- 1State Key Laboratory of Integrated Optoelectronics, College of Electronic Science and Engineering, Jilin University, Changchun, China
- 2College of Communication Engineering, Jilin University, Changchun, China
Graphene is promising for developing soft and flexible electronic skin. However, technologies for graphene processing is still at an early stage, which limits the applications of graphene in advanced electronics. Laser processing technologies permits mask-free and chemical-free patterning of graphene, revealing the potential for developing graphene-based electronics. In this minireview, we overviewed and summarized the recent progresses of laser enabled graphene-based electronic skins. Two typical strategies, laser reduction of graphene oxide (GO) and laser induced graphene (LIG) on polyimide (PI), have been introduced toward the fabrication of graphene electronic skins. The advancement of laser processing technology would push forward the rapid progress of graphene electronic skin.
Introduction
As an ultrathin carbon material, graphene that features high conductivity, flexibility, transparency, bio-compatibility, and mechanical robustness have revealed great potential for developing soft and flexible electronics, especially, electronic skins (Badhulika et al., 2015; Han et al., 2016; Liao et al., 2018; Zeng et al., 2018; Zhu et al., 2018). To date, although graphene has been successfully prepared by mechanical exfoliation (e.g., tape method), chemical vapor deposition (CVD) on metal substrates (e.g., Cu and Ni), epitaxial growth, carbonization of polymers (e.g., laser induced graphene on polyimide, PI), solvents exfoliation and chemical oxidation of natural graphite, the application of graphene in soft electronics is still at an early stage (Chang and Wu, 2013; Han et al., 2015a, 2017; Cheng et al., 2017; Ye et al., 2018b). At present, the processing of graphene for electronic skin remains a challenging task. For instance, large-area and high-quality graphene have been obtained from CVD method. However, from the view point mass production and practical usage, the preparation of CVD graphene is energy-consumption, and the subsequent processing procedures usually involve complex substrate transfer and graphene patterning (Song et al., 2015). In this regard, advanced technologies enabling graphene processing is quite important for the progress of graphene-based soft electronics.
As a mask-free and chemical free method, laser processing of graphene has been successfully employed for both the preparation of graphene and the fabrication of graphene-based electronic skins (El-Kady and Kaner, 2014; Kymakis et al., 2014; Han et al., 2015b). Laser processing has been firstly used for the reduction and patterning of chemically derived graphene oxide (GO) (Zhang et al., 2014; Han et al., 2018; You et al., 2018). After laser treatments, the oxygen containing groups (OCGs) on GO sheets can be effectively removed, and therefore the conductivity can be recovered (Xiong et al., 2015; Hong et al., 2016). At the same time, any desired micropatterns can be directly created without the use of masks. Since the conductivity of laser reduced GO (LRGO) can be tuned by varying the laser intensity, laser reduction of GO has revealed great potential wearable soft sensors that can monitoring the human health (Zhao et al., 2017). Besides, laser processing has been extended to other graphene sources for patterning, structuring, layer control, heteroatom doping, and even device integration (Liu et al., 2016; Yoo et al., 2016). In recent years, laser treatment of PI has been proven an effective route for direct transform the polymer into grapheme (Ye et al., 2018a). Laser induced graphene (LIG) holds great promise for developing graphene-based electronic skins, since it is cost effective and permits flexible patterning.
In this minireview, we summarized the recent advancements in laser fabrication of graphene-based electronic skins (Figure 1). Unique features of laser reduction of GO and LIG technologies have been introduced, typical laser enabled graphene electronic skins have been reviewed. Current challenges and future perspective of this field have been briefly discussed.
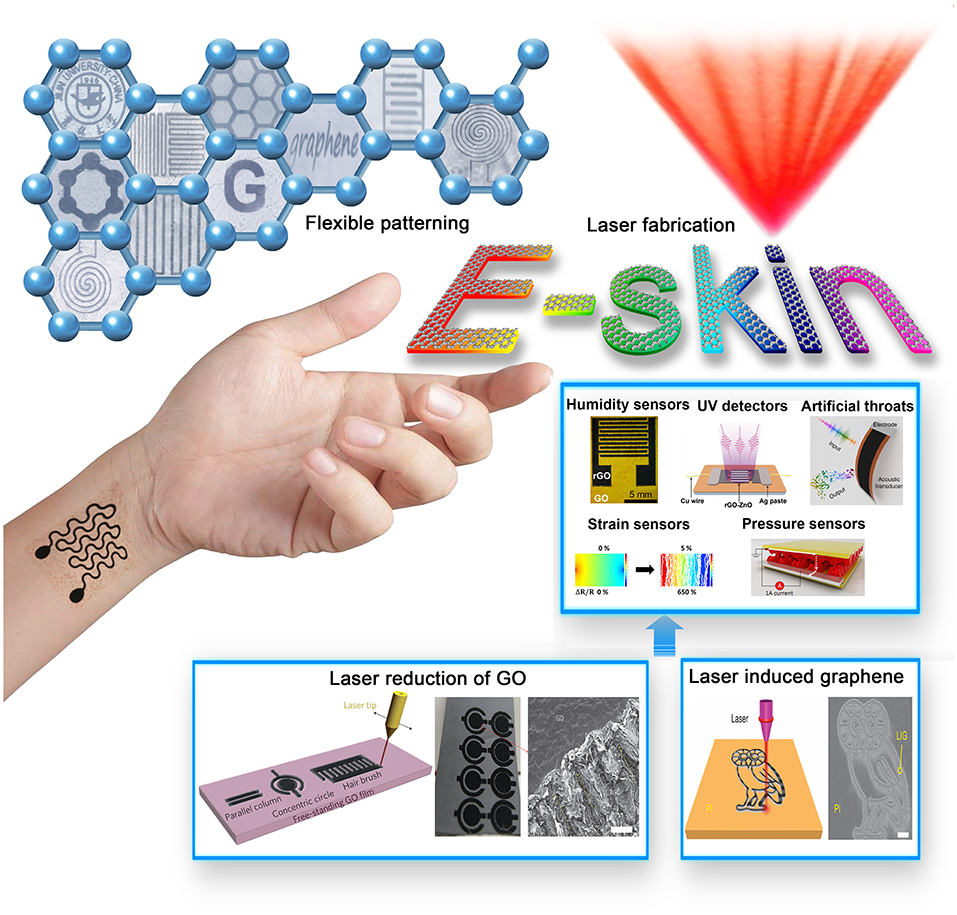
Figure 1. Laser fabrication of graphene-based electronic skin. Reproduced from Guo et al. (2014) with permission of WILEY-VCH. Reproduced from An et al. (2017) with permission of American Chemical Society. Reproduced from An et al. (2018) with permission of WILEY-VCH. Reproduced from Qiao et al. (2018) with permission of American Chemical Society. Reproduced from Tian et al. (2015) with permission of Nature Publishing Group. Reproduced from Tao et al. (2017) with permission of Nature Publishing Group. Reproduced from Gao et al. (2011) with permission of Nature Publishing Group. Reproduced from Lin et al. (2014) with permission of Nature Publishing Group.
Laser Reduction of GO
Laser induced GO reduction can be attributed to either photochemical or photothermal effects. Smirnov et al. investigated the threshold which light (λ ≤ 390 nm) is able to trigger the deoxygenation of GO through the photochemical way (Smirnov et al., 2011). As a pioneer in this field, Zhang et al. demonstrated LRGO using 800 nm femtosecond laser (Zhang et al., 2010a,b). Most of the OCGs were removed at the laser scanned region. LRGO micro-circuits (line width, 500 nm) have been firstly created through a mask free manner. Subsequently, the conductivity and bandgap were tailored by controlling the content of residual OCGs, which can be realized by exposing to different laser power (Guo et al., 2012b). The resistivity of LRGO is significantly reduced to 3.91 × 10−5 Ωm from insulation. Besides, GO film can be reduced by other lasers, for instance, a CO2 laser (Gao et al., 2011). Owing to the thermal effects, the resultant LRGO is highly porous. Similarly, LightScribe DVD optical drive was used to reduce GO (El-Kady et al., 2012). The resulted LRGO nanosheets resistance can be tailored by the grayscale color and laser irradiation times (Strong et al., 2012). In addition to flexible patterning, laser treatment also enables hierarchical structuring, which leads to anisotropic and conductivity (Guo et al., 2012a; Wang et al., 2012; Jiang et al., 2014). On the basis of these results, laser processing technology has been employed for developing soft electronic skins (El-Kady and Kaner, 2013).
Laser Induced Graphene
Recently, laser induced graphene has received great attention for their low cost, large-area, chemical-free and mask-free patterning. Tour's group found that porous graphene films were made from polyimide (PI) via CO2 laser irradiation (Lin et al., 2014). Upon laser irradiation, the C-O, C = O and N-C bonds of PI were broken due to the laser induced high temperature (>2,500°C), in this case, graphene formed through the recombination of atoms. The resultant LIG shows remarkably low sheet resistance (<15 Ω/sq) compared with PI. In addition to PI, other materials were also used to prepare graphene through a similar manner, such as wood (Ye et al., 2017), cloth (Chyan et al., 2018), polysulfone-class polymers (Singh et al., 2018). These methods provide a simple approach to fabricate graphene-based electronic skins.
Graphene-Based Electronic Skin
Based on LRGO or LIG, various graphene-based electronic skins have been developed. Taking advantage of the laser direct writing technologies, the properties of graphene-based electronic skin can be tuned by laser power, scanning paths, and scanning speeds. The resulted porous and patterned graphene is helpful for design of graphene-based electronic skin. In this section, we will briefly summarize some typical applications of LRGO and LIG in electronic skins.
Humidity Sensors
An et al. fabricated all-graphene based non-contact moisture-responsive electronic skin matrix (An et al., 2017). The highly reduced LRGO acts as the electrodes and the GO acts as the sensing materials. In this case, the humidity sensor enables direct measuring human breath and the distance between fingers and humidity sensors. In addition, a 4 × 4 sensing matrix was fabricated via laser direct writing technologies, which can detect the wet tip by the distribution of ΔZ/Z0. Moreover, the response and recovery time can be tailored by the OCGs contents of LRGO (Guo et al., 2012a).
UV Detectors
An et al. produced LRGO-ZnO composites-based UV detectors via single-step and selective laser writing (An et al., 2018). The high writing speed was used to prepare the electrodes and medium writing speed was used to prepare the sensing materials. The porous LRGO-ZnO composites can accelerate the photoresponse of the device, in which the response and recovery time are about 17.9 and 46.6 s, respectively. This flexible UV light detector can act as wearable electronics and prevent human body to the overexposure of UV light.
Strain Sensors
Wang et al. fabricated strain sensors based on the self-locked overlapping LRGO sheets (Wang et al., 2016). The strain sensor owns high gauge factor (>400). Furthermore, LRGO epidermal electronic skin was developed based on the crack directions and electrical characteristics (Qiao et al., 2018). The epidermal electronic skin (ultrahigh gauge factor of 673) is fabricated by the lift-off process based on based on laser scribed graphene. In this way, it can detect the respiration signal, pulse signal, and different finger bending degrees.
Pressure Sensors
Tian et al. developed graphene-based resistive pressure sensor with a sensitivity of 0.96 kPa−1 in the range from 0 to 50 kPa (Tian et al., 2015). This pressure sensor is made of two LRGO film, which are perpendicular to each other. When force was applied on the LRGO film, the contact area increases, and the electrical path ways become more. Therefore, the current increases with a fixed voltage. It can detect pressing, bending, twisting forces.
Temperature Sensors
Temperature response is another function of skin (Harada et al., 2014; He et al., 2015; Zhang et al., 2015; Cao and Wang, 2016; Trung et al., 2016). Sun et al. fabricated gas-permeable temperature sensors based on laser induced grapheme (Sun et al., 2018). This temperature sensor has ability in capturing temperature change of the skin, which the resistance changes as a function of temperature change (the error standard deviation, 0.25°C).
Artificial Throats
Tao et al. reported an LIG-based intelligent artificial throat (Tao et al., 2017). LIG can detect throat vibration based on the piezoresistive property and work as a sound source based on the thermoacoustic property. Therefore, LIG-based intelligent artificial throat can clearly distinguish cough, hum and scream with different tones and volumes. Importantly, this artificial throat enables voice recognition. The sound pressure level has been demonstrated from 100 to 40 kHz.
Conclusion and Outlook
Here, we have summarized two promising protocols for preparation and processing of graphene, LRGO and LIG. Taking advantage of the programmable processing manner of laser technologies, mask-free and chemical free patterning of graphene can be realized, revealing great potential for developing graphene-based electronic skins. The processing efficiency of laser fabrication is actually the key of large-scale applications. And the processing efficiency remains to be improved by spatial light modulator. Besides, the further trend of laser fabrication of graphene-based electronic skin can be integrated with various functional devices and/or reducing the device size. We believe that continued efforts in laser fabrication of graphene would push forward the rapid progress of this field and lead to breakthrough in both fundamental investigations and practical applications.
Author Contributions
All authors listed have made a substantial, direct and intellectual contribution to the work, and approved it for publication.
Funding
The authors acknowledge the National Key Research and Development Program of China and National Natural Science Foundation of China under Grant Nos. 2017YFB1104300, 61775078, 61590930, and 61605055 for support. We also acknowledge the Natural Science Foundation of Jilin Province Grant No. 20180101061JC.
Conflict of Interest Statement
The authors declare that the research was conducted in the absence of any commercial or financial relationships that could be construed as a potential conflict of interest.
References
An, J., Le, T. D., Huang, Y., Zhan, Z., Li, Y., Zheng, L., et al. (2017). All-graphene-based highly flexible noncontact electronic skin. ACS Appl. Mater. Interf. 9, 44593–44601. doi: 10.1021/acsami.7b13701
An, J., Le, T. D., Lim, C. H. J., Tran, V. T., Zhan, Z., Gao, Y., et al. (2018). Single-step selective laser writing of flexible photodetectors for wearable optoelectronics. Adv. Sci. 5:9. doi: 10.1002/advs.201800496
Badhulika, S., Terse-Thakoor, T., Villarreal, C., and Mulchandani, A. (2015). Graphene hybrids: synthesis strategies and applications in sensors and sensitized solar cells. Front. Chem. 3:19. doi: 10.3389/fchem.2015.00038
Cao, Y., and Wang, Y. (2016). Temperature-mediated regulation of enzymatic activity. Chemcatchem 8, 2740–2747. doi: 10.1002/cctc.201600406
Chang, H. X., and Wu, H. K. (2013). Graphene-based nanomaterials: synthesis, properties, and optical and optoelectronic applications. Adv. Funct. Mater. 23, 1984–1997. doi: 10.1002/adfm.201202460
Cheng, H., Huang, Y., Shi, G., Jiang, L., and Qu, L. (2017). Graphene-based functional architectures: sheets regulation and macrostructure construction toward actuators and power generators. Acc. Chem. Res. 50, 1663–1671. doi: 10.1021/acs.accounts.7b00131
Chyan, Y., Ye, R., Li, Y., Singh, S. P., Arnusch, C. J., and Tour, J. M. (2018). Laser-induced graphene by multiple lasing: toward electronics on cloth, paper, and food. ACA Nano 12, 2176–2183. doi: 10.1021/acsnano.7b08539
El-Kady, M. F., and Kaner, R. B. (2013). Scalable fabrication of high-power graphene micro-supercapacitors for flexible and on-chip energy storage. Nat. Commun. 4:9. doi: 10.1038/ncomms2446
El-Kady, M. F., and Kaner, R. B. (2014). Direct laser writing of graphene electronics. ACS Nano 8, 8725–8729. doi: 10.1021/nn504946k
El-Kady, M. F., Strong, V., Dubin, S., and Kaner, R. B. (2012). Laser scribing of high-performance and flexible graphene-based electrochemical capacitors. Science 335, 1326–1330. doi: 10.1126/science.1216744
Gao, W., Singh, N., Song, L., Liu, Z., Reddy, A. L. M., Ci, L. J., et al. (2011). Direct laser writing of micro-supercapacitors on hydrated graphite oxide films. Nat. Nanotechnol. 6, 496–500. doi: 10.1038/nnano.2011.110
Guo, L., Jiang, H. B., Shao, R. Q., Zhang, Y. L., Xie, S. Y., Wang, J. N., et al. (2012a). Two-beam-laser interference mediated reduction, patterning and nanostructuring of graphene oxide for the production of a flexible humidity sensing device. Carbon 50, 1667–1673. doi: 10.1016/j.carbon.2011.12.011
Guo, L., Shao, R. Q., Zhang, Y. L., Jiang, H. B., Li, X. B., Xie, S. Y., et al. (2012b). Bandgap tailoring and synchronous microdevices patterning of graphene oxides. J. Phys. Chem. C 116, 3594–3599. doi: 10.1021/jp209843m
Guo, L., Zhang, Y. L., Han, D. D., Jiang, H. B., Wang, D., Li, X. B., et al. (2014). Laser-mediated programmable N doping and simultaneous reduction of graphene oxides. Adv. Optic. Mater. 2, 120–125. doi: 10.1002/adom.201300401
Han, D. D., Liu, Y. Q., Ma, J. N., Mao, J. W., Chen, Z. D., Zhang, Y. L., et al. (2018). Biomimetic graphene actuators enabled by multiresponse graphene oxide paper with pretailored reduction gradient. Adv. Mater. Technol. 3:7. doi: 10.1002/admt.201800258
Han, D. D., Zhang, Y. L., Jiang, H. B., Xia, H., Feng, J., Chen, Q. D., et al. (2015a). Moisture-responsive graphene paper prepared by self-controlledp. Adv. Mater. 27, 332–338. doi: 10.1002/adma.201403587
Han, D. D., Zhang, Y. L., Liu, Y., Liu, Y. Q., Jiang, H. B., Han, B., et al. (2015b). Bioinspired graphene actuators prepared by unilateral UV irradiation of graphene oxide papers. Adv. Funct. Mater. 25, 4548–4557. doi: 10.1002/adfm.201501511
Han, D. D., Zhang, Y. L., Ma, J. N., Liu, Y., Mao, J. W., Han, C. H., et al. (2017). Sunlight-reduced graphene oxides as sensitive moisture sensors for smart device design. Adv. Mater. Technol. 2:8. doi: 10.1002/admt.201700045
Han, D. D., Zhang, Y. L., Ma, J. N., Liu, Y. Q., Han, B., and Sun, H. B. (2016). Light-mediated manufacture and manipulation of actuators. Adv. Mater. 28, 8328–8343. doi: 10.1002/adma.201602211
Harada, S., Kanao, K., Yamamoto, Y., Arie, T, Akita, S., and Takei, K. (2014). Fully printed flexible fingerprint-like three-axis tactile and slip force and temperature sensors for artificial skin. ACS Nano 8, 12851–12857. doi: 10.1021/nn506293y
He, Y., Liao, S., Jia, H., Cao, Y., Wang, Z., and Wang, Y. (2015). A self-healing electronic sensor based on thermal-sensitive fluids. Adv. Mater. 27, 4622–4627 doi: 10.1002/adma.201501436
Hong, S., Lee, H., Yeo, J., and Ko, S. H. (2016). Digital selective laser methods for nanomaterials: from synthesis to processing. Nano Today 11, 547–564. doi: 10.1016/j.nantod.2016.08.007
Jiang, H. B., Zhang, Y. L., Han, D. D., Xia, H., Feng, J., Chen, Q. D., et al. (2014). Bioinspired fabrication of superhydrophobic graphene films by two-beam laser interference. Adv. Func. Mater. 24, 4595–4602. doi: 10.1002/adfm.201400296
Kymakis, E., Petridis, C., Anthopoulos, T. D., and Stratakis, E. (2014). Laser-assisted reduction of graphene oxide for flexible, large-Area optoelectronics. IEEE J. Select. Top. Quant. Electron. 20:10. doi: 10.1109/jstqe.2013.2273414
Liao, G., Hu, J., Chen, Z., Zhang, R., Wang, G., and Kuang, T. (2018). Preparation, properties, and applications of graphene-based hydrogels. Front. Chem. 6:5. doi: 10.3389/fchem.2018.00450
Lin, J., Peng, Z., Liu, Y., Ruiz-Zepeda, F., Ye, R., Samuel, E. L., et al. (2014). Laser-induced porous graphene films from commercial polymers. Nat. Commun. 5:8. doi: 10.1038/ncomms6714
Liu, Y. Q., Zhang, Y. L., Liu, Y., Jiang, H. B., Han, D. D., Han, B., et al. (2016). Surface and interface engineering of graphene oxide films by controllable photoreduction. Chem. Record 16, 1244–1255. doi: 10.1002/tcr.201500306
Qiao, Y., Wang, Y., Tian, H., Li, M., Jian, J., Wei, Y., et al. (2018). Multilayer graphene epidermal electronic skin. ACS Nano 12, 8839–8846. doi: 10.1021/acsnano.8b02162
Singh, S. P., Li, Y., Zhang, J., Tour, J. M., and Arnusch, C. J. (2018). Sulfur-doped laser-induced porous graphene derived from polysulfone-class polymers and membranes. ACS Nano 12, 289–297. doi: 10.1021/acsnano.7b06263
Smirnov, V. A., Arbuzov, A. A., Shul'ga, Y. M., Baskakov, S. A., Martynenko, V. M., Muradyan, V. E., et al. (2011). Photoreduction of graphite oxide. High Energy Chem. 45, 57–61. doi: 10.1134/s0018143911010176
Song, Y., Fang, W. J., Brenes, R., and Kong, J. (2015). Challenges and opportunities for graphene as transparent conductors in optoelectronics. Nano Today 10, 681–700. doi: 10.1016/j.nantod.2015.11.005
Strong, V., Dubin, S., El-Kady, M. F., Lech, A., Wang, Y., Weiller, B. H., et al. (2012). Patterning and electronic tuning of laser scribed graphene for flexible all-carbon devices. ACS Nano 6, 1395–1403. doi: 10.1021/nn204200w
Sun, B., McCay, R. N., Goswami, S., Xu, Y., Zhang, C., Ling, Y., et al. (2018). Gas-permeable, multifunctional on-skin electronics based on laser-induced porous graphene and sugar-templated elastomer sponges. Adv. Mater. 2018:1804327. doi: 10.1002/adma.201804327
Tao, L. Q., Tian, H., Liu, Y., Ju, Z. Y., Pang, Y., Chen, Y. Q., et al. (2017). An intelligent artificial throat with sound-sensing ability based on laser induced graphene. Nat. Commun. 8:8. doi: 10.1038/ncomms14579
Tian, H., Shu, Y., Wang, X. F., Mohammad, M. A., Bie, Z., Xie, Q. Y., et al. (2015). A graphene-based resistive pressure sensor with record-high sensitivity in a wide pressure range. Sci. Rep. 5:6. doi: 10.1038/srep08603
Trung, T. Q., Ramasundaram, S., Hwang, B. U., and Lee, N. E. (2016). An all-elastomeric transparent and stretchable temperature sensor for body-attachable wearable electronics. Adv. Mater. 28, 502–509. doi: 10.1002/adma.201504441
Wang, D. Y., Tao, L. Q., Liu, Y., Zhang, T. Y., Pang, Y., Wang, Q., et al. (2016). High performance flexible strain sensor based on self-locked overlapping graphene sheets. Nanoscale 8, 20090–20095. doi: 10.1039/c6nr07620c
Wang, J. N., Shao, R. Q., Zhang, Y. L., Guo, L., Jiang, H. B., Lu, D. X., et al. (2012). Biomimetic graphene surfaces with superhydrophobicity and iridescence. Chem. Asian J. 7, 301–304. doi: 10.1002/asia.201100882
Xiong, W., Zhou, Y. S., Hou, W. J., Jiang, L. J., Mahjouri-Samani, M., Park, J., et al. (2015). Laser-based micro/nanofabrication in one, two and three dimensions. Front. Optoelectron. 8, 351–378. doi: 10.1007/s12200-015-0481-3
Ye, R., James, D. K., and Tour, J. M. (2018b). Laser-induced graphene. Acc. Chem. Res. 51, 1609–1620. doi: 10.1021/acs.accounts.8b00084
Ye, R. Q., Chyan, Y., Zhang, J., Li, Y., Han, X., Kittrell, C., et al. (2017). Laser-induced graphene formation on wood. Adv. Mater. 29:7. doi: 10.1002/adma.201702211
Ye, R. Q., James, D. K., and Tour, J. M. (2018a). Laser-induced graphene: from discovery to translation. Adv. Mater. 2018:1803621. doi: 10.1002/adma.201803621
Yoo, J. H., Kim, E., and Hwang, D. J. (2016). Femtosecond laser patterning, synthesis, defect formation, and structural modification of atomic layered materials. MRS Bull. 41, 1002–1007. doi: 10.1557/mrs.2016.248
You, R., Han, D. D., Liu, F. M., Zhang, Y. L., and Lu, G. Y. (2018). Fabrication of flexible room-temperature NO2 sensors by direct laser writing of In2O3 and graphene oxide composites. Sensor Actuat. B Chem. 277, 114–120. doi: 10.1016/j.snb.2018.07.179
Zeng, B. B., Huang, Z. Q., Singh, A., Yao, Y., Azad, A. K., Mohite, A. D., et al. (2018). Hybrid graphene metasurfaces for high-speed mid-infrared light modulation and single-pixel imaging. Light Sci. Appl. 7:8. doi: 10.1038/s41377-018-0055-4
Zhang, F., Zang, Y., Huang, D., Di, C. A., and Zhu, D. (2015). Flexible and self-powered temperature–pressure dual-parameter sensors using microstructure-frame-supported organic thermoelectric materials. Nat. Commun. 6:8356. doi: 10.1038/ncomms9356
Zhang, Y. L., Chen, Q. D., Xia, H., and Sun, H. B. (2010a). Designable 3D nanofabrication by femtosecond laser direct writing. Nano Today 5, 435–448. doi: 10.1016/j.nantod.2010.08.007
Zhang, Y. L., Guo, L., Wei, S., He, Y. Y., Xia, H., Chen, Q. D., et al. (2010b). Direct imprinting of microcircuits on graphene oxides film by femtosecond laser reduction. Nano Today 5, 15–20. doi: 10.1016/j.nantod.2009.12.009
Zhang, Y. L., Guo, L., Xia, H., Chen, Q. D., Feng, J., and Sun, H. B. (2014). Photoreduction of graphene oxides: methods, properties, and applications. Adv. Optic. Mater. 2, 10–28. doi: 10.1002/adom.201300317
Zhao, Y., Han, Q., Cheng, Z. H., Jiang, L., and Qu, L. T. (2017). Integrated graphene systems by laser irradiation for advanced devices. Nano Today 12, 14–30. doi: 10.1016/j.nantod.2016.12.010
Keywords: laser, graphene, laser reduced graphene oxides, laser induced graphene, electronic skin
Citation: Liu Y-Q, Chen Z-D, Mao J-W, Han D-D and Sun X (2019) Laser Fabrication of Graphene-Based Electronic Skin. Front. Chem. 7:461. doi: 10.3389/fchem.2019.00461
Received: 20 April 2019; Accepted: 11 June 2019;
Published: 27 June 2019.
Edited by:
Yang Zhao, University of Michigan, United StatesCopyright © 2019 Liu, Chen, Mao, Han and Sun. This is an open-access article distributed under the terms of the Creative Commons Attribution License (CC BY). The use, distribution or reproduction in other forums is permitted, provided the original author(s) and the copyright owner(s) are credited and that the original publication in this journal is cited, in accordance with accepted academic practice. No use, distribution or reproduction is permitted which does not comply with these terms.
*Correspondence: Dong-Dong Han, aGFuZG9uZ2RvbmdAamx1LmVkdS5jbg==; Xiaoying Sun, c3VueHlAamx1LmVkdS5jbg==