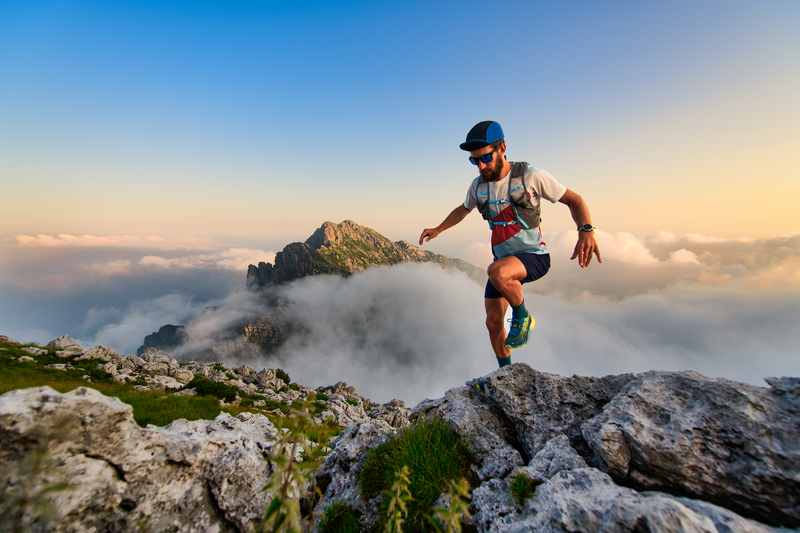
95% of researchers rate our articles as excellent or good
Learn more about the work of our research integrity team to safeguard the quality of each article we publish.
Find out more
METHODS article
Front. Chem. , 14 May 2019
Sec. Analytical Chemistry
Volume 7 - 2019 | https://doi.org/10.3389/fchem.2019.00342
This article is part of the Research Topic Frontiers in Chemistry: Rising Stars View all 75 articles
Viscosity of body fluid is an established biomarker of pathological conditions. Abnormality of cellular viscosity occurs when cells are challenged with external stresses. Small molecule probes to assess the viscosity are sought after for both disease diagnostics and basic studies. Fluorescence based probes are particular attractive due to their potentials for convenient and high spatiotemporal resolution microscopic monitoring of biological samples. The dyes with a floppy push-pull backbone or dyes with a rotatable substituent exhibits a viscosity responsive fluorescence enhancement and therefore viable viscosity probes. The scaffold of the existing viscosity probes contains typically one such floppy site. Therefore, they typically linearly respond to log(viscosity). We argue that minor viscosity fluctuation could potentially be physiological as the biological system is dynamic. We wish to develop a type of conceptually-new, threshold-limited viscosity probes, to complement the existing probes. Such probes do not exhibit a fluorescence enhancement when challenged with minor and presumably physiological enhancement of viscosity. When the viscosity is higher than a certain threshold, their fluorescence turns on. We hypothesize that a dye with two far-apart floppy sites could potentially yield such a threshold-limited signal and designed VPZ2 and VPZ3. Through spectral titration, VPZ3 was found to yield the desired threshold-limited signal. VPZ3 was suitable for in vitro bioimaging of viscosity under one-photon or two-photon excitation. VPZ3 is potentially useful in many downstream applications. Future work includes fine-tune of the threshold to allow tailored limit for fluorescence turn-on to better meet the need of different applications. Besides the implications in the real-world applications, the design concept could also be translated to design of alternative substrates.
Viscosity is a biophysical parameter of homeostasis (Tsien, 1989; Balkwill et al., 2012; Wang et al., 2017). By altering the molecular diffusion kinetics, biomolecular trafficking, lipid fluidity, and protein conformational rate, all physiological processes including enzymatic activity, energy metabolism, and signal transduction are affected (Miyamoto et al., 1990; Uribe and Smpedro, 2003; Boric et al., 2012; Liu et al., 2014; Sekhar et al., 2014). Abnormal fluctuation of microenvironmental viscosity is found to be reliable biomarker of underlying diseases or stresses (Aydemir et al., 2008; Harisa, 2014; Kasperczyk et al., 2014; Herranz et al., 2018). Oxidative burst can alter the membrane fluidity through lipid peroxidation and hence disrupts its function (Richter, 1987; Hormel et al., 2013). Lysosome storage disorders are associated with increased local viscosity (Platt et al., 2012; Devany et al., 2018). Viscosity is also vital to maintain the mitochondrial network organization and energy metabolism (Mecocci et al., 1997). Hyperviscosity, or macroscopic high blood viscosity, is found with patients of many blood diseases, such as myeloma, leukemia, anemia, and sepsis (Gustine et al., 2016). Therefore, fluorescent probes for viscosity are in need for both basic biomedical studies and disease diagnosis to monitor the viscosity of complex biological systems (Haidekker and Theodorakis, 2007; Kuimova et al., 2008; Sutharsan et al., 2010; Kuimova, 2012; Wang et al., 2013; López-Duarte et al., 2014; Yang et al., 2014; Chen et al., 2015; Vyšniauskas et al., 2015, 2016; Lee et al., 2016; Ren et al., 2016; Su et al., 2016; Zhu et al., 2016; Klymchenko, 2017; Lyubov et al., 2017; Ning et al., 2017;Song et al., 2017).
The capability of a dye to sense environmental viscosity originates from its excited state dynamics, including non-radiative rotational deactivation and radiative deactivation (Klymchenko, 2017). For a fluorophore exhibiting a high degree of rotational freedom, it is typically non-fluorescent. When the rotational freedom is restricted, possibility of radiative deactivation enhances. The structural freedom of the fluorophore may come from rotatable bonds of the push-pull backbone, as seen in P1 (Loutfy and Arnold, 1982), and P2 (Cui et al., 2019) (Figure 1). Or, a group may be installed to a rigid push-pull backbone via a single bond to construct a molecular rotor, e.g., P3 (Kuimova et al., 2008). Or, it could be a flexible push-pull backbone installed with a rotatable group, e.g., P4 (Peng et al., 2011), P5 (Babendure et al., 2003), and P6 (Colom et al., 2018) (Figure 1). Typically, these molecular rotors respond linearly to log(viscosity). Minor enhancement of microscopic viscosity could be physiological considering the dynamic nature of a biological system. Therefore, we are interested in development of a new class of molecular rotors which does not respond to minor enhancement of viscosity, until the viscosity surpasses a certain threshold limit. Such threshold-limited molecular rotors could potentially be very useful in disease diagnostics. The existence of one site of high rotational freedom in the scaffold of a fluorophore is required to yield a viscosity-sensitive fluorescence enhancement. We propose that two such sites are warranted to exhibit threshold-limited response to viscosity. Intuitively, minor enhancement of viscosity may restrict the rotational freedom of one site, leaving the other site unaffected to quench the fluorescence of the fluorophore. When the viscosity is higher than a certain limit, the chances of simultaneous restriction of both sites becomes possible and fluorescence enhancement should be noticeable. Also, sterics should be present in the scaffold of such a probe to minimize unintended fluorescence turn-on by aggregation or unselective binding with native biomacromolecules (Lei et al., 2017).
Herein, we report the synthesis, spectral titrations and proof-of-concept bioimaging of threshold-limited fluorescent probe (VPZ1-3) for microscopic viscosity.
VPZ1 is a known compound and synthesized according to the reported method (Lei et al., 2017). The synthesis VPZ2 and VPZ3 are displayed in Scheme 1 2-Bromo-4-fluorobenzaldehyde (1) was condensed with ethylene glycol in the presence of p-toluene-sulfonic acid to the corresponding 1,3-dioxolane derivative (2) in an 85% yield. Then 2 was treated with nBuLi at −78°C to generate the nucleophilic intermediate phenyllithium reagent in situ, which was quenched with DMF to yield the benzaldehyde derivative (3) in good yield. Through aldol condensation of 3 and 1,4-cyclohexanedione monoacetal under basic conditions, 4 was obtained in an 95% yield. (2-Phenoxyphenyl) lithium was added into a solution of 4 in THF. The resulting carbinol product was isolated and treated with methylsulfonic acid without further purification to afford 5 through a cascade of reactions. The crystal structure of the compound 5 was obtained and the diphenylether moiety and the bottom dinaphthylmethanone unit are perpendicular. Replacement of fluorine atoms of 5 with methoxide gave 6 in an 35% yield. Then the two methoxys groups of 6 was demethylated by BBr3 to get 7, the hydroxyls of which were converted to triflate by treatment of triflic anhydride to obtain the key intermediate 8. The viscosity probes (VPZ2 and VPZ3) were furnished by the Suzuki–Miyaura cross coupling reactions with 9 and 10, respectively. The NMR and the HRMS spectra of all new compounds are provided in the SI (Figures S1–S25). The crystal structure of compound 5 was obtained (Figure S26).
With the probes in hand, we firstly tested the photophysical properties of these probes (Figure 2, glycerol and water). The maximum absorption wavelength of VPZ1, VPZ2, and VPZ3 in pure water is about 470, 465, and 373 nm, respectively. Their fluorescence is very weak but still observable. The emission wavelength of VPZ1, VPZ2, and VPZ3 is about 650, 650, and 515 nm, respectively. With their symmetric D-A-D structure, the VPZ probes typically exhibit a Stokes shift of ca. 140–190 nm, much larger than that of a list of common fluorophores, such as fluorescein (24 nm), tetramethylrhodamine (25 nm), BODIPY (20 nm), Cy5 (20 nm), Cy7 (23 nm), which is desirable for imaging-based applications. We tested the viscosity related spectral responses (Figure 2). VPZ probes were added into solvent mixtures of water and glycerol exhibiting different viscosity values, their absorption and emission spectra were recorded. As shown in Figure 2, the absorption of VPZ probes exhibited only very subtle changes with respect to the increase of the solvent viscosity. The fluorescence emission intensity of VPZ1 and VPZ2 remained essentially unchanged with respect to the increase of the solvent viscosity. Notably, VPZ3 show a strong increase in fluorescence (ca.126-fold) with the increase of viscosity. Very interestingly, the fluorescence intensity of VPZ3 did not immediately increase when the solvent viscosity increased (Figure 3). Instead, the fluorescence intensity remained unchanged until the Log(viscosity) of the solution was higher than 10. After that, the fluorescence of VPZ3 enhanced linearly with respect to Log(viscosity). This is in good agreement with the design rational of the VPZ series of probes. The fluorescence life-time decay of VPZ3 in different solvent mixtures of H2O and glycerol was acquired (Figure S27). Since VPZ3 has the best performance against different viscosity, we chosen VPZ3 as the viscosity probe for the cell imaging study. The fact that VPZ1 and VPZ2 do not fluoresce in this solvent mixture is intriguing and subject to further in-depth photophysical studies.
Figure 2. Absorption (A, VPZ1; C, VPZ2; E, VPZ3) and emission (B, VPZ1; D, VPZ2; F, VPZ3) spectra of VPZ probes in different viscosity (glycerol and water), concentration: 10 μM.
The short absorption wavelength of VPZ3 limits its usage in imaging-based application in vivo with one photon excitation. To apply VPZ3 in cell study, we firstly performed the two-photon cross-section (δ) test (Figure 4) since the short one photon excitation problem can be circumvented by two-photon excitation. The maximum δ is about 80 GM at 770 nm in glycerol. Therefore, 770 nm was used for the cell imaging study.
Figure 4. Two-photon excitation action cross-section of VPZ3 in the glycerol–water system with different viscosities (20% glycerol and 100% glycerol). Two-photon excitation wavelength range: 680–880 nm (Δλ = 10 nm).
The cytotoxicity of VPZ3 was determined by MTT. In short, HepG2 cells were incubated with VPZ3 with different concentration for 24 h. The cell viability remained 85% at up to 25 μM (Figure S28). The low cytotoxicity of VPZ3 promotes us to further explore the possibility of VPZ3 as a fluorescence probe for the detection of cell viscosity. In order to demonstrate the imaging ability of VPZ3 in living cells, the HepG2 cells were incubated with VPZ3 (10 μM) at 37 and 25°C for 30 min separately. The ptimages of the cells were collected under two-photon excitation. As we known, lower temperature means higher viscosity for the cells. The fluorescence intensity of cells incubated at 25°C was higher than those at 37°C significantly (Figure 5). The imaging results supported that the VPZ3 could be used for monitoring the viscosity in the cytoplasm of living cells.
Figure 5. Two-photon confocal images of HepG2 cells incubated with VPZ3 (10 μM) after 30 min of incubation at (a1) 37°C and (a2) 25°C, respectively and then washed with 10 mM PBS buffer. λex = 770 nm, emission wavelength from 500 to 540 nm; (b1,b2) bright-field images of HepG2 cells; (c1) the overlay of panels (a1,b1); (c2) the overlay of (a2,b2). (d) Normalized intensity analysis of the probe at 37 and 25°C, respectively. Scale bars: 20 μm.
To further demonstrate the potentials of VPZ3 in monitoring the micro-viscosity change of cells, VPZ3 was used to monitor the real-time viscosity change during cell apoptosis. Because etoposide (a chemotherapy drug used to treat many types of cancer) can cause cell death, the micro-viscosity of the cells will change greatly during the apoptosis process. HepG2 cells were incubated with etoposide. The two-photon fluorescence images were collected at different times during the apoptosis process. As shown in Figure 6, the fluorescence intensity of the cells increased greatly during the apoptosis upon addition of etoposide. In contrast, the fluorescence of the cells without the addition of etoposide kept unchanged (Figure S29). These results clearly show that VPZ3 could be used to monitor the viscosity changes during the apoptosis process.
Figure 6. (a–h) Two-photon confocal images of HepG2 cells incubated with VP3 (10 μM) at different time points after the addition of 2.0 μM etoposide, λex = 770 nm, emission wavelength from 500 to 540 nm. (i) Plot of normalized intensities of the probe against time with (triangle, green line) and without (circle, blue line) etoposide. Scale bars: 20 μm.
In summary, we have developed a series of new fluorescence probes (VPZ) for viscosity monitoring based on a symmetric D-A-D framework. Among the three VPZ probes, VPZ3 showed a great “off-on” fluorescence response (ca. 126-fold) with increasing viscosity in the glycerol-water system. VPZ3 shows low cytotoxicity and could be used to monitor the real-time viscosity change during the cell apoptosis process. We expect VPZ3 to find applications in basic biological studies related cell apoptosis and disease diagnostics.
All datasets generated for this study are included in the manuscript and/or the Supplementary Files.
ZL prepared the compound synthesis. KX and SQ involved in the synthesis and carried spectral titrations. LH carried out biological studies. YY and XM conceived the project. Everyone contributed to manuscript preparation.
The authors declare that the research was conducted in the absence of any commercial or financial relationships that could be construed as a potential conflict of interest.
The Supplementary Material for this article can be found online at: https://www.frontiersin.org/articles/10.3389/fchem.2019.00342/full#supplementary-material
Aydemir, B., Onaran, I., Kiziler, A. R., Alici, B., and Akyolcu, M. C. (2008). The influence of oxidative damage on viscosity of seminal fluid in infertile men. J. Androl. 29, 41–46. doi: 10.2164/jandrol.107.003046
Babendure, J. R., Adams, S. R., and Tsien, R. Y. (2003). Aptamers switch on fluorescence of triphenylmethane dyes. J. Am. Chem. Soc. 125, 14716–14717. doi: 10.1021/ja037994o
Balkwill, F. R., Capasso, M., and Hagemann, T. (2012). The tumor microenvironment at a glance. J. Cell Sci. 125, 5591–5596. doi: 10.1242/jcs.116392
Boric, M., Danevcic, T., and Stopar, D. (2012). Viscosity dictates metabolic activity of Vibrio ruber. Front. Microbiol. 3:255. doi: 10.3389/fmicb.2012.00255
Chen, S., Hong, Y., Zeng, Y., Sun, Q., Liu, Y., Zhao, E., et al. (2015). Mapping live cell viscosity with an aggregation-induced emission fluorogen by means of two-photon fluorescence lifetime imaging. Chem. A Eur. J. 21, 4315–4320. doi: 10.1002/chem.201405658
Colom, A., Derivery, E., Soleimanpour, S., Tomba, C., Molin, M. D., Sakai, N., et al. (2018). A fluorescent membrane tension probe. Nat. Chem. 10, 1118–1125. doi: 10.1038/s41557-018-0127-3
Cui, J., Yao, Y., Chen, C., Huang, R., Zhang, W., and Qian, J. (2019). Mitochondria-targeted ratiometric fluorescent probes for micropolarity and microviscosity and their applications. Chin. Chem. Lett. doi: 10.1016/j.cclet.2018.12.031. [Epub ahead of print].
Devany, J., Chakraborty, K., and Krishnan, Y. (2018). Subcellular nanorheology reveals lysosomal viscosity as a reporter for lysosomal storage diseases. Nano Lett. 18, 1351–1359. doi: 10.1021/acs.nanolett.7b05040
Gustine, J., Meid, K., Manning, R. R., Dubeau, T., Ghobrial, I. M., Treon, S. P., et al.(2016). The high risk for symptomatic hyperviscosity in patients with high serum IgM levels can be used to support initiation of treatment in waldenström macroglobulinemia. Blood 128:2983. Available online at: http://www.bloodjournal.org/content/128/22/2983/tab-article-info
Haidekker, M. A., and Theodorakis, E. A. (2007). Molecular rotors—fluorescent biosensors for viscosity and flow. Organ. Biomol. Chem. 5, 1669–1678. doi: 10.1039/B618415D
Harisa, G. I. (2014). Blood viscosity as a sensitive indicator for paclitaxel induced oxidative stress in human whole blood. J. Androl. 23, 48–54. doi: 10.1016/j.jsps.2014.04.006
Herranz, B., Alvarez, M. D., and Perez-Jimenez, J. (2018). Association of plasma and urine viscosity with cardiometabolic risk factors and oxidative status. A pilot study in subjects with abdominal obesity. PLoS ONE 13:e0204075. doi: 10.1371/journal.pone.0204075
Hormel, T. T., Kurihara, S. Q., Brennan, M. K., and Parthasarathy, R. (2013). Measuring lipid membrane viscosity using rotational and translational particle diffusion. Biophys. J. 104, 426A−427A. doi: 10.1016/j.bpj.2012.11.2374
Kasperczyk, A., Słowinska-Łozynska, L., Dobrakowski, M., Zalejska-Fiolka, J., and Kasperczyk, S. (2014). The effect of lead-induced oxidative stress on blood viscosity and rheological properties of erythrocytes in lead exposed humans. Clin. Hemorheol. Microcirc. 56, 187–195. doi: 10.3233/CH-131678
Klymchenko, A. S. (2017). Solvatochromic and fluorogenic dyes as environment-sensitive probes: design and biological applications. Acc. Chem. Res. 50, 366–375. doi: 10.1021/acs.accounts.6b00517
Kuimova, M. K. (2012). Mapping viscosity in cells using molecular rotors. Phys. Chem. Chem. Phys. 14, 12671–12686. doi: 10.1039/C2CP41674C
Kuimova, M. K., Yahioglu, G., Levitt, J. A., and Suhling, K. (2008). Molecular rotor measures viscosity of live cells via fluorescence lifetime imaging. J. Am. Chem. Soc. 130, 6672–6673. doi: 10.1021/ja800570d
Lee, S. C., Heo, J., Ryu, J. W., Lee, C. L., Kim, S., Tae, J. S., et al. (2016). Pyrrolic molecular rotors acting as viscosity sensors with high fluorescence contrast. Chem. Commun. 52, 13695–13698. doi: 10.1039/C6CC06521J
Lei, Z., Li, X., Luo, X., He, H., Zheng, J., and Qian, X. (2017), Bright, stable, biocompatible organic fluorophores absorbing/emitting in the deep near-infrared spectral region. Angew. Chem. Int. Ed. 56, 2979–2983. doi: 10.1002/anie.201612301.
Liu, T., Liu, X., Spring, D. R., Qian, X., Cui, J., and Xu, Z. (2014). Quantitatively mapping cellular viscosity with detailed organelle information via a designed PET fluorescent probe. Sci. Rep. 4:5418. doi: 10.1038/srep05418
López-Duarte, I., Vu, T. T., Izquierdo, M. A., Bull, J. A., and Kuimova, M. K. (2014). A molecular rotor for measuring viscosity in plasma membranes of live cells. Chem. Commun. 50, 5282–5284. doi: 10.1039/C3CC47530A
Loutfy, R. O., and Arnold, B. A. (1982). Effect of viscosity and temperature on torsional relaxation of molecular rotors. J. Phys. Chem. 86, 4205–4211. doi: 10.1021/j100218a023
Lyubov, 'E.S, Izquierdo, M. A., López-Duarte, I., Bull, J. A., Shirmanova, M. V., Klapshina, L. G., et al. (2017). Imaging tumor microscopic viscosity in vivo using molecular rotors. Sci. Rep. 7:41097. doi: 10.1038/srep41097
Mecocci, P., Beal, M. F., Cecchetti, R., Polidori, M. C., Cherubini, A., Chionne, F., et al. (1997). Mitochondrial membrane fluidity and oxidative damage to mitochondrial DNA in aged and AD human brain. Mol. Chem. Neuropathol. 31, 53–64. doi: 10.1007/BF02815160
Miyamoto, A., Araiso, T., Koyama, T., and Ohshika, H. (1990). Membrane viscosity correlates with α1-adrenergic signal transduction of the aged rat cerebral cortex. J. Neurochem. 55, 70–75. doi: 10.1111/j.1471-4159.1990.tb08822.x
Ning, P., Dong, P., Geng, Q., Bai, L., Ding, Y., Tian, X., et al. (2017). A two-photon fluorescent probe for viscosity imaging in vivo. J. Mater. Chem. B 5, 2743–2749. doi: 10.1039/c7tb00136c
Peng, X., Yang, Z., Wang, J., Fan, J., He, Y., Song, F., et al. (2011). Fluorescence ratiometry and fluorescence lifetime imaging: using a single molecular sensor for dual mode imaging of cellular viscosity. J. Am. Chem. Soc. 133, 6626–6635. doi: 10.1021/ja1104014
Platt, F. M., Boland, B., and van der Spoel, A. C. (2012). Lysosomal storage disorders: the cellular impact of lysosomal dysfunction. J. Cell Biol. 199, 723–734. doi: 10.1083/jcb.201208152
Ren, M., Deng, B., Zhou, K., Kong, X., Wang, J. Y., and Lin, W. (2016). Single fluorescent probe for dual-imaging viscosity and H2O2 in mitochondria with different fluorescence signals in living cells. Anal. Chem. 89, 552–555. doi: 10.1021/acs.analchem.6b04385
Richter, C. (1987). Biophysical consequences of lipid peroxidation in membranes. Chem. Phys. Lipids 44, 175–189. doi: 10.1016/0009-308490049-1
Sekhar, A., Latham, M. P., Vallurupalli, P., and Kay, L. E. (2014). Viscosity-dependent kinetics of protein conformational exchange: microviscosity effects and the need for a small viscogen. J. Phys. Chem. B 118, 4546–4551. doi: 10.1021/jp501583t
Song, X., Li, N., Wang, C., and Xiao, Y. (2017). Targetable and fixable rotor for quantifying mitochondrial viscosity of living cells by fluorescence lifetime imaging. J. Mater. Chem. B 5, 360–368. doi: 10.1039/C6TB02524B
Su, D., Teoh, C., Gao, N., Xu, Q. H., and Chang, Y. T. (2016). A simple BODIPY-based viscosity probe for imaging of cellular viscosity in live cells. Sensors 16:1397. doi: 10.3390/s16091397
Sutharsan, J., Lichlyter, D., Wright, N. E., Dakanali, M., Haidekker, M. A., and Theodorakis, E. A. (2010). Molecular rotors: synthesis and evaluation as viscosity sensors. Tetrahedron 66, 2582–2588. doi: 10.1016/j.tet.2010.01.093
Uribe, S., and Smpedro, J. G. (2003). Measuring solution viscosity and its effect on enzyme activity. 5, 108–115. doi: 10.1251/bpo52
Vyšniauskas, A., Qurashi, M., Gallop, N., Balaz, M., Anderson, H. L., and Kuimova, M. K. (2015). Unravelling the effect of temperature on viscosity-sensitive fluorescent molecular rotors. Chem. Sci. 6, 5773–5778. doi: 10.1039/C5SC02248G
Vyšniauskas, A., Qurashi, M., and Kuimova, M. K. (2016). A molecular rotor that measures dynamic changes of lipid bilayer viscosity caused by oxidative stress. Chem. A Eur. J. 22, 13210–13217. doi: 10.1002/chem.201601925
Wang, L., Xiao, Y., Tian, W., and Deng, L. (2013). Activatable rotor for quantifying lysosomal viscosity in living cells. J. Am. Chem. Soc. 135, 2903–2906. doi: 10.1021/ja311688g
Wang, M., Zhao, J., Zhang, L., Wei, F., Lian, Y., Wu, Y., et al. (2017). Role of tumor microenvironment in tumorigenesis. J. Cancer 8, 761–773. doi: 10.7150/jca.17648
Yang, Z., Cao, J., He, Y., Yang, J. H., Kim, T., Peng, X., et al. (2014). Macro-/micro-environment-sensitive chemosensing and biological imaging. Chem. Soc. Rev. 43, 4563–4601. doi: 10.1039/c4cs00051j
Keywords: threshold-limited, probe, viscosity, two-photon, fluorescence
Citation: Lei Z, Xin K, Qiu S, Hou L, Meng X and Yang Y (2019) A Threshold-Limited Fluorescence Probe for Viscosity. Front. Chem. 7:342. doi: 10.3389/fchem.2019.00342
Received: 28 February 2019; Accepted: 25 April 2019;
Published: 14 May 2019.
Edited by:
Huangxian Ju, Nanjing University, ChinaCopyright © 2019 Lei, Xin, Qiu, Hou, Meng and Yang. This is an open-access article distributed under the terms of the Creative Commons Attribution License (CC BY). The use, distribution or reproduction in other forums is permitted, provided the original author(s) and the copyright owner(s) are credited and that the original publication in this journal is cited, in accordance with accepted academic practice. No use, distribution or reproduction is permitted which does not comply with these terms.
*Correspondence: Youjun Yang, eW91anVueWFuZ0BlY3VzdC5lZHUuY24=
Xiangming Meng, bWVuZ3htQGFodS5lZHUuY24=
Disclaimer: All claims expressed in this article are solely those of the authors and do not necessarily represent those of their affiliated organizations, or those of the publisher, the editors and the reviewers. Any product that may be evaluated in this article or claim that may be made by its manufacturer is not guaranteed or endorsed by the publisher.
Research integrity at Frontiers
Learn more about the work of our research integrity team to safeguard the quality of each article we publish.