the Li-O2 batteries (Peng et al., 2012; Lu et al., 2013; Ottakam Thotiyl et al., 2013; Li et al., 2014, 2015). Although the cyclic stability can be distinctly enhanced by precious metal catalysts, the battery capacity is severely restricted because of the highly chemical formula weight. Moreover, high price of precious metals also hinders the large-scale commercialization in the Li-O2 batteries. Benefiting from the low cost, high stability and good catalytic performance, transition metal oxides have been proposed as the promising catalysts for the Li-O2 batteries (Wang H. et al., 2012; Chen et al., 2016; Gong et al., 2016, 2018a,b; Xue et al., 2016a,c; Dai et al., 2017; Tan et al., 2017; Feng et al., 2019). Many researches have indicated that Fe-based materials possess high catalytic activities for ORR in fuel cells and OER in water electrolysis (Bates et al., 2016; Song et al., 2019). Recently, some reports began to focus on iron oxides (Fe2O3), which can serve as the cathode catalyst in Li-O2 batteries (Zhang et al., 2014). These works show the enhanced electrochemical performance (e.g., higher capacity and lower overpotentials) of the Li-O2 batteries, however the cycling performance still needs to further improve. Therefore, it is necessary to explore an effective approach to enhance the catalytic performance of Fe2O3-based materials.
Tailoring of the morphology is an important method for obtaining the high-performance catalysts in various electrochemical application. Mesoporous hollow architectures show high surface area and large pore volume, which offers fast electron transfer paths and facilitates the electrolyte infiltration (Kresge et al., 1992; Inagaki et al., 2002; Malgras et al., 2016). Normally, mesoporous or hollow metallic oxide are prepared through the template-based methods, using either hard templates (e.g., carbon sphere and mesoporous silica) or soft templates (e.g., surfactants and polymer) (Attard et al., 1997; Crossland et al., 2013; Liu et al., 2015; Xue et al., 2016d). For these template methods, the multi-step processes are unavoidable, and the mesoporous of hollow structures may be damaged after removing the templates. Moreover, the resultant mesoporous or hollow frameworks often show poor crystalline degree and even amorphous, which limits their electrochemical performance (Lin et al., 2015). It should be noted that the low electronic conductivity is an intrinsical characteristic of the most metallic oxides. Although most of pure carbon materials possess low catalytic activity for OER and ORR, they are identified as the good catalyst support due to their high electric conductivity and large specific surface area (Hsin et al., 2007; Stein et al., 2009; Wu et al., 2009; Xia et al., 2018). Among various carbon materials, the carbon nanotubes (CNTs) show low density, very high strength, high chemical stability, and excellent conductivity (Sathiya et al., 2011; Wang Z. et al., 2012; Ma et al., 2018). These advantages are beneficial to fabricate hybrid or composite materials in many applications by using CNTs as useful substrates. However, the fabrication of mesoporous hollow Fe2O3 with high crystalline degree supported on CNTs by a simple and effective method is still an important challenge.
Inspired by the above idea, we proposed a Fe2O3/CNTs composite (denoted as Fe2O3-HMNS@CNT) prepared by using a simple hydrolysis reaction combined with heat treatment, in which spindle-like Fe2O3 with hollow mesoporous structure grown on CNTs backbones. Their hollow interior and mesoporous shell with high specific surface area offer abundant catalytical active sites for OER and ORR, which also promotes the diffusion and infiltration of electrolyte. Furthermore, a great deal of the crisscrossed CNTs form the three-dimensional (3D) conductive network, which benefits the fast and stable electron transport. As a cathodic catalyst for Li-O2 batteries, the Fe2O3-HMNS@CNT exhibits good battery performance, especially excellent outstanding cycling stability (100 cycles).
Experimental Methods
Hollow Mesoporous Fe2O3 Nanospindles on CNT Backbones
Carbon nanotubes (CNTs, 30–60 nm in diameter and 5–15 μm in length) were purchased from Shenzhen Nanotech Port Co. Ltd (Shenzhen, China). CNTs were refluxed in HNO3 and then rinsed with distilled water. After drying, the obtained CNTs (10 mg) was dispersed in FeCl3 solution (20 mL, 0.12 M) under sonication for 1 h. Then, the suspension was heated at 75°C for 6 h in an oil bath with stirring. After several rinsing combined with sonication, the products were dried at 60°C. Finally, the above products were annealed at 400°C for 4 h in air by using a slow heating rate (0.5°C min−1) to form hollow mesoporous Fe2O3 nanospindles on CNT backbones.
Materials Characterization
The X-ray diffraction (XRD, Bruker D8 advance) with Cu Ka radiation (λ = 1.5406 Å) is used to analyze the crystal structure of the sample. The N2 adsorption–desorption measurements conducted on a ASAP-2010 analyzer to investigate the pore structure. The Brunauer-Emmet-Teller (BET) method is used to calculate the specific surface area. The morphology and microstructure are observed by using field-emission scanning electron microscope (FE-SEM, Hitachi S-4800) and high-resolution transmission electron microscope (HR-TEM, JEOL JEM-2100), respectively.
Electrochemical Measurement
The electrochemical measurements are tested under two-electrode system at room temperature in the pure O2 atmosphere, using Li plate as the counter and reference electrode. The work electrode is prepared by mixing Fe2O3-HMNS@CNT catalyst (50 wt %), Super P (45 wt %) and polytetrafluoroethylene (PTFE) binder (5 wt %), followed by vacuum drying at 100°C for 12 h. The capacity and current density of the sample is calculated by the whole electrode' weight. For the assembling of Li-O2 battery, the working electrode and Li plate are separated by a glass-fiber separator in coin cell. The tetraethylene glycol dimethyl ether (TEGDME) containing lithium bis(tri-fluoromethane-sulfonyl)imide (LiTFSI) (1 M) is used as electrolyte. The Land 2100 Charge/Discharge instruments are carried to test the electrochemical measurements.
Results and Discussion
Figure 1 illustrates the fabrication of the mesoporous hollow Fe2O3 nanocrystals covered on CNT by a simple hydrolysis reaction combined with heat treatment. In order to facilitate the nucleation and anchoring of the nanocrystals on the CNT surface, these CNTs are functionally modified with carboxylic or hydroxyl groups under acidic reflux. During the subsequent stirring process, the electropositive Fe3+ ions can preferential adsorb the electronegative oxygen-containing groups on CNTs by the electrostatic attraction. Due to the hydrolysis of Fe3+ ions followed by the olation/oxolation of the FeO6 units, the formed spindle-like β-FeOOH nanocrystals can be spontaneously grown on CNT backbones, which avoids the addition of any structure-directing agent. The TG/DSC is used to explore the reactions in the final annealing step (Figure S1). The TG curve show two mainly weight loss processes at 300°C and 300~400°C during the annealing step, which are attributed to the conversion from FeOOH into Fe2O3. The slowly intramolecular dehydration results in the slow weight loss before 300°C. After 300°C, a distinct weight loss can be found, which is originated from the fast removal of the H2O molecules. After annealing treatment in air, the spindle-like β-FeOOH nanocrystals are converted to hollow mesoporous α-Fe2O3 nanospindles on CNT backbones (denoted as Fe2O3-HMNS@CNT) by the thermal dehydroxylation together with the lattice shrinkage.
The crystalline structure of the Fe2O3-HMNS@CNT composite is confirmed by XRD analysis. As shown in Figure 2A, the XRD pattern exhibits several intensively diffraction peaks at the 2θ values of about 24.2, 33.2, 35.6, 39.3, and 40.9°, which are indexed to the (012), (104), (110), (006), and (113) facets of the hexagonal α-Fe2O3. This result of XRD is in accord with the standard card of α-Fe2O3 (JCPDS no. 33-0664). The pore structure of the Fe2O3-HMNS@CNT composite is analyzed by N2 adsorption–desorption isotherms (Figure 2B). It can be seen that there is a type-IV curve of the Fe2O3-HMNS@CNT with a distinctly hysteresis loop on N2 adsorption–desorption isotherms, which indicates the typically mesoporous structure (Deng et al., 2007; Xue et al., 2016b). Moreover, the nitrogen uptake is found from 0.40 to 0.70 (P/Po), which can be attributed to the mesoporous materials' capillary condensation of nitrogen. Based on N2 adsorption–desorption isotherms, the specific surface area is calculated to be 97 m2 g−1. The above results indicate that the Fe2O3-HMNS@CNT has a typically mesoporous structure with high specific surface area.
The morphologies and structures of the Fe2O3-HMNS@CNT composite are observed by scanning electron microscopy (SEM) and transmission electron microscopy (TEM). As shown in Figure S2, the CNT shows the typical one-dimensional tubular structure with a diameter of ~40 nm. Figure 3a reveals a panoramic view of the sample, in which the spindle-like nanocrystals grown on the entire surface of the sinuous CNTs backbones. It can be noted that there are many obvious porous within the Fe2O3 nanospindles, as shown in Figures 3b,c. TEM observation is used to further investigate the internal structure of the sample. The spindle-like Fe2O3 nanocrystals have a diameter of around 80 nm and a length of about 250 nm (Figure 3d), which show a well-developed hollow interior (marked by orange dotted line) and a typically mesoporous shell (marked by purple dotted line) (Figure 3e). During the calcining process, the decomposition-oxidation of the FeOOH can release abundant H2O and gases, which leads to the formation of mesoporous structure. On the other hand, the density of FeOOH (3 g cm−3) is lower than that of the hematite (Fe2O3, 5.3 g cm−3), so some internal mesoporous slowly forms the larger porous to maintain the spindle-like structure during the lattice shrinkage process, thus leading to the formation of the hollow interior together with the mesoporous shell. As shown in Figure 3f, some clear lattice fringes with an interplanar spacing of 0.251 nm in a single Fe2O3 nanospindle, corresponding to the (110) planes of the hexagonal α-Fe2O3, which confirms the formation of the α-Fe2O3 with high crystallinity.
Inspired by the superiorities of material and structure, the Fe2O3-HMNS@CNT composite is investigated as the cathodic catalyst for rechargeable Li-O2 batteries. Figure 4A shows the discharge and charge curves of the sample, which is tested under O2 atmosphere with a current density of 200 mA g−1. The Fe2O3-HMNS@CNT-based cathode exhibits a large discharge capacity of 7,730 mAh g−1 and a high discharge plateau (~2.70 V) with the low overpotential for discharging (0.26 V). Owing to the low overpotential (1.10 V) for the charge process, the coulombic efficiency of the sample is closed to 100%. The reversible formation/decomposition of the discharging product Li2O2 can be confirmed by SEM (inset of Figure 4A). After fully discharging (defined as II), it is seen that a mass of the Li2O2 particles with the typically toroid-like shape uniformly cover on the electrode surface, which is observably different from the fresh electrode (defined as I). When the electrode is fully charged, the formed Li2O2 particles is rarely observed (defined as III), indicating high reversibility of the Fe2O3-HMNS@CNT-based cathode. We further evaluate the cycling performance of the Fe2O3-HMNS@CNT-based cathode, tesed under the limited capacity (1,000 mAh g−1). It can be found that the sample has the small discharge and charge overpotentials of 0.24 and 0.93 V in the first cycle, respectively, which implies the high catalytic activity for both OER and ORR (Figure 4B). After 100 cycles, no distinct change of the specific capacities of the sample is observed in discharging/charging curves. Moreover, the discharging and charging terminal voltages can still maintain in 2.6 and 4.3 V. These results exhibit the excellent cycling stability of the Fe2O3-HMNS@CNT-based cathode (Figure 4C). As a reference, the cycling stability of the Fe2O3-HMNS@CNT-based cathode is much better than those of previously reported non-precious metal/metal oxide-based materials (Table S1).
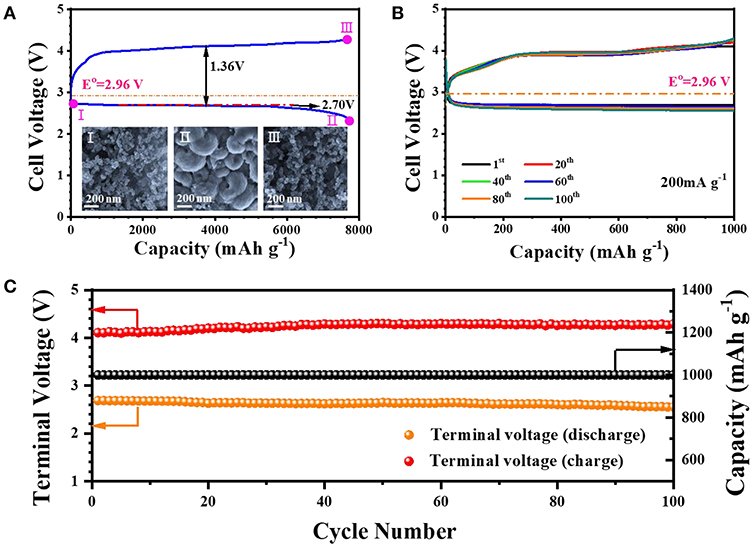
Figure 4. (A) The fully discharging and charging curves in the first cycle and the SEM images [inset of (A)], (B) cyclic stability tested under a limited capacity (1,000 mAh g−1), and (C) the variation of the terminal charge/discharge voltages and specific capacity over 100 cycles of the Fe2O3-HMNS@CNT-based cathode.
As the cathodic catalyst for rechargeable Li-O2 batteries, the as-prepared Fe2O3-HMNS@CNT composite exhibits the excellent cycling stability, which not only benefits by the intrinsic material characteristics but also dependents on the morphology and structure. On the one hand, these largely separated Fe2O3 nanospindles directly grown on the CNT backbones, which makes them accessible to the electrolyte. Different from the conventional nanoparticle catalysts, the adverse agglomeration of the Fe2O3-HMNS@CNT can be effectively decreased, avoiding the elimination of the active interfaces. The Fe2O3 with a hollow interior and a mesoporous shell offers high specific surface area and a mass of catalytical active sites, which also facilitates the diffusion and infiltration of electrolyte. On the other hand, the 3D conductive network composed of the crisscrossed CNTs ensures the fast and stable electron transport, leading to the lower internal resistance of electrode.
Conclusion
In summary, we successfully fabricated hollow mesoporous Fe2O3 nanospindles on CNTs (Fe2O3-HMNS@CNT) though a simple hydrolysis reaction followed by a heat treatment, which are served as cathodic catalyst for Li-O2 batteries. In this catalyst design concept, the spindle-like Fe2O3 nanocrystals possess the hollow interior and mesoporous shell, which not only provides high specific surface area and abundant catalytical active sites but also facilitates the diffusion and infiltration of electrolyte. Moreover, the 3D conductive network formed by the crisscrossed CNTs ensures the fast and stable electron transport, reducing internal resistance of electrode. Benefiting from the intrinsic material characteristics and structural superiorities, the Fe2O3-HMNS@CNT catalyst shows high specific capacity and excellent cyclic stability.
Data Availability
All datasets generated for this study are included in the manuscript/Supplementary Files.
Author Contributions
HX, TW, and JH conceived this research work. HX performed the experiments and wrote the manuscript. YM, HX, HG, BG, XF, and JY tested the electrochemical performance. XM and SZ contributed to analyze the experimental results. All authors read and approved the final manuscript.
Funding
This work was financially supported by the Zhejiang Provincial Natural Science Foundation of China under Grant no. LQ18B010005, National Natural Science Foundation of China (51602153, 11575084, and 21703065), the Natural Science Foundation of Jiangsu Province (BK20160795), the Fundamental Research Funds for the Central Universities (no. NE2018104), and a project funded by the Priority Academic Program Development of Jiangsu Higher Education Institutions (PAPD).
Conflict of Interest Statement
The authors declare that the research was conducted in the absence of any commercial or financial relationships that could be construed as a potential conflict of interest.
Supplementary Material
The Supplementary Material for this article can be found online at: https://www.frontiersin.org/articles/10.3389/fchem.2019.00511/full#supplementary-material
References
Attard, G., Bartlett, P., Coleman, N., Elliott, J., Owen, J., and Wang, J. (1997). Mesoporous platinum films from lyotropic liquid crystalline phases. Science. 278, 838–840. doi: 10.1126/science.278.5339.838
Bates, M., Jia, Q., Doan, H., Liang, W., and Mukerjee, S. (2016). Charge-transfer effects in Ni-Fe and Ni-Fe-Co mixed-metal oxides for the alkaline oxygen evolution reaction. ACS Catal. 6, 155–161. doi: 10.1021/acscatal.5b01481
Bruce, P. G., Freunberger, S. A., Hardwick, L. J., and Tarascon, J. M. (2012). Li-O2 and Li-S batteries with high energy storage. Nat. Mater. 11, 19–29. doi: 10.1038/nmat3191
Chen, Y., Pang, W. K., Bai, H., Zhou, T., Liu, Y., Li, S., et al. (2016). Enhanced structural stability of nickel-cobalt hydroxide via intrinsic pillar effect of metaborate for high-power and long-life supercapacitor electrodes. Nano Lett. 17, 429–436. doi: 10.1021/acs.nanolett.6b04427
Crossland, E. J., Noel, N., Sivaram, V., Leijtens, T., Alexander-Webber, J. A., and Snaith, H. J. (2013). Mesoporous TiO2 single crystals delivering enhanced mobility and optoelectronic device performance. Nature 495, 215–219. doi: 10.1038/nature11936
Dai, Z., Geng, H., Wang, J., Luo, Y., Li, B., Zong, Y., et al. (2017). Hexagonal-phase cobalt monophosphosulfide for highly efficient overall water splitting. ACS. Nano 11, 11031–11040. doi: 10.1021/acsnano.7b05050
Deng, Y., Liu, C., Yu, T., Liu, F., Zhang, F., Wan, Y., et al. (2007). Facile synthesis of hierarchically porous carbons from dual colloidal crystal/block copolymer template approach. Chem. Mater. 19, 3271–3277. doi: 10.1021/cm070600y
Feng, Y., Xue, H., Wang, T., Gong, H., Gao, B., Xia, W., et al. (2019). Enhanced Li2O2 decomposition in rechargeable Li-O2 battery by incorporating WO3 nanowire array photocatalyst. ACS Sustain. Chem. Eng. 7, 5931–5939. doi: 10.1021/acssuschemeng.8b05944
Gao, B., Wang, T., Fan, X., Gong, H., Li, P., Feng, Y., et al. (2019). Enhanced water oxidation reaction kinetics on a BiVO4 photoanode by surface modification with Ni4O4 cubane. J. Mater. Chem. A 7, 278–288. doi: 10.1039/C8TA09404G
Girishkumar, G., McCloskey, B., Luntz, A., Swanson, S., and Wilcke, W. (2010). Lithium-air battery: promise and challenges. J. Phys. Chem. Lett. 1, 2193–2203. doi: 10.1021/jz1005384
Gong, H., Wang, T., Guo, H., Fan, X., Liu, X., Song, L., et al. (2018a). Fabrication of perovskite-based porous nanotubes as efficient bifunctional catalyst and application in hybrid lithium-oxygen batteries. J. Mater. Chem. A 6, 16943–16949. doi: 10.1039/C8TA04599B
Gong, H., Wang, T., Xue, H., Fan, X., Gao, B., Zhang, H., et al. (2018b). Photo-enhanced lithium oxygen batteries with defective titanium oxide as both photo-anode and air electrode. Energy Storage Mater. 13, 49–56. doi: 10.1016/j.ensm.2017.12.025
Gong, H., Xue, H., Wang, T., Guo, H., Fan, X., Song, L., et al. (2016). High-loading nickel cobaltate nanoparticles anchored on three-dimensional N-doped graphene as an efficient bifunctional catalyst for lithium-oxygen batteries. ACS Appl. Mater. Interfaces 8, 18060–18068. doi: 10.1021/acsami.6b04810
Hsin, Y. L., Hwang, K. C., and Yeh, C. T. (2007). Poly (vinylpyrrolidone)–modified graphite carbon nanofibers as promising supports for PtRu catalysts in direct methanol fuel cells. J. Am. Chem. Soc. 129, 9999–10010. doi: 10.1021/ja072367a
Inagaki, S., Guan, S., Ohsuna, T., and Terasaki, O. (2002). An ordered mesoporous organosilica hybrid material with a crystal-like wall structure. Nature 416, 304–307. doi: 10.1038/416304a
Kresge, C., Leonowicz, M., Roth, W., Vartuli, J., and Beck, J. (1992). Ordered mesoporous molecular sieves synthesized by a liquid-crystal template mechanism. Nature 359, 710–712. doi: 10.1038/359710a0
Li, F., Tang, D., Jian, Z., Liu, D., Golberg, D., Yamada, A., et al. (2014). Li-O2 battery based on highly efficient Sb-doped Tin oxide supported Ru nanoparticles. Adv. Mater. 26, 4659–4664. doi: 10.1002/adma.201400162
Li, F., Tang, D., Zhang, T., Liao, K., He, P., Golberg, D., et al. (2015). Superior performance of a Li-O2 battery with metallic RuO2 hollow spheres as the carbon-free cathode. Adv. Energy Mater. 5:1500294. doi: 10.1002/aenm.201500294
Lin, J., Zhao, L., Heo, Y., Wang, L., Bijarbooneh, F., Mozer, A., et al. (2015). Mesoporous anatase single crystals for efficient Co(2+/3+)-based dye-sensitized solar cells. Nano Energy 11, 557–567. doi: 10.1016/j.nanoen.2014.11.017
Liu, J., Wickramaratne, N. P., Qiao, S. Z., and Jaroniec, M. (2015). Molecular-based design and emerging applications of nanoporous carbon spheres. Nat. Mater. 14, 763–774. doi: 10.1038/nmat4317
Lu, J., Lei, Y., Lau, K. C., Luo, X., Du, P., Wen, J., et al. (2013). Nanostructured cathode architecture for low charge overpotential in lithium-oxygen batteries. Nat. Commun. 4:2383. doi: 10.1038/ncomms3383
Lu, J., Li, L., Park, J., Sun, Y., Wu, F., and Amine, K. (2014). Aprotic and aqueous Li-O2 batteries. Chem. Rev. 114, 5611–5640. doi: 10.1021/cr400573b
Ma, L., Zhang, W., Wang, L., Hu, Y., Zhu, G., Wang, Y., et al. (2018). Strong capillarity, chemisorption, and electrocatalytic capability of crisscrossed nanostraws enabled flexible, high-rate, and long-cycling lithium-sulfur batteries. ACS Nano 12, 4868–4876. doi: 10.1021/acsnano.8b01763
Malgras, V., Ataee-Esfahani, H., Wang, H., Jiang, B., Li, C., Wu, K. C., et al. (2016). Nanoarchitectures for mesoporous metals. Adv. Mater. 28, 993–1010. doi: 10.1002/adma.201502593
Ottakam Thotiyl, M. M., Freunberger, S. A., Peng, Z., Chen, Y., Liu, Z., and Bruce, P. G. (2013). A stable cathode for the aprotic Li-O2 battery. Nat. Mater. 12, 1050–1056. doi: 10.1038/nmat3737
Peng, Z., Freunberger, S., Chen, Y., and Bruce, P. (2012). A reversible and higher-rate Li-O2 battery. Science 373, 563–566. doi: 10.1126/science.1223985
Sathiya, M., Prakash, A. S., Ramesha, K., Tarascon, J. M., and Shukla, A. K. (2011). V2O5-anchored carbon nanotubes for enhanced electrochemical energy storage. J. Am. Chem. Soc. 133, 16291–16299. doi: 10.1021/ja207285b
Shui, J. L., Okasinski, J. S., Kenesei, P., Dobbs, H. A., Zhao, D., Almer, J. D., et al. (2013). Reversibility of anodic lithium in rechargeable lithium-oxygen batteries. Nat. Commun. 4:2255. doi: 10.1038/ncomms3255
Song, L., Wang, T., Li, L., Wu, C., and He, J. (2019). [Fe(CN)6]2 derived Fe/Fe5C2@ N-doped carbon as a highly effective oxygen reduction reaction catalyst for zinc-air battery. Appl. Catal. B Environ. 244, 197–205. doi: 10.1016/j.apcatb.2018.11.005
Stein, A., Wang, Z., and Fierke, M. (2009). Functionalization of porous carbon materials with designed pore architecture. Adv. Mater. 21, 265–293. doi: 10.1002/adma.200801492
Tan, G., Chong, L., Amine, R., Lu, J., Liu, C., Yuan, Y., et al. (2017). Toward highly efficient electrocatalyst for Li-O2 batteries using biphasic N-doping cobalt@graphene multiple-capsule heterostructures. Nano Lett. 17, 2959–2966. doi: 10.1021/acs.nanolett.7b00207
Tarascon, J. M., and Armand, M. (2011). Issues and challenges facing rechargeable lithium batteries. Nature 414, 359–367. doi: 10.1038/35104644
Wang, H., Yang, Y., Liang, Y., Zheng, G., Li, Y., Cui, Y., et al. (2012). Rechargeable Li-O2 batteries with a covalently coupled MnCo2O4-graphene hybrid as an oxygen cathode catalyst. Energy Environ. Sci. 5, 7931–7935. doi: 10.1039/c2ee21746e
Wang, J., Zhang, Y., Guo, L., Wang, E., and Peng, Z. (2016). Identifying reactive sites and transport limitations of oxygen reactions in aprotic lithium-O2 batteries at the stage of sudden death. Angew. Chem. Int. Ed. 55, 5201–5205. doi: 10.1002/anie.201600793
Wang, X., Wang, Y., Bu, Y., Yan, X., Wang, J., Cai, P., et al. (2017). Influence of doping and excitation powers on optical thermometry in Yb3+-Er3+ doped CaWO4. Sci. Rep. 7:43383. doi: 10.1038/srep43383
Wang, Z., Luan, D., Madhavi, S., Hu, Y., and Lou, X. (2012). Assembling carbon-coated a-Fe2O3 hollow nanohorns on the CNT backbone for superior lithium storage capability. Energy Environ. Sci. 5, 5252–5256. doi: 10.1039/C1EE02831F
Wang, Z. L., Xu, D., Xu, J. J., and Zhang, X. B. (2014). Oxygen electrocatalysts in metal-air Batteries: from aqueous to nonaqueous electrolytes. Chem. Soc. Rev. 43, 7746–7786. doi: 10.1039/C3CS60248F
Wu, B., Hu, D., Kuang, Y., Liu, B., Zhang, X., and Chen, J. (2009). Functionalization of carbon nanotubes by an ionic-liquid polymer: dispersion of Pt and PtRu nanoparticles on carbon nanotubes and their electrocatalytic oxidation of methanol. Angew. Chem. Int. Ed. 48, 4751–4654. doi: 10.1002/anie.200900899
Xia, W., Li, J., Wang, T., Song, L., Guo, H., Gong, H., et al. (2018). The synergistic effect of Ceria and Co in N-doped leaf-like carbon nanosheets derived from a 2D MOF and their enhanced performance in the oxygen reduction reaction. Chem. Commun. 54, 1623–1626. doi: 10.1039/C7CC09212A
Xue, H., Mu, X., Tang, J., Fan, X., Gong, H., Wang, T., et al. (2016a). A nickel cobaltate nanoparticle-decorated hierarchical porous N-doped carbon nanofiber film as a binder-free self-supported cathode for non-aqueous Li-O2 batteries. J. Mater. Chem. A 4, 9106–9112. doi: 10.1039/C6TA01712F
Xue, H., Tang, J., Gong, H., Guo, H., Fan, X., Wang, T., et al. (2016b). Fabrication of PdCo bimetallic nanoparticles anchored on three-dimensional ordered N-doped porous carbon as an efficient catalyst for oxygen reduction reaction. ACS Appl. Mater. Interfaces. 8, 20766–20771. doi: 10.1021/acsami.6b05856
Xue, H., Wu, S., Tang, J., Gong, H., He, P., He, J., et al. (2016c). Hierarchical porous nickel cobaltate nanoneedle arrays as flexible carbon-protected cathodes for high-performance lithium-oxygen batteries. ACS. Appl. Mater. Interfaces. 8, 8427–8435. doi: 10.1021/acsami.5b10856
Xue, H., Zhao, J., Tang, J., Gong, H., He, P., Zhou, H., et al. (2016d). High-loading Nano-SnO2 encapsulated in situ in three-dimensional rigid porous carbon for superior lithium-ion batteries. Chem. Eur. J. 22, 4915–4923. doi: 10.1002/chem.201504420
Zhang, T., Wang, Y., Song, T., Miyaoka, H., Shinzato, K., Miyaoka, H., et al. (2018). Ammonia, a switch for controlling high ionic conductivity in lithium borohydride ammoniates. Joule 2, 1522–1533. doi: 10.1016/j.joule.2018.04.015
Zhang, Z., Zhou, G., Chen, W., Lai, Y., and Li, J. (2014). Facile synthesis of Fe2O3 nanoflakes and their electrochemical properties for Li-air batteries. ECS Electrochem. Lett. 3, A8–A10. doi: 10.1149/2.006401eel
Keywords: hollow mesoporous structure, carbon support, transition metal oxides, cathodic catalyst, Li-O2 batteries
Citation: Xue H, Ma Y, Wang T, Gong H, Gao B, Fan X, Yan J, Meng X, Zhang S and He J (2019) Hollow Mesoporous Fe2O3 Nanospindles/CNTs Composite: An Efficient Catalyst for High-Performance Li-O2 Batteries. Front. Chem. 7:511. doi: 10.3389/fchem.2019.00511
Received: 16 May 2019; Accepted: 03 July 2019;
Published: 25 July 2019.
Edited by:
Jianqing Zhao, Soochow University, ChinaReviewed by:
Yuxin Tang, University of Macau, ChinaBo Jiang, National Institute for Materials Science, Japan
Jianhua Zhou, Guilin University of Electronic Technology, China
Copyright © 2019 Xue, Ma, Wang, Gong, Gao, Fan, Yan, Meng, Zhang and He. This is an open-access article distributed under the terms of the Creative Commons Attribution License (CC BY). The use, distribution or reproduction in other forums is permitted, provided the original author(s) and the copyright owner(s) are credited and that the original publication in this journal is cited, in accordance with accepted academic practice. No use, distribution or reproduction is permitted which does not comply with these terms.
*Correspondence: Tao Wang, wangtao0729@nuaa.edu.cn; Jianping He, jianph@nuaa.edu.cn