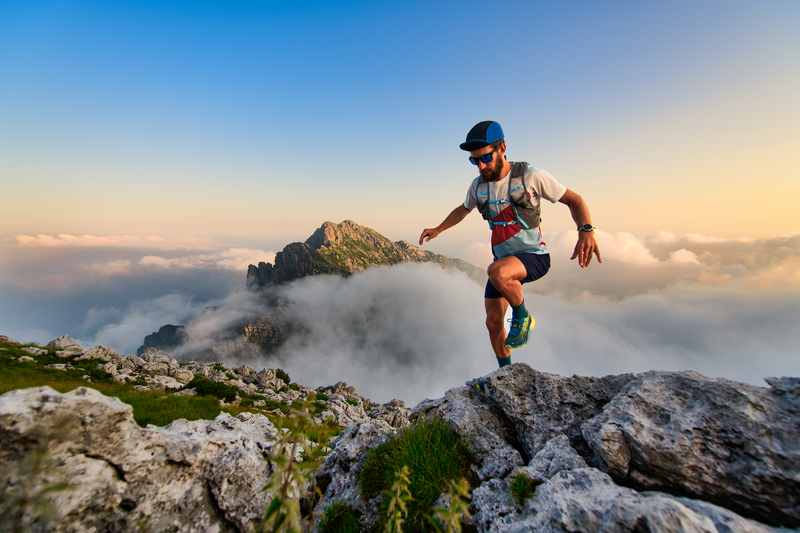
94% of researchers rate our articles as excellent or good
Learn more about the work of our research integrity team to safeguard the quality of each article we publish.
Find out more
REVIEW article
Front. Chem. , 10 July 2019
Sec. Nanoscience
Volume 7 - 2019 | https://doi.org/10.3389/fchem.2019.00495
This article is part of the Research Topic Multifunctional Bioactive Nanomaterials for Tissue Regeneration View all 7 articles
Periodontitis is considered to be the main cause of tooth loss, which affects about 15% of the adult population around the world. Scaling and root-planning are the conventional treatments utilized to remove the contaminated tissue and bacteria, but eventually lead to the formation of a poor connection—long junctional epithelium. Therefore, regenerative therapies, such as guided tissue/bone regeneration (GTR/GBR) for periodontal regeneration have been attempted. GTR membranes, acting as scaffolds, create three-dimensional (3D) environment for the guiding of cell attachment, proliferation and differentiation, and play a significant role in periodontal regeneration. Nano-composite scaffolds based on electrospun nanofibers have gained great attention due to their ability to emulate natural extracellular matrix (ECM) that affects cell survival, attachment and reorganization. Promoted protein absorption, cellular reactions, activation of specific gene expression and intracellular signaling, and high surface area to volume ratio are also important properties of nanofibrous scaffolds. Moreover, several bioactive components, such as bioceramics and functional polymers can be easily blended into nanofibrous matrixes to regulate the physical-chemical-biological properties and regeneration abilities. Simultaneously, functional growth factors, proteins and drugs are also incorporated to regulate cellular reactions and even modify the local inflammatory microenvironment, which benefit periodontal regeneration and functional restoration. Herein, the progress of nano-composite electrospun fibers for periodontal regeneration is reviewed, including fabrication methods, compound types and processes, and surface modifications, etc. Significant proof-of-concept examples are utilized to illustrate the results of material characteristics, cellular interactions and periodontal regenerations. Finally, the existing limitations of nano-composite electrospun fibers and the development tendencies in future are also discussed.
Periodontitis causes the progressive destruction of periodontal tissues, and affects about 15% of the adult population worldwide. The periodontium is composed of gingiva (gum), alveolar bone, periodontal ligament (PDL), and cementum (Figure 1) (Bottino et al., 2012; Sowmya et al., 2013). Cementum and alveolar bone are mineralized tissues, and they mainly surround and support the teeth. Gingiva and PDL are fibrous tissues, and PDL, also called Sharpey fiber, anchors the cementum of tooth root to the adjacent alveolar bone (Melcher, 1976). Periodontal disease can lead to loss of teeth. The worldwide prevalence of this disease has led to great demand for effective therapies. The ultimate ideal outcome is to reconstruct the original hierarchical complex architecture of periodontium, including new cementum, alveolar bone and PDL, which is called periodontal regeneration (Bosshardt and Sculean, 2009; Ripamonti and Petit, 2009). Although defected periodontium can be restored partially in clinics now, complete periodontal tissue regeneration has not been successfully realized in humans, for reasons like oral hygiene, defect size, infection and many others (Polimeni et al., 2006). The weak innate regeneration ability of periodontal tissues demonstrates the demand for clinical therapies for periodontium regeneration.
Figure 1. Compositions of the periodontium. Adapted with permission from Chen et al. (2010a). Copyright 2010 Elsevier.
Conventional treatment, open flap debridement (OFD), provides more access for scaling and root-planning, which can remove the bacteria and contaminated tissue from root surface, while leading to the formation of a poor connection—long junctional epithelium. If left empty after OFD, epithelial cells and fibroblasts will firstly fill in the defects, which prevents the sequential regeneration of true periodontal tissues (Chen et al., 2010a). Therefore, regenerative therapies, such as guided tissue/bone regeneration (GTR/GBR) for periodontal regeneration, have been attempted.
GTR utilizes a barrier membrane to prevent epithelial cells and fibroblasts from migrating into defected space, and maintain sufficient space and time for the regeneration of alveolar bone, cementum and PDL (Zhang et al., 2007; Park et al., 2009). The non-degradable membranes utilized in clinics mainly include polytetrafluoroethylene (PTFE) membrane like Cytoplast® TXT-200 and titanium-strengthened PTEE membrane like Cytoplast® Ti-250, and the main shortcoming lies in its inability to degrade, requiring a second operation (Gentile et al., 2011). To avoid additional surgical procedures, attempts have been made to develop degradable membranes, and the majority of the membranes on the market are based on synthetic polymers like poly-caprolactone (PCL), poly lactic acid (PLA), polyglycolic acid (PGA), and their copolymers, etc. (Coonts et al., 1998; Donos et al., 2002; Hou et al., 2004), and natural polymer like collagen (e.g., from porcine skin, Bio-Gide®) (Bunyaratavej and Wang, 2001), mainly fabricated by melting or solvent casting approaches. However, there are inevitable disadvantages in current GTR membranes, like low attachment to the adjacent tissues, lack of antibacterial properties, and poor ability to enhance tissue regeneration (Behring et al., 2008). In addition, existing biodegradable membranes are weak in appropriate mechanical properties and controllable degradation rate (Jung et al., 2013).
GTR can be conducted in combination with bone grafts to prevent membrane collapse (Figure 2) (Chen et al., 2010a). Currently in clinics, bone grafts including autografts, demineralized freeze-dried allografts and bovine derived xenografts have been used to restore alveolar bone defects. However, pre-existing curative effects indicate that the commercial grafts can just fill in the periodontal bone defects, and perform poorly in promoting hierarchical structure regeneration because of poor osteoinductivity (Yang et al., 2009; Reynolds et al., 2010).
Figure 2. Schematic illustration of GTR membrane combined with bone grafts therapy for periodontal regeneration. Adapted with permission from Chen et al. (2010a). Copyright 2010 Elsevier. (A) Periodontal defect with loss of PDL and alveolar bone. (B) Bone grafts in the defected site. (C) GTR/GBR membrane covered on the grafts. (D) Sewing for closure of the wound.
The ideal membrane has yet to be developed for enhancing periodontal regeneration. GTR/GBR membranes, acting as scaffolds to emulate the ECM, might recruit stem cells and progenitors from the retaining healthy adjacent alveolar bone, PDL and blood, and promote the proliferation and differentiation of these stem cells into fibroblasts, osteoblasts, and cementoblasts (Larsson et al., 2016). They are required to stay in place for at least 4–6 weeks with appropriate mechanical, biocompatible and degradable properties to prevent soft tissue growing into alveolar bone defects (Veríssimo et al., 2010), and induce bone regeneration for optimized periodontal regeneration (Kikuchi et al., 2004).
To obtain membranes with optimal properties, like biocompatibility, biodegradability, osteoconductivity, even osteoinductivity and ability to promote cell attachment, proliferation and differentiation for periodontal regeneration, electrospinning technology utilizing synthetic or/and natural polymers has received increasing attention (Bottino et al., 2012; Liu et al., 2018). Biocompatible electrospun nanofibers have an innate advantage in mimicking natural ECM, controllable degradation rate and excellent mechanic properties by regulating relative parameters. The small pore size of electrospun membranes can effectively inhibit migration of fibroblasts across the membrane barrier. To meet multiple requirements for periodontal regeneration, different polymers and various additives like active bioceramics, growth factors, proteins, and drugs can be incorporated into electrospun nanofibers to obtain ideal properties.
To update the advantage of electrospinning technology for periodontal regeneration, different components of various synthetic and natural polymers as matrixes and inorganic components as bioactive additives for periodontal regeneration are reviewed in this paper. Functional growth factors, proteins for optimized osteogenesis activity, and drugs like antibiotics for better regulation of inflammatory microenvironment are described. The existing limitations of nano-composite electrospun fibers and the future development trends are also discussed.
Electrospinning has gained more and more attention for the reason that it is widely recognized as a powerful tool for fabricating nanoscale materials with controllable fiber diameter, porosity, ideal morphology, and optimized surface characteristics (Lu et al., 2018). Electrospinning utilizes a polymeric solution or melt to generate nanofibers in high electrostatic field. Various components can be added to obtain properties, and random/aligned nanofibers, core-shell structure can be obtained by modulating electrospinning setups (Figure 3) (Min et al., 2015; Wu et al., 2018). A typical electrospinning setup requires four components: a syringe pump (containing solution/melt/suspension to be electrospun), a spinneret with a metallic needle (as a capillary), a high-voltage power supply (for generation of high electrical voltage) and a grounded conducting collector (static plate or rotatable drum) (Min et al., 2015). A proper high voltage makes liquid droplet formed by the polymer solution electrically charged, and the droplet is stretched with electrostatic forces counteracting the solution surface tension. A “Taylor cone” can be formed at the key point of voltage, which is called the threshold voltage, and then a jet of liquid erupts from the surface (Jiang et al., 2015a). A jet of polymer charged fluid is pulled toward the grounded collection, with multiple nanofibers deposited and solidified, during which the solvent evaporates, leaving dry nanofibers on the collector (Dersch et al., 2003; Subbiah et al., 2005).
Figure 3. Schematic illustration for electrospinning process: (A) Various additives incorporated into polymer matrixes. (B) Uniaxial electrospinning setup. Adapted with permission from Min et al. (2015). Copyright 2015 Elsevier. (C) Coaxial electrospinning setup. Adapted with permission from Chen et al. (2010b). Copyright 2010 Elsevier. (D) Random nanofibers, (E) Aligned nanofibers. Adapted with permission from Qasim et al. (2017), doi.org/10.1016/j.dental.2016.10.003, by the terms of the Creative Commons Attribution License (CC BY), http://creativecommons.org/licenses/by/4.0. Copyright 2017 Elsevier. (F) Core-shell structure. Adapted with permission from Tang et al. (2016). Copyright 2016 Elsevier.
Moreover, to be applied in various fields, basic equipment can be improved, like using multiple needle syringes to obtain blended fibers, or rotatable mandrel collectors to fabricate hollow tube-like fibers. Generally, nanofibers made through electrospinning are unwoven if using a static collector, while electrospinning with two strips of electrodes or using a rotatable collector has the ability to fabricate aligned fibers (Li et al., 2003). Apart from uniaxial electrospinning, core-shell structure can be fabricated by coaxial electrospinning for drug loading and functionally graded membrane (FGM) with multi-functional layers that can be obtained by sequential electrospinning. To get the ideal physical-chemical-biological properties of fibrous scaffolds, proper polymer matrixes, suitable additives, optimized spinning processes (temperature, humidity, customized accessories, etc.), and appropriate post-processing should be taken into serious considerations during the electrospinning process.
Nanofibrous scaffolds possess unique properties, including high surface area to volume ratio, controllable porosity with interconnected pores, enhanced protein absorption, activation of specific gene expression and intracellular signaling, and promoted cellular reactions (Zafar et al., 2016). With larger surface to absorb proteins, nanoscale scaffolds present more binding sites to cell receptors (Stevens, 2005). Several bioactive components, such as bioceramics and functional polymers can be blended into matrixes to enhance regeneration ability, and functional growth factors, proteins and drugs can be incorporated to regulate cellular reactions and/or modify the local inflammatory microenvironment.
Functional electrospun fibers can be obtained by blending various polymers together or incorporating functional components into the polymer matrixes. These composites are charming for reasons that these materials fabricated by electrospinning approach display good mechanical, bioactive and biological properties compared with the pure polymer matrixes. Various types of nano-components are summarized in Table 1.
Synthetic polymers have good mechanical properties and electrospinnability, but poor biological characteristics. It is a promising way to blend natural polymers with inherently good bioactive properties with synthetic polymers to promote cellular reactions in periodontal regeneration.
Polysaccharides, like chitosan, cellulose and alginate, etc., are attractive polymers in tissue engineering applications for their ideal biological properties and easy accessibility. Chitosan (CS) is a natural polymer, degraded into glycosylated collagen and chondroitin sulfate in vivo, which is widely studied for periodontal regeneration due to its excellent biological performance or, in other words, biocompatibility, biodegradability, and inherent antimicrobial properties (Eugene and Lee Yong, 2003; Lee et al., 2009). It was reported to improve osteogenic differentiation by means of enhancing mitogenic property of osteoblastic cells for bone regeneration (Peter et al., 2010; Anitha et al., 2014). Amine groups of chitosan offer a positive-charged surface, and chitosan is a relative hydrophilic material, thus, being able to promote protein adsorption and cell adhesion. But it lacks mechanical stability and solubility in water, and leads to the brittle nature of scaffolds. It can be blended with different synthetic polymers, like PLA (Shen et al., 2018), PCL (Masoudi et al., 2017), and bioceramics, etc. to make up for these shortcomings.
Cellulose, with good biocompatibility and biodegradability, is easily obtained from the natural world. Electrospun bacterial cellulose (BC)/hydroxyapatite (HA) nanofibers were prepared to promote osteogenic differentiation of stem cells (Ao et al., 2017). With ideal mechanical properties like high tensile strength and elastic modulus, BC is a promising material in GTR (Zhang et al., 2018). Alginate, similar in structure to glycosaminoglycan (GAG), the component of ECM, is one of most useful natural polymers in the biomedical field. Previous studies indicated that the addition of alginate could promote cell viability and osteogenic differentiation of stem cells (Hu and Yu, 2013;Ataie et al., 2019).
Structure proteins are vital components in ECM, and they are capable of enhancing the mechanical and biological properties of nanofibers, which make them promising GBR materials. Collagen (COL) shows good biological properties like high biocompatibility, good bio-affinity and resorbability, which can make up for the drawback of polyester in poor cell response, but it is insufficient in mechanical properties and dimensional stability (Liao et al., 2005). It degrades rapidly, and cannot shield the defected space efficiently because of its quick collapse (Bottino et al., 2011). Membranes based on collagen need to be further crosslinked, or blended with other polymers and additives like nHA (Wu et al., 2014) and bioactive glass (Zhou et al., 2017b) to overcome these drawbacks. Collagen was also electrospun on a chitosan basement to fabricate a bi-layered collagen/chitosan membrane for periodontal GBR (Lotfi et al., 2015).
In addition to collagen, there are many other proteins. Tussah silk fibroin (TSF), abundant in Arg-Gly-Asp motif and Asp, Ala, can be used to enhance cell adhesion. The addition of TSF can also improve mechanical properties like tensile strength (Shao et al., 2016). Zein, a native protein extracted from corn, has good biocompatibility and electrospinnability. However, the hydrophobicity of zein results in low cell affinity. It can be blended with the hydrophilic polymers, like gelatin, to overcome this disadvantage (Yang et al., 2017). He et al. (2017) constructed core-shell nanofibers utilizing zein as shell structure for its high hydrophobicity. Metronidazole was embedded in the core structure, and the hydrophobic polymer was used to prolong drug release.
Gelatin (GEL) has a structure similar to natural collagen, and possesses bio-signal groups which can enhance proliferation of various cells (Behring et al., 2008). It has been widely explored in tissue regeneration for its ideal biocompatibility and low immunogenicity. However, the high hydrophilicity of gelatin brings dissolubility in organic solution, thus it is attempted to be blended with various synthetic polymer like PCL (Xue et al., 2015; Kim et al., 2016; Ke et al., 2017), PLA (Bottino et al., 2011) and other natural polymers like zein (Yang et al., 2017) for enhanced solubility in spinning solvents and better electrospinnability.
For better electrospinnability and mechanical properties, synthetic polymers are widely utilized in electrospun GBR membranes. PCL is a biocompatible polyester with extraordinary mechanical properties, non-toxicity and ease of being electrospun into nanofibers (Shor et al., 2007). Despite the advantages described above, there are still some drawbacks. PCL increases hydrophobicity when the fiber diameter is electrospun into nanoscale, therefore it lacks in cell-recognizing sites, and leads to slower degradation rate and a lower expression of alkaline phosphatase (ALP) (Calvert et al., 2005). Chitosan blended with PCL provides a feasible strategy to overcome these disadvantages. The addition of hydrophilic chitosan into PCL matrix can lower its hydrophobic behavior and improve cell attachment. Furthermore, good miscibility doesn't constitute a requirement of any toxic crosslinking agents to crosslink them, unlike blends between PCL-gelatin and PCL-collagen (Shalumon et al., 2013; Nivedhitha et al., 2016; Masoudi et al., 2017).
PLA, as a biocompatible polyester, has been widely utilized in periodontitis treatment. Previous studies indicate that PLA can cause tissue inflammatory reaction due to its acidic degradation products. Negative effects can be exerted on the periodontal regeneration by acidic environment, and a relatively low pH value can lead to gingival inflammation (Bickel and Cimasoni, 1985; Patel et al., 2016). In addition, PLA is poor in hydrophilic property (Li et al., 2006). To overcome these disadvantages, Shen et al. (2018) embedded chitosan, the natural alkaline polymer, with PLA by electrospinning approach to improve its hydrophilicity and reduce acid products.
Co-polymers can take full advantages of both polymers, and they are extensively studied to explore materials with better properties. To improve the degradation rate of PLA, glycolic acid, which has analogous structure and faster degradation rate, can be incorporated into PLA chains to construct poly (lactide-co-glycolic acid) (PLGA), and to match repairing period of alveolar bone after periodontal regeneration therapy (6–12 months) (Park et al., 2009; Zhou et al., 2012). PLGA has the ability to regulate the degradation rate and improve the mechanical properties of PLA for bone regeneration (Lyu et al., 2013). However, PLGA has some disadvantages including weak hydrophilicity, cell adhesion, and acidic degradation products. Functional proteins (Campos et al., 2014), cellulose and multiwall carbon nanotubes (MWNTs) (Zhang et al., 2018) were added into PLGA nanofibers to improve cellular affinity, bioactivity, osteoconductivity and reduce aseptic inflammation. Additionally, it was reported that the incorporation of soluble eggshell membrane protein (SEP) could improve the electrospinnability and mechanical strength of PLGA (Jia et al., 2012). Poly (glycolic acid) (PGA) has good biocompatibility, but fast degradation rate by hydrolysis effect. While poly (butylene succinate) (PBS) degrades slower if compared with PGA, it has poor biological properties. Thus, the novel material poly (butylene succinate-co-glycolate) (PBSGL), based on PBS and PGA, was synthesized by Pajoumshariati et al. to take use to their advantage and make up for drawbacks (Pajoumshariati et al., 2018). Electrospun PBSGL membranes, with tunable hydrolytic rate, were proved to possess better mechanical and biological properties (Pajoumshariati et al., 2018).
In previous studies, multiple synthetic polymers have been utilized in electrospinning process for GBR application. Polyethylene oxide (PEO) could enhance viscosities of polymer solutions (Qasim et al., 2017), and was woven into nanofibers with proper morphologies (Hu and Yu, 2013). He et al. constructed naringin loaded polyvinylpyrrolidone (PVP) as a core fiber, which possessed good biocompatibility (He et al., 2018a). Poly (vinyl alcohol) (PVA) has good biodegradability and non-toxicity, and its films are uniform, thick, and foldable, which makes it effective in drug delivery system. Farooq et al. (2015) fabricated novel electrospun chitosan/HA/PVA membrane to load drug for GBR application.
Various synthetic and/or natural polymers can be blended and electrospun into nanofibers. Apart from different components affecting biological properties of nanofibers, alignment, topological, and mechanical cues might also have influence on the outcomes of alveolar bone and PDL regeneration. To achieve aligned PDL regeneration, the effect of the alignment form of nanofibers on cell bio-reactions has received increasing attention (Jose et al., 2009).
Jiang et al. (2015b) incorporated oriented biodegradable poly (caprolactone)-poly (ethylene glycol) (PCE) electrospun nanofibers mats into porous chitosan to realize the aligned PDL regeneration. The scaffolds were tailored into cross-sectioned slices, put against the exposed root surfaces, and then bovine-derived porous xenograft Bio-Oss was implanted to fill in the alveolar bone defect to immobilize the scaffolds. The rat periodontal defects regenerated PDL-like tissue arrangement after 2 months, and showed that aligned groups had more concentrated angles whose characters were closer to native PDL. Higher collagen I/collagen III ratio and more fibrous tissue formation were observed against random groups. These studies indicated that aligned nanofibers embedded scaffolds could enhance infiltration, viability, and expression of periostin of rat bone marrow mesenchymal stem cells (BMSCs), and led to more organized arrangement of regenerated PDL (Figure 4).
Figure 4. Process of aligned nanofibers embedded scaffold for organized PDL regeneration. Adapted with permission from Jiang et al. (2015b). Copyright 2015 Elsevier.
It is well-known that topological and mechanical cues play critical roles in the cell line of differentiation of periodontal ligament cells (PDLCs). PDLCs are exposed to mechanical pressure caused by occlusal forces. To study the effects of simultaneous topological and mechanical cues on the cell alignment and protein expressions of PDLCs, Kim et al. (2016) cultured PDLCs on aligned and random PCL/gelatin scaffolds, respectively under mechanical-stressed condition. The results showed that the cyclic uniaxial stretch and nanofiber alignment brought effects on differentiation orientation of PDLCs and led to higher expression of periostin, tenascin-C and TGF-β1 in aligned groups, which indicated the enhanced potential of PDLCs for ligamentogenesis with aligned fibers. But no unified conclusion on the effect of cyclic uniaxial stretch and nanofiber alignment on the reaction of PDLCs was reached, which needs to be further studied.
Random nanofibers are usually applied in alveolar regeneration, while aligned nanofibers perform better in organized PDL regeneration. To realize the simultaneous regeneration of both alveolar bone and aligned PDL, Qasim et al. (2017) fabricated a tri-layered membrane (Figure 5) consisting of random and aligned PEO-doped chitosan nanofibers, respectively. The aligned layer was designed for ligament and the random layer for alveolar bone. The histological results showed a large proportion of cell infiltration but a disorganized matrix deposition in random fibers group, while more organized deposited matrixes were observed in aligned fibers group. Therefore, a tri-layered membrane with different layer characteristics shows a possible way for simultaneous alveolar bone and aligned PDL regeneration.
Figure 5. Schematic diagram of a tri-layered membrane for simultaneous alveolar bone and aligned PDL regeneration. Adapted with permission from Qasim et al. (2017), doi.org/10.1016/j.dental.2016.10.003, by the terms of the Creative Commons Attribution License (CC BY), http://creativecommons.org/licenses/by/4.0. Copyright 2017 Elsevier.
The strategy of polymer combined with bioceramic components was inspired by the nature hybrid structure of bone matrix: a complex composite constructed by organic collagen fibers and hydroxyapatite (HAp). Bioceramics, such as HAp nanoparticles and bioactive glasses (BG) can be incorporated into the electrospun fiber matrixes to promote bioactivity and biological-physical-chemical properties, such as osteoconductivity, osteoinductivity, and to emulate the native inorganic bone components (Heinemann et al., 2010; Yeo et al., 2011; Qasim et al., 2015). For better alveolar bone regeneration in periodontium restoration, bioceramics can be incorporated into natural and/or synthetic polymeric scaffolds, and the composite scaffolds show enhanced osteoconductivity in comparison with the single-component polymeric scaffolds.
Hydroxyapatite (HAp) nanoparticle component. HAp, with molecular formula Ca10(PO4)6(OH)2, is considered as the most thermodynamically steady synthetic calcium phosphate ceramic. It is biocompatible, bioactive and osteoconductive (Yang et al., 2009). It is the major constituent of natural bone, and has been widely applied in the bone repairing field. However, it is impossible to use HAp alone as scaffold material due to its brittleness. A large amount of researches have proved that HAp incorporated into electrospun nanofibers can improve their mechanical properties, proliferation and mineralization of osteoblasts (Prabhakaran et al., 2009; Zhang et al., 2017).
The incorporation of HAp can effectively promote bone regeneration. Compared with COL/PCL electrospun nanofibers, the incorporation of HAp nanoparticles could promote the expression of bone-related markers of PDLCs, such as alkaline phosphatase (ALP) and osteocalcin (OCN), and it showed possible applications in GBR in periodontium restoration (Wu et al., 2014). The stimulation of nHA on the proliferation, differentiation and mineralization of human mesenchymal stem cells (hMSCs) was also observed in CS/silk fibroin (SF) based electrospun fiber membranes, and the nano-composite electrospun fibers with 30 wt.% nHA were ideal for GBR (Lai et al., 2015). Ao et al. (2017) added activated native cotton cellulose into the well-dispersed nHA suspensions and obtained aligned nanofibers utilizing the high-speed rotating collector. The result showed that the addition of nHA could remarkably enhance the mechanical property of the membrane, and promote cell proliferation. Incorporation of nHA might not lead to cell cytotoxicity or affect nanofiber alignment adversely.
The functionally graded membrane (FGM) was explored for tailoring different layer properties to fabricate a material system with ideal physical, chemical and mechanical properties to optimize periodontal regeneration (Chen et al., 2013). Bottino et al. (2011) carried out a sequential multilayer electrospinning process to design a FGM composed of a core layer and two surface layers, respectively interfaced with bone and epithelial tissues for GBR, which showed to be promising in solving the shortcomings of currently available membranes, like weak mechanical property and poor osteoinductivity. In this multilayer membrane, the core layer was composed of a poly(DL-lactide-co-ε-caprolactone) (PLCL) layer surrounded by two ternary PLCL/PLA/GEL blended layers to provide ideal mechanical properties. The surface layer designed to be interfaced with bone consisted of PLA/GEL and nHAp to mimic natural collagen-HAp bone matrix to promote bone regeneration, while the layer designed to be interfaced with epithelial tissues had the PLA/GEL as matrix, loaded with metronidazole (MNZ) to combat periodontal pathogens (Figure 6).
Figure 6. Schematic diagram of the FGM for periodontal regeneration. (A) Membrane used for GBR. (B) The core layer (CL) and the functional surface layers (SLs), respectively interfaced with bone (n-HAp) and epithelial tissues (MNZ). Adapted with permission from Bottino et al. (2011). Copyright 2011 Elsevier.
Ideal GTR membranes require various properties, like barrier ability, bone regeneration activity, and inflammatory microenvironment regulation, etc. To meet these requirements, different additives need to be incorporated into the nanofibers, but for a more effective release and function of these additives, they should not be blended simultaneously in one single spinning solution. Coaxial electrospinning technology is a promising strategy to fabricate core-shell structure, making it possible to load different additives in core and shell, respectively for different purposes for regeneration. Tang et al. (2016) fabricated PLGA/HA (core)-collagen/amoxicillin (shell) membrane by coaxial electrospinning for GTR. The core consisting of PLGA/HA was designed to prevent fibroblasts growing into defected space, and enhance bone regeneration, and at the same time the shell composed of collagen/amoxicillin was aimed to enhance wound healing process.
Beta tri-calcium phosphate (β-TCP) component. Beta tri-calcium phosphate (β-TCP) has been widely applied in bone repair and GBR membranes for its excellent osteoconductivity and ideal resorbability, which are vital properties for required bone regeneration (Yang et al., 2015). It can be blended into various polymer matrixes to promote physical and mechanical properties along with cell reactions. Masoudi et al. (2017) fabricated a two-layered membrane by electrospinning method in which one layer used poly (glycerol sebacate) (PGS)/PCL and β-TCP for GBR and another one containing PCL/PGS and chitosan functioned for GTR. In particular PGS, as an elastomeric polymer, can provide flexibility for the GTR/GBR membrane (Zaky et al., 2014). The results suggested that the membrane containing 10 wt.% β-TCP showed an overall better performance in contact angle, hydrophilicity, mechanical properties, proliferation and ALP activity of human fetal osteoblasts (hFOBs). Furthermore, incorporation of chitosan into PCL/PGS nanofibers could promote GTR barrier properties, including the reduction of fiber diameter, porosity and contact angle.
Bioactive glasses (BGs) are a typical group of Ca-Si based inorganic materials containing SiO2-CaO-P2O5 networks. The Ca-Si based materials with special components possess excellent osteoconductivity and even osteoinductivity (Murakami et al., 2017). BGs have been developed into various kinds of glasses and glass-ceramics. They perform well in small bone defect repair and are able to provide appropriate environment for the cultured cells to grow naturally like HAp. Its dissolution products have the ability to enhance the cell proliferation and activate the gene expression of osteoblasts (Xia et al., 2007). Therefore, BGs have been widely selected as bioactive components to be blended into various biodegradable electrospun polymers for bone tissue engineering applications, such as PCL/chitosan (Shalumon et al., 2013), PCL/gelatin (El-Fiqi et al., 2015) and PVA (Shankhwar et al., 2016), etc.
A biomimetic fish Col/BG/CS nano-composite electrospun nanofiber membrane was developed by Zhou et al. (2017b). The results revealed that the incorporation of BG promoted cell viability and gene expression of RUNX-2, ALP, OPN and OCN of PDLCs. Moreover, the in vivo results using the periodontal defect model in beagle dogs showed that much more new bone formed and less inflammation presented in the Col/BG/CS membrane group when compared with the Col/CS control group.
Compared with the traditional BG, the mesoporous bioactive glass nanoparticles (mBGn) possessed excellent ability for bone tissue regeneration due to prominent bone regeneration activity. The release of Ca and Si ions from mBGn could enhance the differentiation of stem cells or progenitors into osteoblasts (El-Fiqi et al., 2013). El-Fiqi et al. (2015) incorporated mBGn loaded with osteogenic drug Dexamethasone (DEX) into PCL/gelatin matrix by electrospinning method. Compared to the pure biopolymer matrix, the addition of mBGn enhanced the mechanical tensile strength, elasticity, and hydrophilicity. Moreover, the sustainable release of DEX further accelerated the proliferation and osteogenic differentiation of PDLCs.
HA and BG are both widely used for bone regeneration, but great attention has been devoted to determining which one performs better. Shalumon et al. (2013) compared the electrospun composite nanofiber membranes of nHA/PCL/CS (PCH) and nBG/PCL/CS (PCB) with the same amount (3 wt.%) of nHA and nBG. The results showed that both nHA and nBG could apparently enhance the ALP activity of human periodontal ligament fibroblast cells (hPDLFs), while the incorporation of nBG showed superior performance in the adhesion and proliferation of hPDLFs and osteoblast like cells (MG-63 cell lines). A similar phenomenon was also observed by Sunandhakumari et al. (2018). These studies indicate that, compared to nHA, mBG performs better in enhancing viability, attachment, proliferation and differentiation of osteogenic-relative cells.
Periodontitis is an infective disease, in which bacteria release toxins, and the periodontium is then destructed. The main treatment strategy in clinics is topical antibiotics therapy, but the undesirable effects of antibiotics severely inhibit the curative effects. Therefore, it is important to control the release of antibiotics to regulate the inflammatory environment in periodontal regeneration. Incorporating antibacterial materials into electrospun nanofibers is a promising way due to controllable antibacterial function and enhanced bone regeneration activity at the same time. ZnO particles have potentials to introduce antibacterial activity and improve the osteoconductivity (Augustine et al., 2014; Münchow et al., 2015a). Nasajpour et al. (2018) incorporated ZnO into PCL by electrospinning method with ZnO concentrations of 0, 0.5, and 1 wt.%, respectively. In vitro periodontal ligament stem cells (PDLSCs) culture results indicated that PCL blended with 0.5 wt.% ZnO showed the optimal properties of cell viability, mineralization ability, and bone-related gene expression of Runx2, OCN, and ALP. Furthermore, in vitro Porphyromonas gingivalis culture proved that ZnO incorporated PCL membrane possessed excellent antibacterial activity. In conclusion, this novel membrane not only has antibacterial activity, but also has the ability to improve the osteoconductivity, which can makes it a promising candidate to regulate inflammatory environment and promote bone regeneration in periodontal regeneration.
Münchow et al. (2015b) incorporated CaO into PCL by electrospinning with different concentrations of CaO at 0, 5, 10, and 15 wt.%, respectively. The mechanical properties reduced progressively with the increase of CaO concentrations. The CaO-loaded membranes did not provide consistent antibacterial activity, while they increased the viability and osteogenic differentiation of MC3T3-E1. It is important to decide the appropriate type and concentration of oxide added into nanofibers during the electrospinning process to guarantee the biocompatibility of the implanted material.
Multiwall carbon nanotubes (MWNTs) have received great attention for their excellent mechanical properties, biocompatibility and stability for tissue engineering. Zhang et al. (2018) used bacterial cellulose (BC) membrane as lower membrane to collect electrospun PLGA/MWNT nanofibers to fabricate a bi-layered composite membrane, constructed by long, continuous fibers and weaved into a 3D network structure. The addition of MWNT remarkably improved the strength and toughness of the PLGA nanofiber scaffolds. Histological results of periodontal defect model tests showed that in PLGA/MWNT/BC composite membrane group, more newly formed PDL was found adjacent to new alveolar bone, and more collagen fiber bundles imbedded in the cementum and much more newly formed alveolar bone could be observed than in control group, indicating a greater ability to promote periodontal regeneration using MWNTs as additives.
It is also a strategy to blend MWNTs with HA to bring higher bioactivity. Mei et al. (2007) designed a PLA/MWNTs/HA composite GTR membrane in which the MWNTs and HA nanoparticles uniformly dispersed among the membrane. Comparing with the single PLA group, the addition of MWNTs and HA improved degradation property, and accelerated the adhesion and proliferation of PDLCs, at the same time the ingrowth of gingival epithelial cells was inhibited.
Graphene oxide (GO), derived from graphene, has received great attention in bone regeneration for its prominent mechanical strength, antibacterial property and capability of promoting osteogenic differentiation (Luo et al., 2015). Zhou et al. (2017a) fabricated electrospun P34HB/GO nanofibers, and the results indicated that GO could enhance tensile strength and Young's modulus of the membrane, and promote bone regeneration in vivo.
Various metal nanoparticles with different characteristics can be incorporated into nanofibers to improve membrane properties like antibacterial activity and bone regeneration activity, etc. Silver nanoparticles (AgNPs), which possess excellent antibacterial properties, show 1.4- to 1.9-times stronger antibacterial properties compared with silver ions (Ingle et al., 2008). Moreover, AgNPs have a much lower tendency to induce bacterial resistance compared with classical antibiotics (Sondi and Salopeksondi, 2004). Shao et al. (2017) fabricated a chitosan-based membrane blended with AgNPs. The results of antibacterial property evaluation revealed that AgNPs could have sustained antibacterial properties against Porphyromonas gingivalis and Fusobacterium nucleatum in a dose-dependent manner. Furthermore, appropriate amounts of AgNPs added into chitosan-based membranes did not cause noticeable cytotoxic effects on PDLCs, and the incorporation of AgNPs did not exert adverse influences on soft tissue responses. However, the safety intake amount of the AgNPs should be taken into consideration seriously.
Gold nanoparticles (AuNPs) were proved to have the potential to promote the differentiation of hPDLSCs into osteoblasts, increase their osteogenic-related expression of ALP, OCN, COL1, and RUNX2 via MAPK signaling pathway (Niu et al., 2017; Jadhav et al., 2018), while AuNPs incorporated electrospun fiber scaffolds still require more exploration in bone regeneration.
In the treatment for periodontitis, it is a feasible approach to incorporate multiple drugs into nanofibers to realize periodontal regeneration and anti-inflammation simultaneously. Anti-inflammatory agents can activate signaling cascades and trigger the healing process. Additionally, drugs loaded electrospun nanofibers can bring in remarkable characteristics, such as high loading capacity, high surface area to volume ratio and easy modulation of drug release profile.
Antibiotics, among the most useful drugs in clinics, are increasingly utilized in periodontal regeneration. Clinicians view metronidazole (MNZ) as the gold standard for the treatment of anaerobic infection, which is the main infection form of periodontitis. Reise et al. (2012) fabricated MNZ loaded electrospun PLA membrane, and found that this membrane could significantly inhibit the viability of periodontopathogenic species F. nucleatum and P. gingivalis for up to 2 days. Sequential electrospinning to fabricate FGM (Bottino et al., 2011) and coaxial electrospinning to construct core-shell structure (Tang et al., 2016) were utilized to incorporate MNZ into nanofibers for better drug delivery. Additionally, multiple drugs were added into nanofibers in attempts to assist periodontal regeneration, including ampicillin (Schkarpetkin et al., 2016), amoxicillin (Furtos et al., 2017), tetracycline hydrochloride (Ranjbar-Mohammadi et al., 2016), doxycycline hydrochloride (Debridement, 2016), and tinidazole (Khan et al., 2017), etc.
One of the purposes of periodontal treatment is to inhibit inflammatory reaction. Sustained release of prostaglandin (PG) during periodontal wound healing process exerts adverse effects on periodontal regeneration. Non-steroidal anti-inflammatory drugs (NSAIDs), like ibuprofen (Batool et al., 2018) and piroxicam (Farooq et al., 2015), can inhibit the activity of cyclooxygenase (COX), thus blocking arachidonic acid (AA) being converted into PG.
Growth factors can be used to promote osteogenesis of alveolar bone. Bone morphogenetic proteins (BMPs) were widely reported to enhance bone reconstruction in GBR (Shalumon and Jyh-Ping, 2015). Ho et al. (2017) incorporated platelet-derived growth factor (PDGF), a potent mitogen, into the nanofibers to facilitate alveolar ridge regeneration.
Functional proteins are also promising candidates to improve anti-bacterial and biological properties of materials. Antimicrobial peptides (AMPs) have a broad spectrum of antibacterial activity, distinguished from conventional antibiotics, which may result in bacterial resistance. He et al. (2018b) incorporated AMP-loaded PLGA microspheres into electrospun chitosan/gelatin nanofibers, which maintained the continuing release of Pac-525 to promote anti-bacterial activity. Fibronectin (FN) is a ligand–integrin affinity protein that can be found in ECM and cell membranes. It can aggregate adjacent cells by Arg-Gly-Asp (RGD) motif, and create binding sites to promote cell recognition. Campos et al. (2014) deposited FN onto hydrolyzed PLGA nanofibers to promote the bioactivity of scaffolds.
Periodontitis is a chronic infection disease, which requires effective treatments for clinical applications. Conventional OFD can result in only weak epithelium connection, without affecting native periodontium structure. GTR is a promising method to promote the complex reconstruction of periodontium. Existing commercial GTR membranes still have no desirable curative effects, and have innate disadvantages, like poor mechanical property, inappropriate degradation rate and weak ability in promoting hierarchical periodontium regeneration, etc. Novel GTR membranes are required to possess three main properties to meet clinic requirements:
(1) Proper degradability, mechanical properties and biocompatibility;
(2) Optimized alveolar bone and organized PDL regeneration activity;
(3) Antibacterial activity.
Electrospinning technology is prominently efficient in the fabrication of GTR membranes. Different polymers and various additives, soluble in relative solvents, can be subsequently electrospun into composite nanofibers. Electrospun nanofibers possess innate advantages in promoting periodontal regeneration, including high surface area to volume ratio for higher protein absorption ability, activation of specific gene expression and intracellular signaling, as well as enhanced cellular interactions. Furthermore, the small pore size of the electrospinning nanofiber network can effectively inhibit the migration of fibroblasts across the barrier, which is vital in GTR/GBR therapies for periodontal regeneration. To obtain dual additives incorporated nanofibers with ideal releasing efficiency and active function, FGM can be fabricated by sequential electrospinning and core-shell structure can be obtained using coaxial electrospinning process.
Appropriate biopolymers and additives should be selected to guarantee their excellent biocompatibility. And biopolymers and additives in proper proportions make it possible to regulate the degradation rate of the membranes. To maintain the membranes in the implanted site for at least 4–6 weeks and avoid collapsing too early, mechanic properties (like flexibility) need to be enhanced. Natural polymers (like chitosan, cellulose, alginate, gelatin, collagen, silk fibroin, zein), with good biological properties and synthetic polymers (like PCL, PLA, PLGA, PBSGL), with good mechanical properties and electrospinnability can be blended to make full use of both advantages.
Capability to promote periodontal regeneration is one of the vital properties required for GTR membranes. Electrospun nanofibers, with inherent ability to mimic natural ECM, perform excellently in inducing osteo-differentiation. Inorganic ceramics like nHA, β-TCP, BGs, ZnO, AuNPs and carbon-based MWNTs are proposed to facilitate the bone regeneration process, and it is possible to enhance mechanical properties at the same time. Furthermore, the functional biomolecules like proteins and growth factors are also utilized to promote regenerative properties, emulating natural in vivo osteo-differentiation stimulus. Therefore, aligned electrospun nanofibers can be a promising alternative in organized PDL regeneration.
Infection is regarded as the main factor inducing GTR failure in clinics. Adding multiple drugs facilitates periodontal regeneration and improves the anti-inflammatory microenvironment simultaneously. Till now, various drugs like antibiotics (amoxicillin, ampicillin, metronidazole, tinidazole, doxycycline hydrochloride and tetracycline hydrochloride and combination of amoxicillin-metronidazole-lidocaine, etc.) and NSAIDs, functional proteins like AMPs, oxide components like ZnO and metal nanoparticles like AgNPs, have been incorporated into electrospun nanofibers to inhibit bacterial growing and create ideal anti-inflammatory environment.
Regenerating complex hierarchical structure is vital in periodontal regeneration. Not only alveolar bone should be reconstructed, but also the structure of aligned Sharpey fibers anchoring root cementum to adjacent alveolar bone, which is challenging in hierarchical periodontium regeneration. Although electrospinning technology might be a promising way in tissue regeneration, there are still some problems to be further explored.
Electrospinning technology has distinct advantages in periodontal regeneration. Parameters can be regulated to control relative properties of electrospun fibers, like fiber diameter, porosity and pore size, etc. Various materials including functional polymers and bioceramics, with their respective advantages and disadvantages in physical-chemical characteristics, can be utilized for fabrication. Therefore, it is vital to explore ideal materials in appropriate proportions and optimized parameters for electrospinning process, depending on clinical requirements. Pore size can be controlled by regulating relative parameters during electrospinning process. Relatively big pore size is beneficial for cell infiltration but unfavorable for membrane barrier, while relatively small size leads to opposite characteristic. It is required to strike a balance in pore size, or acquire a multi-layered membrane, one layer with small pore size for membrane barrier and another one with relatively big core size for better cell infiltration.
Electrospun nanofiber membranes usually have a dense inner packing structure, and pore sizes are too small for cells to infiltrate into the mats. Although some methods like salt leaching can improve cell infiltration in electrospun mats, the results are not desirable. Therefore, electrospun mats are widely applied in the GTR field, but not as scaffolds implanted into defected sites. Additionally, vascularization is one of the vital factors in tissue regeneration, because this process supplies essential oxygen and nutrients for cells to proliferate and differentiate. Taken together, the next step is to evaluate the bone regeneration and vascularization in vivo.
To maintain the bioactivity and control the releasing profile of drugs polymer-related parameters including component, crystallinity and molecular weight of polymer, and drug-related parameters including molecular weight, drug loading, and crystallinity of drug should be taken into consideration. With the increasingly wide utilization of antibiotics in clinics, bacterial resistance has become a great challenge in bacterial inhibition, thus it is desirable to explore more types of drugs to reduce bacterial resistance, and control periodontal inflammation efficiently. Growth factors degrade quickly in vivo, which severely limits their clinical efficiency. Release of the macromolecules based on electrospun nanofibers is not desirable. To construct effective vehicles for controllable release of growth factors, and even realize the temporally distinct release in target period during the regeneration process, electrospinning technology can be combined with other approaches.
Apart from scaffolds, stem cells like PDLCs also play important roles in periodontal tissue engineering. Absence in adequate healthy stem cells is one of the reasons contributing to the incapability of instinctive periodontal regeneration. Stem cells can be cultured on scaffold in vitro and then implanted in defected sites to provide sufficient progenitors for tissue regeneration by tissue engineering technology. But the origin of stem cells, the in vitro culture condition, the viability of stem cells and clinical effects are challenging for their clinical applications. The next step is to select proper growth factors and stem cells, explore appropriate proportions in scaffolds and improve viability of these stem cells for optimal regeneration of periodontium.
In conclusion, the electrospinning technology has received great attention in periodontal regeneration. Although there still exist some disadvantages and shortcomings to overcome, it is believed that electrospun nanofibers will be further explored and widely applied in clinics for their innate advantages.
YZ wrote the manuscript. KL and HY conceived the concept of this review. All authors discussed and commented on the manuscript.
The authors declare that the research was conducted in the absence of any commercial or financial relationships that could be construed as a potential conflict of interest.
The authors gratefully acknowledge the support of the National Natural Science Foundation of China (Nos. 81571022, 81871490, 81672134), Science and Technology Commission of Shanghai Municipality (Nos. 16DZ0503800, 17410710500), Three Year Action Plan to Promote Clinical Skills and Clinical Creativity in Municipal Hospitals (No. 16CR10308B), Pudong New Area Commission of Health and Family Planning (No. PW2016E-1), Program of Shanghai Academic/Technology Research Leader (No. 19XD1434500).
Anitha, A., Sowmya, S., Kumar, P. T. S., Deepthi, S., Chennazhi, K. P., Ehrlich, H., et al. (2014). Chitin and chitosan in selected biomedical applications. Prog. Polym. Sci. 39, 1644–1667. doi: 10.1016/j.progpolymsci.2014.02.008
Ao, C. H., Niu, Y., Zhang, X. M., He, X., Zhang, W., and Lu, C. H. (2017). Fabrication and characterization of electrospun cellulose/nano-hydroxyapatite nanofibers for bone tissue engineering. Int. J. Biol. Macromol. 97, 568–573. doi: 10.1016/j.ijbiomac.2016.12.091
Ataie, M., Shabani, I., and Seyedjafari, E. (2019). Surface mineralized hybrid nanofibrous scaffolds based on poly(l-lactide) and alginate enhances osteogenic differentiation of stem cells. J. Biomed. Mater. Res. 107, 586–596. doi: 10.1002/jbm.a.36574
Augustine, R., Malik, H. N., Singhal, D. K., Mukherjee, A., Malakar, D., Kalarikkal, N., et al. (2014). Electrospun polycaprolactone/ZnO nanocomposite membranes as biomaterials with antibacterial and cell adhesion properties. J. Polym. Res. 21, 1–17. doi: 10.1007/s10965-013-0347-6
Batool, F., Morand, D.-N., Thomas, L., Bugueno, I. M., Aragon, J., Irusta, S., et al. (2018). Synthesis of a novel electrospun polycaprolactone scaffold functionalized with ibuprofen for periodontal regeneration: an in vitro and in vivo study. Materials 11:E580. doi: 10.3390/ma11040580
Behring, J., Junker, R., Walboomers, X. F., Chessnut, B., and Jansen, J. A. (2008). Toward guided tissue and bone regeneration: morphology, attachment, proliferation, and migration of cells cultured on collagen barrier membranes. A systematic review. Odontology 96, 1–11. doi: 10.1007/s10266-008-0087-y
Bickel, M., and Cimasoni, G. (1985). The pH of human crevicular fluid measured by a new microanalytical technique. J. Periodont. Res. 20, 35–40. doi: 10.1111/j.1600-0765.1985.tb00408.x
Bosshardt, D. D., and Sculean, A. (2009). Does periodontal tissue regeneration really work? Periodontol. 2000 51, 208–219. doi: 10.1111/j.1600-0757.2009.00317.x
Bottino, M. C., Thomas, V., and Janowski, G. M. (2011). A novel spatially designed and functionally graded electrospun membrane for periodontal regeneration. Acta Biomater. 7, 216–224. doi: 10.1016/j.actbio.2010.08.019
Bottino, M. C., Thomas, V., Schmidt, G., Vohra, Y. K., Chu, T.-M. G., Kowolik, M. J., et al. (2012). Recent advances in the development of GTR/GBR membranes for periodontal regeneration—a materials perspective. Dent. Mater. 28, 703–721. doi: 10.1016/j.dental.2012.04.022
Bunyaratavej, P., and Wang, H. L. (2001). Collagen membranes: a review. J. Periodontol. 72, 215–229. doi: 10.1902/jop.2001.72.2.215
Calvert, J. W., Chua, W. C., Gharibjanian, N. A., Dhar, S., and Evans, G. R. D. (2005). Osteoblastic phenotype expression of MC3T3-E1 cells cultured on polymer surfaces. Plast. Reconstr. Surg. 116, 567–576. doi: 10.1097/01.prs.0000172986.68749.2d
Campos, D. M., Gritsch, K., Salles, V., Attik, G. N., and Grosgogeat, B. (2014). Surface entrapment of fibronectin on electrospun PLGA scaffolds for periodontal tissue engineering. Biores. Open Access 3, 117–126. doi: 10.1089/biores.2014.0015
Chen, D. W.-C., Lee, F.-Y., Liao, J.-Y., Liu, S.-J., Hsiao, C.-Y., and Chen, J.-K. (2013). Preclinical experiments on the release behavior of biodegradable nanofibrous multipharmaceutical membranes in a model of four-wall intrabony defect. Antimicrob. Agents Chemother. 57, 9–14. doi: 10.1128/AAC.00506-12
Chen, F. M., Zhang, J., Zhang, M., An, Y., Chen, F., and Wu, Z. F. (2010a). A review on endogenous regenerative technology in periodontal regenerative medicine. Biomaterials 31, 7892–7927. doi: 10.1016/j.biomaterials.2010.07.019
Chen, R., Huang, C., Ke, Q., He, C., Wang, H., and Mo, X. (2010b). Preparation and characterization of coaxial electrospun thermoplastic polyurethane/collagen compound nanofibers for tissue engineering applications. Colloids Surf. B Biointerfaces 79, 315–325. doi: 10.1016/j.colsurfb.2010.03.043
Coonts, B. A., Whitman, S. L., O'Donnell, M., Poison, A. M., Bogle, G., Garrett, S., et al. (1998). Biodegradation and biocompatibility of a guided tissue regeneration barrier membrane formed from a liquid polymer material. J. Biomed. Mater. Res. 42, 303–311. doi: 10.1002/(SICI)1097-4636(199811)42:2<303::AID-JBM16>3.0.CO;2-J
Debridement, A. S. (2016). Development of a doxycycline hydrochloride-loaded electrospun nanofibrous membrane for GTR/GBR applications. J. Nanomater. 2016, 1–10. doi: 10.1155/2016/6507459
Dersch, R., Liu, T., Schaper, A. K., Greiner, A., and Wendorff, J. H. (2003). Electrospun nanofibers: internal structure and intrinsic orientation. J. Polym. Sci. A Polym. Chem. 41, 545–553. doi: 10.1002/pola.10609
Donos, N., Kostopoulos, L., and Karring, T. (2002). Alveolar ridge augmentation using a resorbable copolymer membrane and autogenous bone grafts: an experimental study in the rat. Clin. Oral Implants Res. 13, 203–213. doi: 10.1034/j.1600-0501.2002.130211.x
El-Fiqi, A., Kim, J. H., and Kim, H. W. (2015). Osteoinductive fibrous scaffolds of biopolymer/mesoporous bioactive glass nanocarriers with excellent bioactivity and long-term delivery of osteogenic drug. ACS Appl. Mater. Interfaces 7, 1140–1152. doi: 10.1021/am5077759
El-Fiqi, A., Lee, J. H., Lee, E.-J., and Kim, H.-W. (2013). Collagen hydrogels incorporated with surface-aminated mesoporous nanobioactive glass: improvement of physicochemical stability and mechanical properties is effective for hard tissue engineering. Acta Biomater. 9, 9508–9521. doi: 10.1016/j.actbio.2013.07.036
Eugene, K., and Lee Yong, L. (2003). Implantable applications of chitin and chitosan. Biomaterials 24, 2339–2349. doi: 10.1016/S0142-9612(03)00026-7
Farooq, A., Yar, M., Khan, A. S., Shahzadi, L., Siddiqi, S. A., Mahmood, N., et al. (2015). Synthesis of piroxicam loaded novel electrospun biodegradable nanocomposite scaffolds for periodontal regeneration. Mater. Sci. Eng. C Mater. Biol. Appl. 56, 104–113. doi: 10.1016/j.msec.2015.06.006
Furtos, G., Rivero, G., Rapuntean, S., and Abraham, G. A. (2017). Amoxicillin-loaded electrospun nanocomposite membranes for dental applications. J. Biomed. Mater. Res. B Appl. Biomater. 105, 966–976. doi: 10.1002/jbm.b.33629
Gentile, P., Chiono, V., Tonda-Turo, C., Ferreira, A. M., and Ciardelli, G. (2011). Polymeric membranes for guided bone regeneration. Biotechnol. J. 6, 1187–1197. doi: 10.1002/biot.201100294
He, M., Jiang, H., Wang, R., Xie, Y., and Zhao, C. (2017). Fabrication of metronidazole loaded poly (ε-caprolactone)/zein core/shell nanofiber membranes via coaxial electrospinning for guided tissue regeneration. J. Colloid Interface Sci. 490, 270–278. doi: 10.1016/j.jcis.2016.11.062
He, P., Zhong, Q., Ge, Y., Guo, Z., Tian, J., Zhou, Y., et al. (2018a). Dual drug loaded coaxial electrospun PLGA/PVP fiber for guided tissue regeneration under control of infection. Mater. Sci. Eng. C Mater. Biol. Appl. 90:549. doi: 10.1016/j.msec.2018.04.014
He, Y. Z., Jin, Y. H., Wang, X. M., Yao, S. L., Li, Y. Y., Wu, Q., et al. (2018b). An antimicrobial peptide-loaded gelatin/chitosan nanofibrous membrane fabricated by sequential layer-by-layer electrospinning and electrospraying techniques. Nanomaterials 8:13. doi: 10.3390/nano8050327
Heinemann, C., Heinemann, S., Bernhardt, A., Lode, A., Worch, H., and Hanke, T. (2010). In vitro osteoclastogenesis on textile chitosan scaffolds. Eur. Cell. Mater. 19, 96–106. doi: 10.22203/eCM.v019a10
Ho, M.-H., Chang, H.-C., Chang, Y.-C., Claudia, J., Lin, T.-C., and Chang, P.-C. (2017). PDGF-metronidazole-encapsulated nanofibrous functional layers on collagen membrane promote alveolar ridge regeneration. Int. J. Nanomedicine 12, 5525–5535. doi: 10.2147/IJN.S137342
Hou, L. T., Yan, J. J., Tsai, A. Y. M., Lao, C. S., Lin, S. J., and Liu, C. M. (2004). Polymer-assisted regeneration therapy with Atrisorb® barriers in human periodontal intrabony defects. J. Clin. Periodontol. 31, 68–74. doi: 10.1111/j.0303-6979.2004.00436.x
Hu, W. W., and Yu, H. N. (2013). Coelectrospinning of chitosan/alginate fibers by dual-jet system for modulating material surfaces. Carbohydr. Polym. 95, 716–727. doi: 10.1016/j.carbpol.2013.02.083
Ingle, A. P., Gade, A. K., Pierrat, S., Soennichsen, C., and Rai, M. K. (2008). Mycosynthesis of silver nanoparticles using the fungus fusarium acuminatum and its activity against some human pathogenic bacteria. Curr. Nanosci. 4, 141–144. doi: 10.2174/157341308784340804
Jadhav, K., Hr, R., Deshpande, S., Jagwani, S., Dhamecha, D., Jalalpure, S., et al. (2018). Phytosynthesis of gold nanoparticles: characterization, biocompatibility, and evaluation of its osteoinductive potential for application in implant dentistry. Mater. Sci. Eng. C 93, 664–670. doi: 10.1016/j.msec.2018.08.028
Jia, J., Liu, G., Yu, J., and Duan, Y. (2012). Preparation and characterization of soluble eggshell membrane protein/PLGA electrospun nanofibers for guided tissue regeneration membrane. J. Nanomater. 2012:25. doi: 10.1155/2012/282736
Jiang, S., Liu, F., Lerch, A., Ionov, L., and Agarwal, S. (2015a). Unusual and superfast temperature-triggered actuators. Adv. Mater. 27, 4865–4870. doi: 10.1002/adma.201502133
Jiang, W., Li, L., Zhang, D., Huang, S., Jing, Z., Wu, Y., et al. (2015b). Incorporation of aligned PCL-PEG nanofibers into porous chitosan scaffolds improved the orientation of collagen fibers in regenerated periodontium. Acta Biomater. 25, 240–252. doi: 10.1016/j.actbio.2015.07.023
Jose, M. V., Vinoy, T., Johnson, K. T., Dean, D. R., and Elijah, N. (2009). Aligned PLGA/HA nanofibrous nanocomposite scaffolds for bone tissue engineering. Acta Biomater. 5, 305–315. doi: 10.1016/j.actbio.2008.07.019
Jung, R. E., Fenner, N., Hämmerle, C. H., and Zitzmann, N. U. (2013). Long-term outcome of implants placed with guided bone regeneration (GBR) using resorbable and non-resorbable membranes after 12–14 years. Clin. Oral Implants Res. 24, 1065–1073. doi: 10.1111/j.1600-0501.2012.02522.x
Ke, R., Yi, W., Tao, S., Wen, Y., and Zhang, H. (2017). Electrospun PCL/gelatin composite nanofiber structures for effective guided bone regeneration membranes. Mater. Sci. Eng. C 78:324. doi: 10.1016/j.msec.2017.04.084
Khan, G., Yadav, S. K., Patel, R. R., Kumar, N., Bansal, M., and Mishra, B. (2017). Tinidazole functionalized homogeneous electrospun chitosan/poly (ε-caprolactone) hybrid nanofiber membrane: development optimization and its clinical implications. Int. J. Biol. Macromol. 103:1311. doi: 10.1016/j.ijbiomac.2017.05.161
Kikuchi, M., Koyama, Y., Yamada, T., Imamura, Y., Okada, T., Shirahama, N., et al. (2004). Development of guided bone regeneration membrane composed of β-tricalcium phosphate and poly (l-lactide-co-glycolide-co-ε-caprolactone) composites. Biomaterials 25, 5979–5986. doi: 10.1016/j.biomaterials.2004.02.001
Kim, J. H., Min, S. K., Eltohamy, M., Kim, T. H., and Kim, H. W. (2016). Dynamic mechanical and nanofibrous topological combinatory cues designed for periodontal ligament engineering. PLoS ONE 11:e0149967. doi: 10.1371/journal.pone.0149967
Lai, G. J., Shalumon, K. T., and Chen, J. P. (2015). Response of human mesenchymal stem cells to intrafibrillar nanohydroxyapatite content and extrafibrillar nanohydroxyapatite in biomimetic chitosan/silk fibroin/nanohydroxyapatite nanofibrous membrane scaffolds. Int. J. Nanomedicine 10, 567–584. doi: 10.2147/IJN.S73780
Larsson, L., Decker, A. M., Nibali, L., Pilipchuk, S. P., Berglundh, T., and Giannobile, W. V. (2016). Regenerative medicine for periodontal and peri-implant diseases. J. Dent. Res. 95:255. doi: 10.1177/0022034515618887
Lee, K. Y., Jeong, L., Kang, Y. O., Lee, S. J., and Park, W. H. (2009). Electrospinning of polysaccharides for regenerative medicine. Adv. Drug Deliv. Rev. 61, 1020–1032. doi: 10.1016/j.addr.2009.07.006
Li, D., Wang, Y., and Xia, Y. (2003). Electrospinning of polymeric and ceramic nanofibers as uniaxially aligned arrays. Nano Lett. 3, 1167–1171. doi: 10.1021/nl0344256
Li, W. J. Jr., C., J., Mauck, R. L., and Tuan, R.S. (2006). Fabrication and characterization of six electrospun poly(alpha-hydroxy ester)-based fibrous scaffolds for tissue engineering applications. Acta Biomater. 2, 377–385. doi: 10.1016/j.actbio.2006.02.005
Liao, S., Wang, W., Uo, M., Ohkawa, S., Akasaka, T., Tamura, K., et al. (2005). A three-layered nano-carbonated hydroxyapatite/collagen/PLGA composite membrane for guided tissue regeneration. Biomaterials 26, 7564–7571. doi: 10.1016/j.biomaterials.2005.05.050
Liu, G. T., Ding, Z. F., Yuan, Q. J., Xie, H. X., and Gu, Z. P. (2018). Multi-layered hydrogels for biomedical applications. Front. Chem. 6:449. doi: 10.3389/fchem.2018.00449
Lotfi, G., Shokrgozar, M. A., Mofid, R., Abbas, F. M., Ghanavati, F., Baghban, A. A., et al. (2015). Biological evaluation (in vitro and in vivo) of bilayered collagenous coated (nano electrospun and solid wall) chitosan membrane for periodontal guided bone regeneration. Ann. Biomed. Eng. 44, 1–13. doi: 10.1007/s10439-015-1516-z
Lu, Z. R., Zhou, Q., Wang, C. S., Wei, Z. J., Xu, L. N., and Gui, Y. G. (2018). Electrospun ZnO-SnO2 composite nanofibers and enhanced sensing properties to SF6 decomposition byproduct H2S. Front. Chem. 6:540. doi: 10.3389/fchem.2018.00540
Luo, Y., Shen, H., Fang, Y., Cao, Y., Huang, J., Zhang, M., et al. (2015). Enhanced proliferation and osteogenic differentiation of mesenchymal stem cells on graphene oxide-incorporated electrospun poly(lactic-co-glycolic acid) nanofibrous mats. ACS Appl. Mater. Interfaces 7, 6331–6339. doi: 10.1021/acsami.5b00862
Lyu, S., Huang, C., Yang, H., and Zhang, X. (2013). Electrospun fibers as a scaffolding platform for bone tissue repair. J. Orthop. Res. 31, 1382–1389. doi: 10.1002/jor.22367
Masoudi, R. M., Nouri, K. S., Ghasemi-Mobarakeh, L., Prabhakaran, M. P., Foroughi, M. R., Kharaziha, M., et al. (2017). Fabrication and characterization of two-layered nanofibrous membrane for guided bone and tissue regeneration application. Mater. Sci. Eng. C Mater. Biol. Appl. 80:75. doi: 10.1016/j.msec.2017.05.125
Mei, F., Zhong, J. S., Yang, X. P., Ouyang, X. Y., Zhang, S., Hu, X. Y., et al. (2007). Improved biological characteristics of poly(L-lactic acid) electrospun membrane by incorporation of multiwalled carbon nanotubes/hydroxyapatite nanoparticles. Biomacromolecules 8, 3729–3735. doi: 10.1021/bm7006295
Melcher, A. H. (1976). “Periodontal ligament,” in Orhan's Oral History and Embryology, ed S. N. Bhaskar (St. Louis, MO: C. V. Mosby Company), 213–214.
Min, L. L., Yuan, Z. H., Zhong, L. B., Liu, Q., Wu, R. X., and Zheng, Y. M. (2015). Preparation of chitosan based electrospun nanofiber membrane and its adsorptive removal of arsenate from aqueous solution. Chem. Eng. J. 267, 132–141. doi: 10.1016/j.cej.2014.12.024
Münchow, E. A., Albuquerque, M. T. P., Zero, B., Kamocki, K., Piva, E., Gregory, R. L., et al. (2015a). Development and characterization of novel ZnO-loaded electrospun membranes for periodontal regeneration. Dent. Mater. 31, 1038–1051. doi: 10.1016/j.dental.2015.06.004
Münchow, E. A., Pankajakshan, D., Albuquerque, M. T. P., Kamocki, K., Piva, E., Gregory, R. L., et al. (2015b). Synthesis and characterization of CaO-loaded electrospun matrices for bone tissue engineering. Clin. Oral Investig. 20, 1921–1933. doi: 10.1007/s00784-015-1671-5
Murakami, S., Miyaji, H., Nishida, E., Kawamoto, K., Miyata, S., Takita, H., et al. (2017). Dose effects of beta-tricalcium phosphate nanoparticles on biocompatibility and bone conductive ability of three-dimensional collagen scaffolds. Dent. Mater. J. 36, 573–583. doi: 10.4012/dmj.2016-295
Nasajpour, A., Ansari, S., Rinoldi, C., Rad, A. S., Aghaloo, T., Shin, S. R., et al. (2018). A multifunctional polymeric periodontal membrane with osteogenic and antibacterial characteristics. Adv. Funct. Mater. 28:1703437. doi: 10.1002/adfm.201703437
Niu, C., Yuan, K., Ma, R., Gao, L., Jiang, W., Hu, X., et al. (2017). Gold nanoparticles promote osteogenic differentiation of human periodontal ligament stem cells via the p38 MAPK signaling pathway. Mol. Med. Rep. 16, 4879–4886. doi: 10.3892/mmr.2017.7170
Nivedhitha, S. M., Sowmya, S., Deepthi, S., Bumgardener, J. D., and Jayakumar, R. (2016). Bilayered construct for simultaneous regeneration of alveolar bone and periodontal ligament. J. Biomed. Mater. Res. B Appl. Biomater. 104, 761–770. doi: 10.1002/jbm.b.33480
Pajoumshariati, S., Shirali, H., Yavari, S. K., Sheikholeslami, S. N., Lotfi, G., Mashhadi, A. F., et al. (2018). GBR membrane of novel poly (butylene succinate-co-glycolate) co-polyester co-polymer for periodontal application. Sci. Rep. 8:7513. doi: 10.1038/s41598-018-25952-1
Park, J. K., Yeom, J., Oh, E. J., Reddy, M., Kim, J. Y., Cho, D.-W., et al. (2009). Guided bone regeneration by poly(lactic-co-glycolic acid) grafted hyaluronic acid bi-layer films for periodontal barrier applications. Acta Biomater. 5, 3394–3403. doi: 10.1016/j.actbio.2009.05.019
Patel, R. M., Varma, S., Suragimath, G., and Zope, S. (2016). Estimation and comparison of salivary calcium, phosphorous, alkaline phosphatase and ph levels in periodontal health and disease: a cross-sectional biochemical study. J. Clin. Diagn. Res. 10, ZC58–ZC61. doi: 10.7860/JCDR/2016/20973.8182
Peter, M., Binulal, N. S., Soumya, S., Nair, S. V., Furuike, T., Tamura, H., et al. (2010). Nanocomposite scaffolds of bioactive glass ceramic nanoparticles disseminated chitosan matrix for tissue engineering applications. Carbohydr. Polym. 79, 284–289. doi: 10.1016/j.carbpol.2009.08.001
Polimeni, G., Xiropaidis, A. V., and Wikesjö, U. M. E. (2006). Biology and principles of periodontal wound healing/regeneration. Periodontol. 2000 41, 30–47. doi: 10.1111/j.1600-0757.2006.00157.x
Prabhakaran, M. P., Venugopal, J., and Ramakrishna, S. (2009). Electrospun nanostructured scaffolds for bone tissue engineering. Acta Biomater. 5, 2884–2893. doi: 10.1016/j.actbio.2009.05.007
Qasim, S. B., Delaine-Smith, R. M., Fey, T., Rawlinson, A., and Rehman, I. U. (2015). Freeze gelated porous membranes for periodontal tissue regeneration. Acta Biomater. 23, 317–328. doi: 10.1016/j.actbio.2015.05.001
Qasim, S. B., Najeeb, S., Delaine-Smith, R. M., Rawlinson, A., and Rehman, I. U. (2017). Potential of electrospun chitosan fibers as a surface layer in functionally graded GTR membrane for periodontal regeneration. Dent. Mater. 33, 71–83. doi: 10.1016/j.dental.2016.10.003
Ranjbar-Mohammadi, M., Zamani, M., Prabhakaran, M. P., Bahrami, S. H., and Ramakrishna, S. (2016). Electrospinning of PLGA/gum tragacanth nanofibers containing tetracycline hydrochloride for periodontal regeneration. Mater. Sci. Eng. C 58, 521–531. doi: 10.1016/j.msec.2015.08.066
Reise, M., Wyrwa, R., Mueller, U., Zylinski, M., Voelpel, A., Schnabelrauch, M., et al. (2012). Release of metronidazole from electrospun poly(L-lactide-co-D/L-lactide) fibers for local periodontitis treatment. Dent. Mater. 28, 179–188. doi: 10.1016/j.dental.2011.12.006
Reynolds, M. A., Aichelmann-Reidy, M. E., and Branch-Mays, G. L. (2010). Regeneration of periodontal tissue: bone replacement grafts. Dent. Clin. North Am. 54, 55–71. doi: 10.1016/j.cden.2009.09.003
Ripamonti, U., and Petit, J. C. (2009). Bone morphogenetic proteins, cementogenesis, myoblastic stem cells and the induction of periodontal tissue regeneration. Cytokine Growth Factor Rev. 20, 489–499. doi: 10.1016/j.cytogfr.2009.10.016
Schkarpetkin, D., Reise, M., Wyrwa, R., Voelpel, A., Berg, A., Schweder, M., et al. (2016). Development of novel electrospun dual-drug fiber mats loaded with a combination of ampicillin and metronidazole. Dent. Mater. 32, 951–960. doi: 10.1016/j.dental.2016.05.002
Shalumon, K. T., and Jyh-Ping, C. (2015). Scaffold-based drug delivery for cartilage tissue regeneration. Curr. Pharm. Des. 21, 1979–1990. doi: 10.2174/1381612821666150302152836
Shalumon, K. T., Sowmya, S., Sathish, D., Chennazhi, K. P., Nair, S. V., and Jayakumar, R. (2013). Effect of incorporation of nanoscale bioactive glass and hydroxyapatite in PCL/chitosan nanofibers for bone and periodontal tissue engineering. J. Biomed. Nanotechnol. 9, 430–440. doi: 10.1166/jbn.2013.1559
Shankhwar, N., Kumar, M., Mandal, B. B., and Srinivasan, A. (2016). Novel polyvinyl alcohol-bioglass 45S5 based composite nanofibrous membranes as bone scaffolds. Mater. Sci. Eng. C Mater. Biol. Appl. 69, 1167–1174. doi: 10.1016/j.msec.2016.08.018
Shao, J., Yu, N., Kolwijck, E., Wang, B., Tan, K. W., Jansen, J. A., et al. (2017). Biological evaluation of silver nanoparticles incorporated into chitosan-based membranes. Nanomedicine 12, 2771–2785. doi: 10.2217/nnm-2017-0172
Shao, W., He, J., Han, Q., Feng, S., Wang, Q., Li, C., et al. (2016). A biomimetic multilayer nanofiber fabric fabricated by electrospinning and textile technology from polylactic acid and Tussah silk fibroin as a scaffold for bone tissue engineering. Mater. Sci. Eng. C Mater. Biol. Appl. 67, 599–610. doi: 10.1016/j.msec.2016.05.081
Shen, R., Xu, W., Xue, Y., Chen, L., Ye, H., Zhong, E., et al. (2018). The use of chitosan/PLA nano-fibers by emulsion eletrospinning for periodontal tissue engineering. Artif. Cells 168:1. doi: 10.1080/21691401.2018.1458233
Shor, L., Güçeri, S., Wen, X., Gandhi, M., and Sun, W. (2007). Fabrication of three-dimensional polycaprolactone/hydroxyapatite tissue scaffolds and osteoblast-scaffold interactions in vitro. Biomaterials 28, 5291–5297. doi: 10.1016/j.biomaterials.2007.08.018
Sondi, I., and Salopeksondi, B. (2004). Silver nanoparticles as antimicrobial agent: a case study on E. coli as a model for gram-negative bacteria. J. Colloid Interface Sci. 275, 177–182. doi: 10.1016/S0021-9797(04)00163-8
Sowmya, S., Bumgardener, J. D., Chennazhi, K. P., Nair, S. V., and Jayakumar, R. (2013). Role of nanostructured biopolymers and bioceramics in enamel, dentin and periodontal tissue regeneration. Prog. Polym. Sci. 38, 1748–1772. doi: 10.1016/j.progpolymsci.2013.05.005
Stevens, M. M. (2005). Exploring and engineering the cell-surface interface. Science 310, 1135–1138. doi: 10.1126/science.1106587
Subbiah, T., Bhat, G. S., Tock, R. W., Parameswaran, S., and Ramkumar, S. S. (2005). Electrospinning of nanofibers. J. Appl. Polym. Sci. 96, 557–569. doi: 10.1002/app.21481
Sunandhakumari, V., Vidhyadharan, A., Alim, A., Kumar, D., Ravindran, J., Krishna, A., et al. (2018). Fabrication and in vitro characterization of bioactive glass/nano hydroxyapatite reinforced electrospun poly(ε-caprolactone) composite membranes for guided tissue regeneration. Bioengineering 5:E54. doi: 10.3390/bioengineering5030054
Tang, Y., Chen, L., Zhao, K., Wu, Z., Wang, Y., and Tan, Q. (2016). Fabrication of PLGA/HA (core)-collagen/amoxicillin (shell) nanofiber membranes through coaxial electrospinning for guided tissue regeneration. Compos. Sci. Technol. 125, 100–107. doi: 10.1016/j.compscitech.2016.02.005
Veríssimo, D. M., Leitão, R. F. C., Ribeiro, R. A., Figueiró, S. D., Sombra, A. S. B., Góes, J. C., et al. (2010). Polyanionic collagen membranes for guided tissue regeneration: effect of progressive glutaraldehyde cross-linking on biocompatibility and degradation. Acta Biomater. 6, 4011–4018. doi: 10.1016/j.actbio.2010.04.012
Wu, L., Hao, Y. Y., Shi, S. N., Zhang, X. P., Li, H. C., Sui, Y. L., et al. (2018). Controllable synthesis of Na3V2(PO4)3/C nanofibers as cathode material for sodium-ion batteries by electrostatic spinning. Front. Chem. 6:617. doi: 10.3389/fchem.2018.00617
Wu, X. N., Miao, L. Y., Yao, Y. F., Wu, W. L., Liu, Y., Chen, X. F., et al. (2014). Electrospun fibrous scaffolds combined with nanoscale hydroxyapatite induce osteogenic differentiation of human periodontal ligament cells. Int. J. Nanomedicine 9, 4135–4143. doi: 10.2147/IJN.S65272
Xia, W., Zhang, D., and Chang, J. (2007). Fabrication and in vitro biomineralization of bioactive glass (BG) nanofibres. Nanotechnology 18:135601. doi: 10.1088/0957-4484/18/13/135601
Xue, J., Shi, R., Niu, Y., Gong, M., Coates, P., Crawford, A., et al. (2015). Fabrication of drug-loaded anti-infective guided tissue regeneration membrane with adjustable biodegradation property. Colloids Surf B Biointerfaces 135, 846–854. doi: 10.1016/j.colsurfb.2015.03.031
Yang, F., Both, S. K., Yang, X., Walboomers, X. F., and Jansen, J. A. (2009). Development of an electrospun nano-apatite/PCL composite membrane for GTR/GBR application. Acta Biomater. 5, 3295–3304. doi: 10.1016/j.actbio.2009.05.023
Yang, F., Miao, Y., Wang, Y., Zhang, L. M., and Lin, X. (2017). Electrospun zein/gelatin scaffold-enhanced cell attachment and growth of human periodontal ligament stem cells. Materials 10:1168. doi: 10.3390/ma10101168
Yang, K., Zhang, J., Ma, X., Ma, Y., Kan, C., Ma, H., et al. (2015). β-Tricalcium phosphate/poly(glycerol sebacate) scaffolds with robust mechanical property for bone tissue engineering. Mater. Sci. Eng. C 56, 37–47. doi: 10.1016/j.msec.2015.05.083
Yeo, M., Lee, H., and Kim, G. (2011). Three-dimensional hierarchical composite scaffolds consisting of polycaprolactone, beta-tricalcium phosphate, and collagen nanofibers: fabrication, physical properties, and in vitro cell activity for bone tissue regeneration. Biomacromolecules 12, 502–510. doi: 10.1021/bm1013052
Zafar, M., Najeeb, S., Khurshid, Z., Vazirzadeh, M., Zohaib, S., Najeeb, B., et al. (2016). Potential of electrospun nanofibers for biomedical and dental applications. Materials 9:21. doi: 10.3390/ma9020073
Zaky, S. H., Hangadora, C. K., Tudares, M. A., Gao, J., Jensen, A., Wang, Y., et al. (2014). Poly (glycerol sebacate) elastomer supports osteogenic phenotype for bone engineering applications. Biomed. Mater. 9:025003. doi: 10.1088/1748-6041/9/2/025003
Zhang, B., Zhang, X. J., Bao, C. Y., Wang, Q., Yao, J. F., Fan, H. S., et al. (2007). Repairing periodontal bone defect with in vivo tissue engineering bone. Key Eng. Mater. 330–332, 1121–1124. doi: 10.4028/www.scientific.net/KEM.330-332.1121
Zhang, H., Wang, J., Wang, K., and Xu, L. (2018). A bilayered PLGA/multiwall carbon nanotubes/bacterial cellulose composite membrane for tissue regeneration of maxillary canine periodontal bone defects. Mater. Lett. 212, 118–121. doi: 10.1016/j.matlet.2017.10.058
Zhang, S., Jiang, G. J., Prabhakaran, M. P., Qin, X. H., and Ramakrishna, S. (2017). Evaluation of electrospun biomimetic substrate surface-decorated with nanohydroxyapatite precipitation for osteoblasts behavior. Mater. Sci. Eng. C Mater. Biol. Appl. 79, 687–696. doi: 10.1016/j.msec.2017.05.113
Zhou, H., Lawrence, J. G., and Bhaduri, S. B. (2012). Fabrication aspects of PLA-CaP/PLGA-CaP composites for orthopedic applications: a review. Acta Biomater. 8, 1999–2016. doi: 10.1016/j.actbio.2012.01.031
Zhou, T., Li, G., Lin, S., Tian, T., Ma, Q., Zhang, Q., et al. (2017a). Electrospun poly(3-hydroxybutyrate-co-4-hydroxybutyrate)/graphene oxide scaffold: enhanced properties and promoted in vivo bone repair in rats. ACS Appl. Mater. Interfaces 9, 42589–42600. doi: 10.1021/acsami.7b14267
Keywords: nano-composite, electrospun fibers, synthetic polymers, natural polymers, inorganic components, periodontal regeneration
Citation: Zhuang Y, Lin K and Yu H (2019) Advance of Nano-Composite Electrospun Fibers in Periodontal Regeneration. Front. Chem. 7:495. doi: 10.3389/fchem.2019.00495
Received: 28 January 2019; Accepted: 26 June 2019;
Published: 10 July 2019.
Edited by:
Bo Lei, Xi'an Jiaotong University, ChinaReviewed by:
Jianping Yang, Donghua University, ChinaCopyright © 2019 Zhuang, Lin and Yu. This is an open-access article distributed under the terms of the Creative Commons Attribution License (CC BY). The use, distribution or reproduction in other forums is permitted, provided the original author(s) and the copyright owner(s) are credited and that the original publication in this journal is cited, in accordance with accepted academic practice. No use, distribution or reproduction is permitted which does not comply with these terms.
*Correspondence: Kaili Lin, bGtsZWNudUBhbGl5dW4uY29t; Hongbo Yu, eWhiMzUwOEAxNjMuY29t
Disclaimer: All claims expressed in this article are solely those of the authors and do not necessarily represent those of their affiliated organizations, or those of the publisher, the editors and the reviewers. Any product that may be evaluated in this article or claim that may be made by its manufacturer is not guaranteed or endorsed by the publisher.
Research integrity at Frontiers
Learn more about the work of our research integrity team to safeguard the quality of each article we publish.