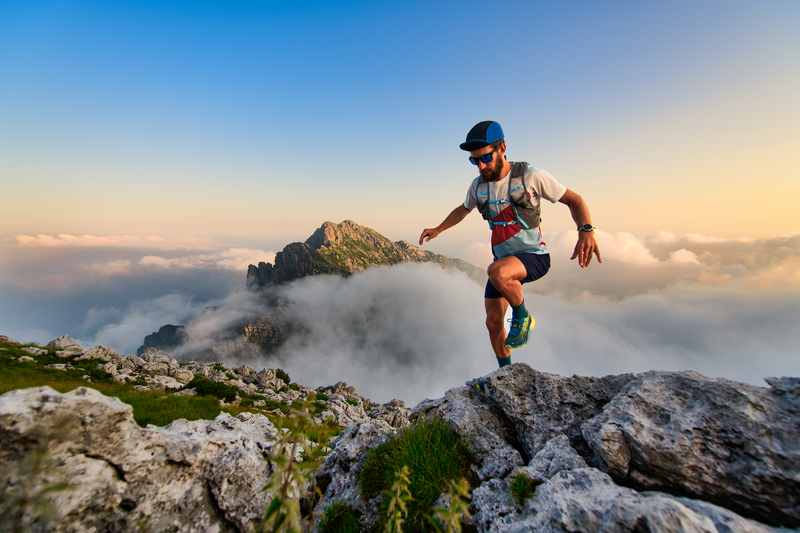
94% of researchers rate our articles as excellent or good
Learn more about the work of our research integrity team to safeguard the quality of each article we publish.
Find out more
REVIEW article
Front. Chem. , 14 May 2019
Sec. Nanoscience
Volume 7 - 2019 | https://doi.org/10.3389/fchem.2019.00335
This article is part of the Research Topic Frontiers in Chemistry: Rising Stars View all 75 articles
Sodium-ion batteries are considered to be the most promising alternative to lithium-ion batteries for large-scale stationary energy storage applications due to the abundant sodium resource in the Earth' crust and as a result, relatively low cost. Sodium layered transition metal oxides (NaxTMO2) are proper Na-ion cathode materials because of low cost and high theoretical capacity. Currently most researchers focus on the improvement of electrochemical performance such as high rate capability and long cycling stability. However, for NaxTMO2, the structure stability against humid atmosphere is essentially important since most of them are instable in air, which is not favorable for practical application. Here we provide a comprehensive review of recent progresses on air-stable NaxTMO2 oxides. Several effective strategies are discussed, and further investigations on the air-stable cathodes are prospected.
The growing demand for large-scale energy storage applications has driven the research interest into new energy storage systems with low cost. Although lithium-ion battery (LIB) can deliver high energy and power density, the limited resource and the rising cost of lithium may restrict their application in grid scale energy storage. Recently, sodium-ion battery (SIB), which owns a similar chemical storage mechanism to LIB, has been rapidly developed as a complementary technology. As the second lightest alkali metal, sodium resource is inexpensive and almost globally available. The common abundant sodium salt such as Na2SO4, NaCl, and Na2CO3 could be obtained from marine or mineral. In addition, copper foil can be replaced by cheaper aluminum foil for anode current collector since sodium has no reaction with aluminum. Therefore, SIB has received considerable attention as a promising alternative to LIB (Dunn et al., 2011; Yang et al., 2011, 2017; Palomares et al., 2013; Pan et al., 2013; Yabuuchi et al., 2014; Han et al., 2015; Kubota and Komaba, 2015; Kundu et al., 2015; Xiang et al., 2015; Hwang et al., 2017; Luo et al., 2017; Nayak et al., 2018; Zhu et al., 2019).
The SIB system consists of five parts: cathode, anode, membrane, electrolyte and current collector, which has the same structure as LIB. Figure 1 shows typical configuration of a SIB coin cell, in which sodium layered transition metal oxide (NaxTMO2) and hard carbon are employed as cathode and anode, respectively. During the charge process, the Na+ and e− migrates to hard carbon anode with voltage increasing. During the discharge process, Na+ and e− return to NaxTMO2 cathode reversibly with voltage decreasing. The overall reaction can be described as:
Numerous cathode materials such as polyanion compounds (Tripathi et al., 2013; Zhang Y. et al., 2016), layered transition metal (TM) oxides (Roger et al., 2007; Berthelot et al., 2011; Carlier et al., 2011) and Prussian blue or Metal-Organic compounds (Fang et al., 2017; Su et al., 2017; Qian et al., 2018) have been applied as Na+ host materials. Layered TM oxides show a high theoretical capacity among these cathode materials (Wang et al., 2018c). In addition, taking the preparation process and cost into consideration, the layered transition metal oxides are the optimal choice for practical application because they can be easily obtained by calcining the precursors in air. As a result, the layered transition metal oxides with general formula NaxTMO2 have attracted more and more attention since the first report by Delmas' group in the 1980s (Delmas et al., 1980, 1981).
Most of researches about NaxTMO2 focused on the improvement of electrochemical properties, such as: (i) eliminating Na+ vacancy ordering to improve rate capability; (Wang et al., 2015, 2018a; Kang et al., 2018) (ii) suppressing phase transition or surface coating to achieve long cycling life; (Wang et al., 2016c; Wang P.-F. et al., 2017; You et al., 2017; Sathiya et al., 2018) (iii) exploring oxygen ion redox mechanism to achieve high energy density (Rozier et al., 2015; Kim et al., 2017; Bai et al., 2018; Maitra et al., 2018; Qiao et al., 2018; Rong et al., 2018), and so on. However, most NaxTMO2 materials are hygroscopic and air-instable, which limit their practical applications because huge cost will be spent on materials' storage and transportation (Blesa et al., 1993; Franger et al., 2000; Lu and Dahn, 2001b; Caballero et al., 2002; Monyoncho and Bissessur, 2013; Duffort et al., 2015; Kubota and Komaba, 2015; Boyd et al., 2018; You et al., 2018). So in recent years, the design and synthesis of air-stable NaxTMO2 materials have become a hot topic. In this review, we summarize the recent progress on air-stable NaxTMO2 materials from structure understanding to corresponding solutions, and at the same time we address the remaining problems and challenges for further development.
In NaxTMO2 compounds, TM layers are usually occupied by Ti, (Senguttuvan et al., 2011; Wu D. et al., 2015) V, (Hamani et al., 2011; Guignard et al., 2013; Wang et al., 2018d) Cr, (Braconnier et al., 1982; Yu et al., 2015) Mn, (Ma et al., 2011) Fe, (Blesa et al., 1993; Yabuuchi et al., 2012b) Co, (Berthelot et al., 2011; Rai et al., 2014) Ni, (Vassilaras et al., 2013; Wang et al., 2018b) Cu, (Ono et al., 2014; Jiang et al., 2018; Ono, 2018) a mixture of two (Saadoune et al., 1996; Yabuuchi et al., 2012a; Mortemard de Boisse et al., 2013; Gonzalo et al., 2014; Guo et al., 2014; Kalluri et al., 2014; Zhu et al., 2014, 2016; Chen et al., 2015; Jiang et al., 2015; Kang et al., 2015; Wang et al., 2015, 2016d; Bucher et al., 2016; Kee et al., 2016; Liu et al., 2016; Manikandan et al., 2017; Sabi et al., 2017; Song et al., 2017) or more elements (Lu and Dahn, 2001b; Buchholz et al., 2014; Li et al., 2014; Liu et al., 2015; Li Y. et al., 2015; Li Z.-Y. et al., 2015; Yue et al., 2015; Han et al., 2016a; Kang et al., 2016; Qi et al., 2016; Satyanarayana et al., 2016; Sun et al., 2016; Wang et al., 2016b; Zhang X.-H. et al., 2016; Wang L. et al., 2017; Zheng and Obrovac, 2017) The corresponding redox potential ranges of these TM are presented in Figure 2. NaxTiO2 compound is usually used as anode material due to its low redox potential range. Nax(NiyMn1−y)O2 compound has been thoroughly investigated as cathode material because of the relatively high redox potential and theoretical capacity. (Lu and Dahn, 2001a; Fielden and Obrovac, 2015) V, Cr and Co substitution also shows a proper potential range for cathode but it may not suitable for practical application since V, Cr, and Co are expensive and toxic. Although Fe and Cu are almost electrochemical inactive when used as LiTMO2 for LIB system, (Ado et al., 1997; Arachi et al., 2012) these two elements are proven to be highly active in NaxTMO2 as Na-ion host. (Yabuuchi et al., 2012a; Ono, 2018) Since Ni and Co resources are mostly consumed by LIB system, the abundant Fe and Cu resources with low price are suitable for NaxTMO2 as sodium storage materials. (Li Y. et al., 2015; Mu et al., 2015) In addition, electrochemical inactive metal ions such as Li+, Mg2+ and Zn2+ could also be introduced into the TM layer for the improvement of electrochemical performance (Xu et al., 2014; Wu X. et al., 2015; Wang et al., 2016c). Table 1 lists the most common metal ions for the construction of TM layers and their corresponding ionic radii with coordination number of six (Shannon, 1976). Cations with similar ionic radius can partially substitute each other to form solid solutions, and hence various NaxTMO2 compounds could be designed by choosing two or more proper metal ions for the TM layer to improve electrochemical performance.ta
The crystal structure of NaxTMO2 can be usually classified into two types, P2 and O3 (Figure 3). The symbols of “P” and “O” are from the abbreviation of “prismatic” and “octahedral,” “2” and “3” represents the stacking arrangement per unit of O ions. For P2 type structure (usually x = 2/3), Na ions occupy two different prismatic sites, one shares faces between TMO6 octahedra called Naf sites and another shares edges between TMO6 octahedra called Nae sites. TM ions are surrounded by oxygen frameworks with a stacking mode of ABBA. For O3 structure (usually x = 1), all Na ions share one edge and one face with TMO6 octahedra. The oxygen frameworks are arranged in ABCABC pattern (Delmas et al., 1980, 1981; Shu and Chou, 2008; Morris et al., 2009; Toumar et al., 2015; Zheng C. et al., 2017).
Figure 3. The schematic of the crystal structure for layered sodium TM oxides, left is P2 type and right is O3 type.
So far, many researches have proven that the water and CO2 molecules from air can react with NaxTMO2, bringing negative influence on its morphology, crystal structure and electrochemical performance. The water molecules are easy to react with air-instable NaxTMO2 by inserting into the Na layer (Le Goff et al., 1993; Paulsen and Dahn, 1999; Franger et al., 2000; Lu and Dahn, 2001b; Caballero et al., 2002; Duffort et al., 2015; Boyd et al., 2018) or exchanging Na+ with H+, (Blesa et al., 1993; Monyoncho and Bissessur, 2013; Kubota and Komaba, 2015; Han et al., 2016b; Yao et al., 2017) leading to the expansion of interlayer spacing and the formation of impure phase (Figure 4). While CO2 can transform to CO on the surface of NaxTMO2 (Duffort et al., 2015; You et al., 2018). These air exposed NaxTMO2 usually show serious capacity decay and large polarization because of: (i) the side reaction between water and electrolyte (Kawamura et al., 2006; Lux et al., 2012; Han et al., 2016b); (ii) the active-materials' surface dissolution triggered by the acid attack of proton, which is released by water molecules (Blyr et al., 1998; Thackeray et al., 1998; Benedek and van de Walle, 2008); (iii) capacity and electronic conductivity decrease caused by inactive Na2CO3 layer (Duffort et al., 2015; You et al., 2018). Therefore, the air-instable NaxTMO2 cannot maintain its original crystal structure and electrochemical property under moisture atmosphere condition.
Figure 4. (A) Insertion of water molecules in Na+ layer, (B) Ion exchange between H+ and Na+ in Na+ layer.
Water molecules can insert into Na layer to form a NaxTMO2·yH2O hydrate phase, which usually occurs in P2 type structure (Le Goff et al., 1993; Franger et al., 2000; Lu and Dahn, 2001b; Caballero et al., 2002; Duffort et al., 2015; Boyd et al., 2018). In 2001, Lu et al. (Lu and Dahn, 2001b) studied the water insertion reaction mechanism for the first time in P2-Na2/3CoxNi1/3−xMn2/3O2 compound (x = 1/6 or 1/3). Compared with the XRD patterns of pristine Na2/3CoxNi1/3−xMn2/3O2, two new peaks around 14° and 28° were observed after exposing Na2/3CoxNi1/3−xMn2/3O2 samples in humid air environment (Figures 5A,B). These two peaks were assigned as hydrate phase due to the insertion of water molecules in Na layers. Rietveld refinement of hydrate Na2/3Co1/3Mn2/3O2·yH2O indicated that the ratio of water/Na is close to 1:1 and the oxygen atoms from water was in the 2c site of the crystal structure (Figures 5C,D). Franger et al. (Franger et al., 2000) investigated the influence of water soaking on α-Na0.7MnO2. With the increasing of water soaking time, the two peaks around 8° and 16° were vanished and four new peaks around 6.5°, 13°, 19° and 21° appeared gradually. The α-Na0.7MnO2 was totally transformed to Na0.45MnO2·0.6H2O after 60 min of water soaking treatment (Figure 5E). In 2018, Boyd et al. compared the air-stability of P2-Na0.62Ni0.22Mn0.66Fe0.1O2 (NaNMFe), P2-Na0.61Ni0.22Mn0.66Co0.1O2 (NaNMCo), P2-Na0.64Ni0.22Mn0.66Cu0.11O2 (NaNMCu) and P2-Na0.64Mn0.62Cu0.31O2 (NaMCu) samples. After air-exposure treatment of these four samples for 8 days, the XRD patterns of NaNMCu and NaMCu samples remained unchanged, while two new peaks around 12.5° and 25° appeared in the patterns of NaNMFe and NaNMCo samples, indicating that water can insert in the interlayer spacing of NaNMFe and NaNMCo samples (Figure 5F). From the STEM images of these four samples before and after air-exposure, an obvious extension in interlayer spacing could be seen after air-exposure, proving the insertion of water molecules in the interlayer spacing (Figure 5G). Although water molecules can insert into the interlayer spacing of P2-NaxTMO2 to form a P2-NaxTMO2·yH2O hydrate phase, NaxTMO2 phase can be regenerated by heat treatment at 200 °C to remove the water molecules (Lu and Dahn, 2001b).
Figure 5. (A) XRD patterns of pristine and air-exposed Na2/3Co1/6Ni1/6Mn2/3O2 samples, (B) XRD patterns of pristine and air-exposed Na2/3Co1/3Mn2/3O2 samples, (C) Rietveld refinement of (Na·H2O)2/3Co1/3Mn2/3O2, (D) Crystal structure of (Na·H2O)2/3Co1/3Mn2/3O2. Reproduced with permission (Lu and Dahn, 2001b). Copyright 2001, American Chemical Society. (E) Water molecules insert into the Na layer of Na0.7MnO2 and the change of XRD patterns with the increasing time of water soaking. Reproduced with permission (Franger et al., 2000). Copyright 2000, The Electrochemical Society. (F) XRD patterns of P2-Na0.62Ni0.22Mn0.66Fe0.1O2 (NaNMFe), P2-Na0.61Ni0.22Mn0.66Co0.1O2 (NaNMCo), P2-Na0.64Ni0.22Mn0.66Cu0.11O2 (NaNMCu) and P2-Na0.64Mn0.62Cu0.31O2 (NaMCu) samples after water soaking, (G) STEM images of pristine and water soaked NaNMFe, NaNMCo, NaNMCu and NaMCu samples. Reproduced with permission (Boyd et al., 2018). Copyright 2018, Royal Society of Chemistry.
For most O3-type NaTMO2, water molecules can release H+ to exchange the Na+, (Blesa et al., 1993; Monyoncho and Bissessur, 2013; Kubota and Komaba, 2015; Han et al., 2016b; Yao et al., 2017) which could be regarded as hydrolysis reaction:
Specially, if the TM layers contain a certain amount of Ni2+ ions, NiO would be emerged during the air exposure treatment:
This hydrolysis phenomenon has been confirmed in NaNi0.5Mn0.5O2, NaNi0.7Mn0.15Co0.15O2 and NaFeO2 compounds (Blesa et al., 1993; Monyoncho and Bissessur, 2013; Kubota and Komaba, 2015; You et al., 2018). A simple way to verify this hydrolysis reaction is to analyze the change of pH value after soaking NaTMO2 in deionized water due to the release of NaOH (Blesa et al., 1993). In 2013, Monyoncho and Bissessur reported that the pH of aqueous solution was higher than 12 after mixing O3-NaFeO2 sample with deionized water (Monyoncho and Bissessur, 2013). In addition, compared with the XRD patterns of pristine NaFeO2 sample, the 003 peak of hydrolysis Na1−xHxFeO2 sample became broader and shifted to low angle (Figure 6A), indicating the formation of a disordered crystal structure. Wang et al. investigated the hydrolysis reaction of O3-NaNi0.5Mn0.5O2 sample by testing the temperature after water soaking because this reaction can release heats (Yao et al., 2017). After NaNi0.5Mn0.5O2 was added into water, the temperature of the water was increased from 24.4 to 30.8°C. In contrast to the XRD pattern of as-synthesized sample, the 003 and 006 peaks of water soaked NaNi0.5Mn0.5O2 shifted to low angle with the generation of NiO impurity phase (Figure 6B). Importantly, unlike P2 type, the hydrolysis reaction between O3 type NaTMO2 and water is irreversible.
Figure 6. (A) XRD patterns of pristine and water soaked NaFeO2 samples. Reproduced with permission (Monyoncho and Bissessur, 2013). Copyright 2013, Elsevier. (B) XRD patterns of pristine, air exposed and water soaked NaNi0.5Mn0.5O2 samples. Reproduced with permission (Yao et al., 2017). Copyright 2017, American Chemical Society.
As mentioned above, NaOH is generated on the surface of O3 type NaxTMO2 during the air exposure process, then CO2 can further react with NaOH to form Na2CO3 (Sathiya et al., 2012; Monyoncho and Bissessur, 2013; You et al., 2018). This reaction can be described as:
Sathiya et al. (2012) proved the formation of Na2CO3 on the surface of NaNi1/3Mn1/3Co1/3O2 particles. Compared to the pristine sample (Figure 7a), the surface showed no obvious change after 15 days air-exposure (Figure 7b) but became quite rough after 30 days air-exposure (Figure 7c). IR spectrum revealed the bands of CO at 1,450 and 863 cm−1, suggesting the existence of sodium carbonates on NaNi1/3Mn1/3Co1/3O2 particles' surface (Figure 7d). Monyoncho and Bissessur (2013) extracted the aqueous solution from the mixture of NaFeO2 and water (Figure 7e). The XRD pattern of extracted sample matched very well to commercial Na2CO3, proving the reaction of CO2 and NaFeO2 compounds (Figure 7f).
Figure 7. SEM images of (a) pristine NaNi1/3Mn1/3Co1/3O2, (b) NaNi1/3Mn1/3Co1/3O2 after 15 days air-exposure, (c) NaNi1/3Mn1/3Co1/3O2 after 30 days air-exposure. (d) Infrared spectrum of NaNi1/3Mn1/3Co1/3O2 after 30 days air-exposure. Reproduced with permission (Sathiya et al., 2012). Copyright 2012, American Chemical Society. (e) SEM image of extracted sample, the sample is prepared by drying the aqueous solution of water-NaFeO2 mixture, (f) XRD patterns of the extracted sample and commercial Na2CO3. Reproduced with permission (Monyoncho and Bissessur, 2013). Copyright 2013, Elsevier.
After the formation of Na2CO3 on the surface, the CO can even be inserted into the TM layer, forming a “CO4” tetrahedron. Duffort et al. elucidated the mechanism of CO insertion in Na0.67Mn0.5Fe0.5O2 crystal (Duffort et al., 2015). With increasing the time of air exposure, ribbon-like particles start to appear and grow longer gradually (Figures 8a–c). In addition, the corresponding XRD patterns of Na0.67Mn0.5Fe0.5O2 are also changed. New peak is observed around 13° after a month air exposure (Figure 8d), indicating the formation of hydrate phase (phase 3) and sodium-depleted P2 phase (phase 2). In Fourier difference map, the existence of large residual nuclear density is caused by the carbonate ions (Figure 8e) because the insertion of CO leading to the changing of the nuclear density distribution (Figure 9g). In the TM layer, the CO is combined with one C-O bond to form a CO4 tetrahedron structure (Figure 8f).
Figure 8. (a) SEM images of Na0.67Mn0.5Fe0.5O2 after 1 day air-exposure, (b) Na0.67Mn0.5Fe0.5O2 after a couple of weeks' air-exposure, (c) Na0.67Mn0.5Fe0.5O2 after a couple of months' air-exposure, (d) XRD patterns of Na0.67Mn0.5Fe0.5O2 samples under different air-exposure time, (e) Nuclear Fourier difference map of Na0.67Mn0.5Fe0.5O2, (f) The local environment of CO, (g) The nuclear density around the carbon element position. Reproduced with permission (Duffort et al., 2015). Copyright 2015, American Chemical Society.
Figure 9. SEM images of (a) pristine NaNi0.7Mn0.15Co0.15O2, (b) NaNi0.7Mn0.15Co0.15O2 after 24 h air-exposure, (c) NaNi0.7Mn0.15Co0.15O2 after 7 days air-exposure, (d) dendrite-like impurity in part b with the corresponding elemental mappings of Na, C and Ni, (e) TOF-SIMS chemical mapping of NaNi0.7Mn0.15Co0.15O2 after 24 h exposure, (f) XRD patterns of NaNi0.7Mn0.15Co0.15O2 samples under different air exposed time, (g) the enlarged peaks from part f, (h) TGA profiles of pristine NaNi0.7Mn0.15Co0.15O2 and 7 days exposed NaNi0.7Mn0.15Co0.15O2 samples. Reproduced with permission (You et al., 2018). Copyright 2018, American Chemical Society.
Except for Na2CO3, other surface components are also observed. You et al. studied the surface reaction between NaNi0.7Mn0.15Co0.15O2 and air systematically by using time-of-flight secondary ion mass spectroscopy (TOF-SIMS) (You et al., 2018). The pristine sample shows a microsphere morphology, which is consisted of nanosized particles (Figure 9a). After 24 h air exposure treatment, the surface of this microsphere becomes smooth with the absence of nano-particles (Figure 9b). Finally, a thick layer of impurities is formed on the surface after 7 days air exposure (Figure 9c). Elements of Na, Ni, and C are distributed uniformly on the impurity surface (Figure 9d). The existence of NaNi+, NiO, NaC2O, C3H, Na2F+, and F− composition are confirmed by TOF-SIMS, indicating the surface degradation of NaNi0.7Mn0.15Co0.15O2 as well as the reaction between sodium carbonates and PVDF (Figure 9e). The 003 peak shifts to lower angles gradually because of the migration of Na+ to the surface while the 104 peak becomes weak and vanishes after 48 h air exposure (Figure 9f). According to the XRD patterns in Figure 9g, impurities' peaks such as NiO and Na2CO3 are observed, indicating the surface reaction when NaNi0.7Mn0.15Co0.15O2 contacts to CO2 and H2O. Since the CO2 and H2O are absorbed and reacted with NaNi0.7Mn0.15Co0.15O2, the air-exposed sample loses more weight than the fresh sample (Figure 9h). All the results above can prove hat NaNi0.7Mn0.15Co0.15O2 can react with the water and carbon dioxide in the air and the impurities of NaNi+, NiO, NaC2O, C3H, Na2F+ and F− are generated on the surface.
As mentioned above, NaTMO2 compounds can react with water and CO2 in air, which lead to: (i) the formation of impure phase on the surface; (ii) the insertion of H2O and CO into interlayer spacing and TM layers, respectively. On one side, the formed NaOH and Na2CO3 impure phase are electrochemical inactive and have low conductivity hence the rate capability of NaxTMO2 suffer serious decrease. On the other side, the water molecules can bring side reaction with electrolyte while the insertion of CO affects the valence state of TM ions, leading to severe capacity decay. Therefore, more and more researchers are focusing on strategies to address this air-instable problems.
One strategy is to prevent the materials from contacting moisture. During the materials preparation process, once the high-temperature treatment is done, the NaxTMO2 products are transferred to drying room (Wang et al., 2016a) or argon-filled glove box (Yabuuchi et al., 2012a; Vassilaras et al., 2014) immediately for the cooling process and subsequent cell assembling. However, this strategy may not be suitable for the large-scale application because huge cost will rise for materials' storage. Another strategy is to design air-stable NaxTMO2 material. Recently, several P2 and O3 type NaxTMO2 materials with high stability against moisture have been reported (Lu and Dahn, 2001b; Li Y. et al., 2015; Mu et al., 2015; Guo et al., 2017; Yao et al., 2017; Zheng L. et al., 2017; Chen et al., 2018; Deng et al., 2018). Under the treatment of air exposure and water soaking, these air-stable cathodes can maintain their original crystal structure and electrochemical performance. In this part, several effective strategies for air-stable NaxTMO2 designing are summarized.
Lu et al. first reported an air-stable P2-Na2/3Ni1/3Mn2/3O2 with high stability under moisture condition (Lu and Dahn, 2001b). For the P2-Na2/3Ni1/3Mn2/3O2 sample, after undergoing a 10 days air-exposure treatment, no peaks shift or new peaks formation were observed in the XRD pattern, indicating that water could not be inserted into the interlayer spacing (Figures 10A,B). According to neutron diffraction analysis, the Ni2+ and Mn4+ ions formed an honeycomb structure with an √3a × √3a ordering arrangement in the TM layers (Figure 10C) (Lu et al., 2000). This Ni/Mn ordering arrangement in TM layers was supposed to induce a strong interlayer interaction to prevent the water insertion. When this ordering arrangement was suppressed by the substitution of Co or Fe for Ni, water molecules could be inserted into the interlayer spacing (Figures 5A,B,F). However, this “interlayer interaction” between adjacent ordering TM layer has not been confirmed yet.
Figure 10. (A) XRD pattern of pristine Na2/3Ni1/3Mn2/3O2, (B) XRD pattern of Na2/3Ni1/3Mn2/3O2 after 10 days air-exposure, (C) the Ni/Mn cationic ordering arrangement. Reproduced with permission (Lu and Dahn, 2001b). Copyright 2001, American Chemical Society.
Coating a protective layer on the surface of NaxTMO2 is an effective method to prevent the NaxTMO2 from air contacting. The most common way is to coat high-voltage metal oxides with high stability against moisture. In 2017, Zhou and co-workers (Guo et al., 2017). designed an efficient spinel-like titanium (III) oxides protective interface to improve the structure/electrochemical stability of NaMnTi0.1Ni0.1O2. The sample surface was covered by a high Ti concentration layer with thickness of 2 nm, as shown in the electron energy-loss spectroscopy image (Figure 11a). Two distinct phases could be observed from the high-angle annular dark field scanning transmission electron microscopy (HAADF-STEM) image (Figure 11b). The bulk phase was a typical layered structure (Figure 11c) while the surface phase was spinel-like structure (Figures 11d,e). After exposing the naked bulk phase in humid air, two new peaks appeared around 12° and 25° (Figure 11f), indicating the insertion of water molecules. Compared to the naked bulk phase, the XRD pattern of NaMnTi0.1Ni0.1O2 sample showed no peak change since the spinel-like titanium (III) oxides interface can act as shield to protected the bulk phase from water attacking and the electrochemical performance of bulk phase can be maintained. In half cell system, the naked bulk phase showed a dramatic decrease after 50 cycles whereas NMTN sample only showed a slight decay after 100 cycles (Figure 11g).
Figure 11. (a) EELS chemical mapping of Ti element in NMTN sample, (b) STEM-HADDF image of NMTN sample, (c) Enlarged image extracted from the blue rectangle of part b, (d) Enlarged image extracted from the red rectangle of part b, (e) vulnerable mechanism of naked samples and protective mechanism of NMTN sample under moisture condition. (f) XRD patterns of naked sample (left) and NMTN sample (right) before/after air-exposure, (g) cycling performance of naked sample and NMTN sample. Reproduced with permission (Guo et al., 2017). Copyright 2017, Nature Publishing Group.
You et al. (2018) coated the surface of NaNi0.7Mn0.15Co0.15O2 with a ZrO2 protective layer. This protective layer notably maintains the rate capability of NaNi0.7Mn0.15Co0.15O2 against moisture atmosphere. After 7 days air exposure, the ZrO2@NaNi0.7Mn0.15Co0.15O2 sample still delivers a capacity of 96 mAh/g while NaNi0.7Mn0.15Co0.15O2 shows abnormal charge profile. The surface charge-transfer kinetics are also improved by this protective layer.
Except for Ti and Zr oxides, we suppose that other metal oxides such as MgO, ZnO, and Al2O3 also have the ability to work as protective layer because these high-voltage metal oxides all have high tolerance for moisture.
The Cu2+ substitution is the simplest way to obtain air-stable NaxTMO2 compounds. The success of Cu2+ substitution to achieve high stability against moisture has been proven by many reports (Li Y. et al., 2015; Mu et al., 2015; Yao et al., 2017; Zheng L. et al., 2017; Chen et al., 2018; Deng et al., 2018), few references give the working mechanisms of Cu2+ in these air-stable NaxTMO2 compounds.
In, 2017, Yao et al. designed an air-stable O3-NaNi0.45Cu0.05Mn0.4Ti0.1O2 (NaNCMT) cathode though cosubstitution of Cu2+ and Ti4+ in O3-NaNi0.5Mn0.5O2 (NaNM) compound. This strategy could decrease the Na+ interlayer distance and increase the valence state of TM ions. According to the refined crystal structure of NaNM and NaNCMT, the interlayer distance was reduced from 3.45 Å to 3.37 Å, respectively (Figure 12A), which was in favor of preventing the insertion of water molecules. DFT calculation revealed that Cu/Ti cosubstitution facilitated the increasing in valence state of Ni (Figure 12B). Compared with NaNM compound (Figure 6B), the XRD pattern of NaNCMT sample showed no obvious peaks change after air-exposure or water soaking (Figure 12C). During charge/discharge process, only slight capacity decay was observed after aging experiments (Figure 12D). (Yao et al., 2017) However, the explanation about the relationship between valence state of TM ions and air-stability was not mentioned.
Figure 12. (A) refined crystal structure of NaNM and NaNCMT samples, (B) Electronic density of states on Ni ion of NaNM, NaNCM, and NaNMT samples, respectively. (C) XRD patterns of pristine, air-exposure and water soaking NaNCMT samples. (D) charge/discharge curves of pristine, air-exposure and water soaking NaNCMT samples. Reproduced with permission (Yao et al., 2017). Copyright 2017, American Chemical Society.
Zheng et al. investigated the structure stability of Na2/3CuxNi1/3−xMn2/3O2 compounds (0 ≤ x ≤ 1/4) by air-exposure treatment. Compared to the XRD patterns of pristine samples, neither peaks position change nor new peaks formation were observed after exposing Na2/3CuxNi1/3−xMn2/3O2 in air condition for 21 days (Figure 13A). According to charge/discharge profiles, all exposed electrodes had a little higher open circuit voltage than the un-exposed electrodes, but the average voltage and reversible capacity of the exposed electrodes showed no change or decay, indicating the air stability of these electrodes (Figure 13B). Since the radii of Cu2+ (0.73 Å) and Ni2+ (0.69 Å) were similar, replacing Ni2+ in Na2/3Ni1/3Mn2/3O2 by Cu2+ had no influence on the Ni/Mn cationic ordering arrangement. Therefore, the existence of Cu/Ni/Mn ordering arrangement could prevent the insertion of water molecules into the Na2/3CuxNi1/3−xMn2/3O2 interlayer spacing because of the interlayer interaction between the adjacent Cu/Ni/Mn layer. However, no evidence was provided to prove the Cu/Ni/Mn ordering arrangement.
Figure 13. (A) XRD patterns of Na2/3CuxNi1/3−xMn2/3O2 samples (x = 0, 1/12, 1/6 and 1/4) before/after air-exposure, (B) the corresponding charge/discharge profiles. Reproduced with permission (Zheng L. et al., 2017). Copyright 2017, American Chemical Society.
Other compounds such as O3-Na0.9Cu0.22Fe0.30Mn0.48O2, P2-Na7/9Cu2/9Fe1/9Mn2/3O2, O3-NaLi0.05Mn0.5Ni0.3Cu0.1Mg0.05O2, and P2-Na0.6Mn0.9Cu0.1O2 have been proved to be air-stable because all of their XRD patterns remained unchanged after air-exposure and water soaking treatment (Li Y. et al., 2015; Mu et al., 2015; Chen et al., 2018; Deng et al., 2018).
It seems that the Cu2+ plays an important role in maintaining the structure stability of these compounds under moisture. The reported Cu2+ substituted NaxTMO2 compounds, such as O3-NaNi0.45Cu0.05Mn0.4Ti0.1O2, P2-Na2/3CuxNi1/3−xMn2/3O2, O3-Na0.9Cu0.22Fe0.30Mn0.48O2, O3-NaLi0.05Mn0.5Ni0.3Cu0.1Mg0.05O2, P2-Na7/9Cu2/9Fe1/9Mn2/3 O2, and P2-Na0.6Mn0.9Cu0.1O2, all show excellent structure stability under moisture condition. However, few investigations explain the working mechanism of Cu2+ substitution in these air-stable compounds. In O3-NaNi0.45Cu0.05Mn0.4Ti0.1O2 compound system, the working mechanism of Cu2+ is attributed to the increase of the Ni valence state by DFT calculation, but the relationship between valence state of Ni and air-stability is not clear so far. In addition, how to explain the Ni free compound systems for their air stability after Cu2+ substitution still remains problems.
Table 2 lists most of the air-stable NaxTMO2 compounds published to date, including the design strategies and the corresponding electrochemical performance.
Air-stability is one of the key issues for practical application of NaxTMO2 SIB cathode materials. In recent years, with understanding the structure of NaxTMO2, several strategies have been developed to obtain air-stable NaxTMO2 compounds, including constructing TM ordering arrangement, coating protective layer and Cu2+ substitution. However, there still remain some challenges. For example, the reaction mechanism of the “strong interlayer interaction” for TM ordering arrangement as well as the substitution of Cu2+ and other cations should be further understood. In any case, we believe that the air-stable NaxTMO2 materials with low cost and high theoretical capacity are highly competitive as SIB cathode materials in the large-scale energy storage application.
RZ contributed conception and design of the manuscript. YZ organized the reference and wrote the first draft of the manuscript. All authors contributed to manuscript revision, read and approved the submitted version.
The authors declare that the research was conducted in the absence of any commercial or financial relationships that could be construed as a potential conflict of interest.
We gratefully acknowledge the financial support from the National Natural Science Foundation of China (Nos. 51602221 and 51632001), Shanghai Municipal Natural Science Foundation (16ZR1438400) and the Fundamental Research Funds for the Central Universities.
Ado, K., Tabuchi, M., Kobayashi, H., Kageyama, H., Nakamura, O., Inaba, Y., et al. (1997). Preparation of LiFeO2 with Alpha- NaFeO2-type structure using a mixed-alkaline hydrothermal method. J. Electrochem. Soc. 144, L177–L180.
Arachi, Y., Setsu, T., Ide, T., Hinoshita, K., and Nakata, Y. (2012). Reversible electrochemical reaction of CuO with Li in the LiCuO2 system. Solid State Ionics 225, 611–614. doi: 10.1016/j.ssi.2011.12.006
Bai, X., Sathiya, M., Mendoza-Sánchez, B., Iadecola, A., Vergnet, J., Dedryvère, R., et al. (2018). Anionic redox activity in a newly Zn-doped sodium layered oxide P2-Na2/3Mn1–yZnyO2 (0 < y < 0.23). Adv. Energy Mater. 8:1802379. doi: 10.1002/aenm.201802379
Benedek, R., and van de Walle, A. (2008). Free energies for acid attack reactions of lithium cobaltate. J. Electrochem. Soc. 155, A711–A715. doi: 10.1149/1.2954958
Berthelot, R., Carlier, D., and Delmas, C. (2011). Electrochemical investigation of the P2–NaxCoO2 phase diagram. Nat. Mater. 10, 74–80. doi: 10.1038/nmat2920
Blesa, M. C., Morán, E., Menéndez, N., Tornero, J. D., and Torrón, C. (1993). Hydrolysis of sodium orthoferrite [α-NaFeO2]. Mater. Res. Bull. 28, 837–847. doi: 10.1016/0025-5408(93)90025-9
Blyr, A., Sigala, C., Amatucci, G., Guyomard, D., Chabre, Y., Tarascon, J., et al. (1998). Self-discharge of LiMn2O4/C Li-Ion cells in their discharged state: understanding by means of three-electrode measurements. J. Electrochem. Soc. 145, 194–209. doi: 10.1149/1.1838235
Boyd, S., Dhall, R., LeBeau, J., and Augustyn, V. (2018). Charge storage mechanism and degradation of P2-type sodium transition metal oxides in aqueous electrolytes. J. Mater. Chem. A 6, 22266–22276. doi: 10.1039/C8TA08367C
Braconnier, J. J., Delmas, C., and Hagenmuller, P. (1982). Etude par desintercalation electrochimique des systemes NaxCrO2 et NaxNiO2. Mater. Res. Bull. 17, 993–1000. doi: 10.1016/0025-5408(82)90124-6
Bucher, N., Hartung, S., Franklin, J. B., Wise, A. M., Lim, L. M., Chen, H.-Y., et al. (2016). P2–NaxCoyMn1−yO2 (y = 0, 0.1) as cathode materials in sodium-ion batteries—effects of doping and morphology to enhance cycling stability. Chem. Mater. 28, 2041–2051. doi: 10.1021/acs.chemmater.5b04557
Buchholz, D., Chagas, L. G., Vaalma, C., Wu, L., and Passerini, S. (2014). Water sensitivity of layered P2/P3-NaxNi0.22Co0.11Mn0.66O2 cathode material. J. Mater. Chem. A 2, 13415–13421. doi: 10.1039/C4TA02627F
Caballero, A., Hernan, L., Morales, J., Sanchez, L., Pena, J. S., Aranda, M. A. G., et al. (2002). Synthesis and characterization of high-temperature hexagonal P2-Na0.6MnO2 and its electrochemical behaviour as cathode in sodium cells. J. Mater. Chem. 12, 1142–1147. doi: 10.1039/b108830k
Carlier, D., Cheng, J. H., Berthelot, R., Guignard, M., Yoncheva, M., Stoyanova, R., et al. (2011). The P2-Na2/3Co2/3Mn1/3O2 phase: structure, physical properties and electrochemical behavior as positive electrode in sodium battery. Dalton Trans. 40, 9306–9312. doi: 10.1039/c1dt10798d
Chen, T. R., Sheng, T., Wu, Z. G., Li, J. T., Wang, E.-H., Wu, C.-H., et al. (2018). Cu(2+) dual-doped layer-tunnel hybrid Na0.6Mn1−xCuxO2 as a cathode of sodium-ion battery with enhanced structure stability, electrochemical property, and air stability. ACS Appl. Mater. Interfaces 10, 10147–10156. doi: 10.1021/acsami.8b00614
Chen, X., Zhou, X., Hu, M., Liang, J., Wu, D., Wei, J., et al. (2015). Stable layered P3/P2 Na0.66Co0.5Mn0.5O2 cathode materials for sodium-ion batteries. J. Mater. Chem. A 3, 20708–20714. doi: 10.1039/C5TA05205J
Delmas, C., Braconnier, J.-J., Fouassier, C., and Hagenmuller, P. (1981). Electrochemical intercalation of sodium in NaxCoO2 bronzes. Solid State Ionics 3, 165–169. doi: 10.1016/0167-2738(81)90076-X
Delmas, C., Fouassier, C., and Hagenmuller, P. (1980). Structural classification and properties of the layered oxides. Physica B+C 99, 81–85. doi: 10.1016/0378-4363(80)90214-4
Deng, J., Luo, W.-B., Lu, X., Yao, Q., Wang, Z., Liu, H.-K., et al. (2018). High energy density sodium-ion battery with industrially feasible and air-stable O3-type layered oxide cathode. Adv. Energy Mater. 8:1701610. doi: 10.1002/aenm.201701610
Duffort, V., Talaie, E., Black, R., and Nazar, L. F. (2015). Uptake of CO2 in layered P2-Na0.67Mn0.5Fe0.5O2 insertion of carbonate anions. Chem. Mater. 27, 2515–2524. doi: 10.1021/acs.chemmater.5b00097
Dunn, B., Kamath, H., and Tarascon, J.-M. (2011). Electrical energy storage for the grid: a battery of choices. Science 334, 928–935. doi: 10.1126/science.1212741
Fang, C., Huang, Y., Yuan, L., Liu, Y., Chen, W., Huang, Y., et al. (2017). A metal–organic compound as cathode material with superhigh capacity achieved by reversible cationic and anionic redox chemistry for high-energy sodium-ion batteries. Angew. Chem. Int. Ed. 56, 6793–6797. doi: 10.1002/anie.201701213
Fielden, R., and Obrovac, M. N. (2015). Investigation of the NaNixMn1−xO2 (0 < = x < = 1) system for na-ion battery cathode materials. J. Electrochem. Soc 162, A453–A459. doi: 10.1149/2.0551503jes
Franger, S., Bach, S., Pereira-Ramos, J. P., and Baffier, N. (2000). Chemistry and electrochemistry of low-temperature manganese oxides as lithium intercalation compounds. J. Electrochem. Soc. 147, 3226–3230. doi: 10.1149/1.1393887
Gonzalo, E., Han, M. H., del Amo, J. M. L., Acebedo, B., Casas-Cabanas, M., Rojo, T., et al. (2014). Synthesis and characterization of pure P2-and O3-Na2/3Fe2/3Mn1/3O2 as cathode materials for Na ion batteries. J. Mater. Chem. A 2, 18523–18530. doi: 10.1039/C4TA03991B
Guignard, M., Didier, C., Darriet, J., Bordet, P., Elkaïm, E., and Delmas, C. (2013). P2-NaxVO2 system as electrodes for batteries and electron-correlated materials. Nat Mater. 12, 74–80. doi: 10.1038/nmat3478
Guo, S., Li, Q., Liu, P., Chen, M., and Zhou, H. (2017). Environmentally stable interface of layered oxide cathodes for sodium-ion batteries. Nat. Commun. 8:135. doi: 10.1038/s41467-017-00157-8
Guo, S., Yu, H., Liu, D., Tian, W., Liu, X., Hanada, N., et al. (2014). A novel tunnel Na0.61Ti0.48Mn0.52O2 cathode material for sodium-ion batteries. Chem. Commun. 50, 7998–8001. doi: 10.1039/c4cc02362e
Hamani, D., Ati, M., Tarascon, J.-M., and Rozier, P. (2011). NaxVO2 as possible electrode for Na-ion batteries. Electrochem. Commun. 13, 938–941. doi: 10.1016/j.elecom.2011.06.005
Han, M. H., Gonzalo, E., Sharma, N. J., López del Amo, M., Armand, M., Avdeev, M., et al. (2016a). High-performance P2-phase Na2/3Mn0.8Fe0.1Ti0.1O2 cathode material for ambient-temperature sodium-ion batteries. Chem. Mater. 28, 106–116. doi: 10.1021/acs.chemmater.5b03276
Han, M. H., Gonzalo, E., Singh, G., and Rojo, T. (2015). A comprehensive review of sodium layered oxides: powerful cathodes for Na-ion batteries. Energy Environ. Sci. 8, 81–102. doi: 10.1039/C4EE03192J
Han, M. H., Sharma, N., Gonzalo, E., Pramudita, J. C., Brand, H. E., et al. (2016b). Moisture exposed layered oxide electrodes as Na-ion battery cathodes. J. Mater. Chem. A 4, 18963–18975. doi: 10.1039/C6TA07950D
Hwang, J.-Y., Myung, S.-T., and Sun, Y.-K. (2017). Sodium-ion batteries: present and future. Chem. Soc. Rev. 46, 3529–3614. doi: 10.1039/C6CS00776G
Jiang, K., Xu, S., Guo, S., Zhang, X., Zhang, X., Qiao, Y., et al. (2018). A phase-transition-free cathode for sodium-ion batteries with ultralong cycle life. Nano Energy 52, 88–94. doi: 10.1016/j.nanoen.2018.07.042
Jiang, X., Liu, S., Xu, H., Chen, L., Yang, J., and Qian, Y. (2015). Tunnel-structured Na0.54Mn0.50Ti0.51O2 and Na0.54Mn0.50Ti0.51O2/C nanorods as advanced cathode materials for sodium-ion batteries. Chem. Commun. 51, 8480–8483. doi: 10.1039/C5CC02233A
Kalluri, S., Seng, K. H., Pang, W. K., Guo, Z., Chen, Z., Liu, H. K., et al. (2014). Electrospun P2-type Na2/3Fe1/2Mn1/2O2 hierarchical nanofibers as cathode material for sodium-ion batteries. ACS Appl. Mater. Interfaces 6, 8953–8958. doi: 10.1021/am502343s
Kang, S. M., Park, J.-H., Jin, A., Jung, Y. H., Mun, J., and Sung, Y.-E. (2018). Na+/vacancy disordered P2-Na0.67Co1−xTixO2: high-energy and high-power cathode materials for sodium ion batteries. ACS Appl. Mater. Interfaces 10, 3562–3570. doi: 10.1021/acsami.7b16077
Kang, W., Yu, D. Y., Lee, P.-K., Zhang, Z., Bian, H., Li, W., et al. (2016). P2-Type NaxCu0.15Ni0.20Mn0.65O2 cathodes with high voltage for high-power and long-life sodium-ion batteries. ACS Appl. Mater. Interfaces 8, 31661–31668. doi: 10.1021/acsami.6b10841
Kang, W., Zhang, Z., Lee, P.-K., Ng, T.-W., Li, W., Tang, Y., et al. (2015). Copper substituted P2-type Na0.67CuxMn1−xO2: a stable high-power sodium-ion battery cathode. J. Mater. Chem. A 3, 22846–22852. doi: 10.1039/C5TA06371J
Kawamura, T., Okada, S., and Yamaki, J.-I. (2006). Decomposition reaction of LiPF6-based electrolytes for lithium ion cells. J. Power Sources 156, 547–554. doi: 10.1016/j.jpowsour.2005.05.084
Kee, Y., Dimov, N., Champet, S., Gregory, D. H., and Okada, S. (2016). Investigation of Al-doping effects on the NaFe0.5Mn0.5O2 cathode for Na-ion batteries. Ionics 22, 2245–2248. doi: 10.1007/s11581-016-1839-2
Kim, D., Cho, M., and Cho, K. (2017). Rational design of Na(Li1/3Mn2/3)O2 operated by anionic redox reactions for advanced sodium-ion batteries. Adv Mater 29:1701788. doi: 10.1002/adma.201701788
Kubota, K., and Komaba, S. (2015). Review—practical issues and future perspective for Na-Ion batteries. J. Electrochem. Soc. 162, A2538–A2550. doi: 10.1149/2.0151514jes
Kundu, D., Talaie, E., Duffort, V., and Nazar, L. F. (2015). The emerging chemistry of sodium ion batteries for electrochemical energy storage. Angew. Chem. Int. Ed. Engl. 54, 3431–3448. doi: 10.1002/anie.201410376
Le Goff, P., Baffier, N., Bach, S., Pereira-Ramos, J. P., and Messina, R. (1993). Structural and electrochemical characteristics of a lamellar sodium manganese oxide synthesized via a sol-gel process. Solid State Ionics 61, 309–315. doi: 10.1016/0167-2738(93)90397-L
Li, X., Wu, D., Zhou, Y. N., Liu, L., Yang, X.-Q., Ceder, G., et al. (2014). O3-type Na(Mn0.25Fe0.25Co0.25Ni0.25)O2: a quaternary layered cathode compound for rechargeable Na ion batteries. Electrochem. Commun. 49, 51–54. doi: 10.1016/j.elecom.2014.10.003
Li, Y., Yang, Z., Xu, S., Mu, L., Gu, L., Hu, Y.-S., et al. (2015). Air-stable copper-based P2-Na7/9Cu2/9Fe1/9Mn2/3O2 as a new positive electrode material for sodium-ion batteries. Adv. Sci. 2:1500031. doi: 10.1002/advs.201500031
Li, Z.-Y., Gao, R., Sun, L., Hu, Z., and Liu, X. (2015). Designing an advanced P2-Na0.67Mn0.65Ni0.2Co0.15O2 layered cathode material for Na-ion batteries. J. Mater. Chem. A 3, 16272–16278. doi: 10.1039/C5TA02450A
Liu, L., Li, X., Bo, S.-H., Wang, Y., Chen, H., Twu, N., et al. (2015). High-performance P2-type Na2/3(Mn1/2Fe1/4Co1/4)O2 cathode material with superior rate capability for Na-Ion batteries. Adv. Energy Mater. 5:1500944. doi: 10.1002/aenm.201500944
Liu, Y., Fang, X., Zhang, A., Shen, C., Liu, Q., Enaya, H., et al. (2016). Layered P2-Na2/3[Ni1/3Mn2/3]O2 as high-voltage cathode for sodium-ion batteries: the capacity decay mechanism and Al2O3 surface modification. Nano Energy 27, 27–34. doi: 10.1016/j.nanoen.2016.06.026
Lu, Z., and Dahn, J. R. (2001a). In situ X-ray diffraction study of P2-Na2/3[Ni1/3Mn2/3]O2. J. Electrochem. Soc. 148, A1225–A1229. doi: 10.1149/1.1407247
Lu, Z., and Dahn, J. R. (2001b). Intercalation of water in P2, T2 and O2 structure Az[CoxNi1/3−xMn2/3]O2. Chem. Mater. 13, 1252–1257. doi: 10.1021/cm000721x
Lu, Z., Donaberger, R. A., and Dahn, J. R. (2000). Superlattice ordering of Mn, Ni, and Co in layered alkali transition metal oxides with P2, P3, and O3 structures. Chem. Mater. 12, 3583–3590. doi: 10.1021/cm000359m
Luo, W., Chen, X., Xia, Y., Chen, M., Wang, L., Wang, Q., et al. (2017). Surface and interface engineering of silicon-based anode materials for lithium-ion batteries. Adv. Energy Mater. 7:1701083. doi: 10.1002/aenm.201701083
Lux, S. F., Lucas, I. T., Pollak, E., Passerini, S., Winter, M., and Kostecki, R. (2012). The mechanism of HF formation in LiPF6 based organic carbonate electrolytes. Electrochem. Commun. 14, 47–50. doi: 10.1016/j.elecom.2011.10.026
Ma, X., Chen, H., and Ceder, G. (2011). Electrochemical properties of monoclinic NaMnO2. J. Electrochem. Soc. 158, A1307–A1312. doi: 10.1149/2.035112jes
Maitra, U., House, R. A., Somerville, J. W., Tapia-Ruiz, N., Lozano, J. G., Guerrini, N., et al. (2018). Oxygen redox chemistry without excess alkali-metal ions in Na2/3[Mg0.28Mn0.72]O2. Nat. Chem. 10:288. doi: 10.1038/nchem.2923
Manikandan, P., Heo, S., Kim, H. W., Jeong, H. Y., Lee, E., and Kim, Y. (2017). Structural characterization of layered Na0.5Co0.5Mn0.5O2 material as a promising cathode for sodium-ion batteries. J. Power Sources 363, 442–449. doi: 10.1016/j.jpowsour.2017.07.116
Monyoncho, E., and Bissessur, R. (2013). Unique properties of α-NaFeO2: De-intercalation of sodium via hydrolysis and the intercalation of guest molecules into the extract solution. Mater. Res. Bull. 48, 2678–2686. doi: 10.1016/j.materresbull.2013.03.027
Morris, D. J. P., Roger, M., Gutmann, M. J., Goff, J. P., Tennant, D. A., Prabhakaran, D., et al. (2009). Crystal-to-stripe reordering of sodium ions in NaxCoO2. Phys. Rev. B 79:100103. doi: 10.1103/PhysRevB.79.100103
Mortemard de Boisse, B., Carlier, D., Guignard, M., and Delmas, C. (2013). Structural and electrochemical characterizations of P2 and new O3-NaxMn1−yFeyO2 phases prepared by auto-combustion synthesis for Na-Ion batteries. J. Electrochem. Soc 160, A569–A574. doi: 10.1149/2.032304jes
Mu, L., Xu, S., Li, Y., Hu, Y.-S., Li, H., Chen, L., et al. (2015). Prototype sodium-ion batteries using an air-stable and Co/Ni-Free O3-layered metal oxide cathode. Adv. Mater. 27, 6928–6933. doi: 10.1002/adma.201502449
Nayak, P. K., Yang, L., Brehm, W., and Adelhelm, P. (2018). From lithium-ion to sodium-ion batteries: advantages, challenges, and surprises. Angew. Chem. Int. Ed. 57, 102–120. doi: 10.1002/anie.201703772
Ono, Y. (2018). Structural analysis of NaCuO2 cathode at various charged/discharged stages and its reaction mechanism. Electrochemistry 86, 309–314. doi: 10.5796/electrochemistry.18-6-E2674
Ono, Y., Yui, Y., Hayashi, M., Asakura, K., Kitabayashi, H., and Takahashi, K. I. (2014). Electrochemical properties of NaCuO2 for sodium-ion secondary batteries. ECS Trans. 58, 33–39. doi: 10.1149/05812.0033ecst
Palomares, V., Casas-Cabanas, M., Castillo-Martínez, E., Han, M. H., and Rojo, T. (2013). Update on Na-based battery materials. A growing research path. Energy Environ. Sci. 6, 2312–2337. doi: 10.1039/c3ee41031e
Pan, H., Hu, Y.-S., and Chen, L. (2013). Room-temperature stationary sodium-ion batteries for large-scale electric energy storage. Energy Environ. Sci. 6, 2338–2360. doi: 10.1039/c3ee40847g
Paulsen, J. M., and Dahn, J. R. (1999). Studies of the layered manganese bronzes, Na2/3[Mn1−xMx]O2 with M = Co, Ni, Li, and Li2/3[Mn1−xMx]O2 prepared by ion-exchange. Solid State Ionics 126, 3–24. doi: 10.1016/S0167-2738(99)00147-2
Qi, X., Wang, Y., Jiang, L., Mu, L., Zhao, C., Liu, L., et al. (2016). Sodium-Deficient O3-Na0.9Ni0.4MnxTi0.6−xO2 Layered-Oxide Cathode Materials for Sodium-Ion Batteries. Part. Part. Syst. Charact. 33, 538–544. doi: 10.1002/ppsc.201500129
Qian, J., Wu, C., Cao, Y., Ma, Z., Huang, Y., Ai, X., et al. (2018). Sodium-ion batteries: prussian blue cathode materials for sodium-ion batteries and other ion batteries. Adv. Energy Mater. 8:1870079. doi: 10.1002/aenm.201870079
Qiao, Y., Guo, S., Zhu, K., Liu, P., Li, X., Jiang, K., et al. (2018). Reversible anionic redox activity in Na3RuO4 cathodes: a prototype Na-rich layered oxide. Energy Environ. Sci. 11, 299–305. doi: 10.1039/C7EE03554C
Rai, A. K., Anh, L. T., Gim, J., Mathew, V., and Kim, J. (2014). Electrochemical properties of NaxCoO2 (x~0.71) cathode for rechargeable sodium-ion batteries. Ceram. Int. 40, 2411–2417. doi: 10.1016/j.ceramint.2013.08.013
Roger, M., Morris, D. J., Tennant, D. A, Gutmann, M. J., Goff, J. P., Hoffmann, J.-U., et al. (2007). Patterning of sodium ions and the control of electrons in sodium cobaltate. Nature 445:631. doi: 10.1038/nature05531
Rong, X., Liu, J., Hu, E., Liu, Y., Wang, Y., Wu, J., et al. (2018). Structure-induced reversible anionic redox activity in Na layered oxide cathode. Joule 2, 125–140. doi: 10.1016/j.joule.2017.10.008
Rozier, P., Sathiya, M., Paulraj, A.-R., Foix, D., Desaunay, T., Taberna, P.-L., et al. (2015). Anionic redox chemistry in Na-rich Na2Ru1−ySnyO3 positive electrode material for Na-ion batteries. Electrochem. Commun. 53, 29–32. doi: 10.1016/j.elecom.2015.02.001
Saadoune, I., Maazaz, A., Ménétrier, M., and Delmas, C. (1996). On the NaxNi0.6Co0.4o2system: physical and electrochemical studies. J. Solid State Chem. 122, 111–117. doi: 10.1006/jssc.1996.0090
Sabi, N., Doubaji, S., Hashimoto, K., Komaba, S., Amine, K., Solhy, A., et al. (2017). Layered P2-Na2/3Co1/2Ti1/2O2 as a high-performance cathode material for sodium ion batteries. J. Power Sources 342, 998–1005. doi: 10.1016/j.jpowsour.2017.01.025
Sathiya, M., Hemalatha, K., Ramesha, K., Tarascon, J. M., and Prakash, A. S. (2012). Synthesis, structure, and electrochemical properties of the layered sodium insertion cathode material: NaNi1/3Mn1/3Co1/3O2. Chem. Mater. 24, 1846–1853. doi: 10.1021/cm300466b
Sathiya, M., Jacquet, Q., Doublet, M.-L., Karakulina, O. M., Hadermann, J., and and Tarascon, J.-M. (2018). A chemical approach to raise cell voltage and suppress phase transition in O3 sodium layered oxide electrodes. Adv. Energy Mater. 0, 1702599. doi: 10.1002/aenm.201702599
Satyanarayana, M., Jibin, A. K., and Varadaraju, U. V. (2016). Synthesis, structural and electrochemical study of O3-NaNi0.4Mn0.4Co0.2O2 as a cathode material for Na-ion batteries. Rsc Adv. 6, 61334–61340. doi: 10.1039/C6RA06785A
Senguttuvan, P., Rousse, G., Seznec, V., Tarascon, J.-M., and Palacín, M. R. (2011). Na2Ti3O7: lowest voltage ever reported oxide insertion electrode for sodium ion batteries. Chem. Mater. 23, 4109–4111. doi: 10.1021/cm202076g
Shannon, R. D. (1976). Revised effective ionic-radii and systematic studies of interatomic distances in halides and chalcogenides. Acta Crystallogr. Sect. A 32, 751–767. doi: 10.1107/S0567739476001551
Shu, G. J., and Chou, F. C. (2008). Sodium-ion diffusion and ordering in single-crystal P2-NaxCoO2. Phys. Rev. B 78:052101. doi: 10.1103/PhysRevB.78.052101
Song, S., Kotobuki, M., Chen, Y., Manzhos, S., Xu, C., Hu, N., et al. (2017). Na-rich layered Na2Ti1−xCrxO3−x/2 (x = 0, 0.06): Na-ion battery cathode materials with high capacity and long cycle life. Sci. Rep. 7:373. doi: 10.1038/s41598-017-00346-x
Su, D., Cortie, M., Fan, H., and Wang, G. (2017). Prussian blue nanocubes with an open framework structure coated with PEDOT as high-capacity cathodes for lithium-sulfur batteries. Adv. Mater 29:1700587. doi: 10.1002/adma.201700587
Sun, X., Ji, X.-Y., Xu, H.-Y., Zhang, C.-Y., Shao, Y., Zang, Y., et al. (2016). Sodium insertion cathode material Na0.67Ni0.4Co0.2Mn0.4O2 with excellent electrochemical properties. Electrochim. Acta 208, 142–147. doi: 10.1016/j.electacta.2016.04.067
Thackeray, M. M., Shao-Horn, Y., Kahaian, A. J., Kepler, K. D., Skinner, E., Vaughey, J. T., et al. (1998). Structural fatigue in spinel electrodes in high voltage (4 V) Li/LixMn2O4 cells. Electrochem. Solid-State Lett. 1, 7–9. doi: 10.1149/1.1390617
Toumar, A. J., Ong, S. P., Richards, W. D., Dacek, S., and Cedert, G (2015). Vacancy ordering in O3-type layered metal oxide sodium-ion battery cathodes. Phys. Rev. Appl. 4:064002. doi: 10.1103/PhysRevApplied.4.064002
Tripathi, R., Wood, S. M., Islam, M. S., Nazar, L., and Nazar, L. F. (2013). Na-ion mobility in layered Na2FePO4F and olivine Na[Fe,Mn]PO4. Energy Environ. Sci. 6, 2257–2264. doi: 10.1039/c3ee40914g
Vassilaras, P., Ma, X., Li, X., and Ceder, G. (2013). Electrochemical properties of monoclinic NaNiO2. J. Electrochem. Soc 160, A207–A211. doi: 10.1149/2.023302jes
Vassilaras, P., Toumar, A. J., and Ceder, G. (2014). Electrochemical properties of NaNi1/3Co1/3Fe1/3O2 as a cathode material for Na-ion batteries. Electrochem. Commun. 38, 79–81. doi: 10.1016/j.elecom.2013.11.015
Wang, H., Gu, M., Jiang, J., Lai, C., and Ai, X. (2016a). An O3-type NaNi0.5Mn0.3Ti0.2O2 compound as new cathode material for room-temperature sodium-ion batteries. J. Power Sources 327, 653–657. doi: 10.1016/j.jpowsour.2016.07.109
Wang, J., He, X., Zhou, D., Schappacher, F., Zhang, X., Liu, H., et al. (2016b). O3-type Na[Fe1/3Ni1/3Ti1/3]O2 cathode material for rechargeable sodium ion batteries. J. Mater. Chem. A 4, 3431–3437. doi: 10.1039/C5TA10520J
Wang, L., Sun, Y.-G., Hu, L.-L., Piao, J.-Y., Guo, J., Manthiram, A., et al. (2017). Copper-substituted Na0.67Ni0.3−xCuxMn0.7O2 cathode materials for sodium-ion batteries with suppressed P2-O2 phase transition. J. Mater. Chem. A 5, 8752–8761. doi: 10.1039/C7TA00880E
Wang, P.-F., Xin, H., Zuo, T.-T., Li, Q., Yang, X., Yin, Y.-X., et al. (2018b). An abnormal 3.7 Volt O3-type sodium-ion battery cathode. Angew. Chem. Int. Ed. 57, 8178–8183. doi: 10.1002/anie.201804130
Wang, P.-F., Yao, H.-R., Liu, X.-Y., Zhang, J.-N., Gu, L., Yu, X.-Q., et al. (2017). Ti-substituted NaNi0.5Mn0.5−xTixO2 cathodes with reversible O3–P3 phase transition for high-performance sodium-ion batteries. Adv. Mater. 29:1700210. doi: 10.1002/adma.201700210
Wang, P.-F., You, Y., Yin, Y.-X., and Guo, Y.-G. (2016d). An O3-type NaNi0.5Mn0.5O2 cathode for sodium-ion batteries with improved rate performance and cycling stability. J. Mater. Chem. A 4, 17660–17664. doi: 10.1039/C6TA07589D
Wang, P.-F., You, Y., Yin, Y.-X., and Guo, Y.-G. (2018c). Layered oxide cathodes for sodium-ion batteries: phase transition, air stability, and performance. Adv. Energy Mater. 8:1701912. doi: 10.1002/aenm.201701912
Wang, P.-F., You, Y., Yin, Y.-X., Wang, Y.-S., Wan, L.-J., Gu, L., et al. (2016c). Suppressing the P2–O2 phase transition of Na0.67Mn0.67Ni0.33O2 by magnesium substitution for improved sodium-ion batteries. Angew. Chem. Int. Ed. Engl. 55, 7445–7449. doi: 10.1002/anie.201602202
Wang, P. F., Yao, H. R., Liu, X. Y., Yin, Y.-X, Zhang, J. N., Wen, Y., et al. (2018a). Na(+)/vacancy disordering promises high-rate Na-ion batteries. Sci. Adv. 4:eaar6018. doi: 10.1126/sciadv.aar6018
Wang, Q., Xu, J., Zhang, W., Mao, M., Wei, Z., Wang, L., et al. (2018d). Research progress on vanadium-based cathode materials for sodium ion batteries. J. Mater. Chem. A 6, 8815–8838. doi: 10.1039/C8TA01627E
Wang, Y., Xiao, R., Hu, Y. S., Avdeev, M., and Chen, L. (2015). P2-Na0.6[Cr0.6Ti0.4]O2 cation-disordered electrode for high-rate symmetric rechargeable sodium-ion batteries. Nat. Commun. 6:6954. doi: 10.1038/ncomms7954
Wu, D., Li, X., Xu, B., Twu, N., Liu, L., and Ceder, G. (2015). NaTiO2: a layered anode material for sodium-ion batteries. Energy Environ. Sci. 8, 195–202. doi: 10.1039/C4EE03045A
Wu, X., Guo, J., Wang, D., Zhong, G., McDonald, M. J., and Yang, Y. (2015). P2-type Na0.66Ni0.33−xZnxMn0.67O2 as new high-voltage cathode materials for sodium-ion batteries. J. Power Sources 281, 18–26. doi: 10.1016/j.jpowsour.2014.12.083
Xiang, X., Zhang, K., and Chen, J. (2015). Recent advances and prospects of cathode materials for sodium-ion batteries. Adv. Mater. 27, 5343–5364. doi: 10.1002/adma.201501527
Xu, J., Lee, D. H., Clément, R. J., Yu, X., Leskes, M., Pell, A. J., et al. (2014). Identifying the critical role of Li substitution in P2–Nax[LiyNizMn1−y−z]O2 (0 < x, y, z < 1) intercalation cathode materials for high-energy Na-Ion batteries. Chem. Mater. 26, 1260–1269. doi: 10.1021/cm403855t
Yabuuchi, N., Kajiyama, M., Iwatate, J., Nishikawa, H., Hitomi, S., Okuyama, R., et al. (2012a). P2-type Nax[Fe1/2Mn1/2]O2 made from earth-abundant elements for rechargeable Na batteries. Nat. Mater. 11, 512–517. doi: 10.1038/nmat3309
Yabuuchi, N., Kubota, K., Dahbi, M., and Komaba, S. (2014). Research development on sodium-ion batteries. Chem. Rev. 114, 11636–11682. doi: 10.1021/cr500192f
Yabuuchi, N., Yoshida, H., and Komaba, S. (2012b). Crystal structures and electrode performance of alpha-NaFeO2 for rechargeable sodium batteries. Electrochemistry 80, 716–719. doi: 10.5796/electrochemistry.80.716
Yang, J., Wang, Y., Li, W., Wang, L., Fan, Y., Jiang, W., et al. (2017). Amorphous TiO2 shells: a vital elastic buffering layer on silicon nanoparticles for high-performance and safe lithium storage. Adv. Mater. 29:1700523. doi: 10.1002/adma.201700523
Yang, Z., Zhang, J., Kintner-Meyer, M. C., Lu, X., Choi, D., Lemmon, J. P., et al. (2011). Electrochemical energy storage for green grid. Chem. Rev. 111, 3577–3613. doi: 10.1021/cr100290v
Yao, H.-R., Wang, P.-F., Gong, Y., Zhang, J., Yu, X., Gu, L., et al. (2017). Designing air-stable O3-type cathode materials by combined structure modulation for Na-Ion batteries. J. Am. Chem. Soc. 139, 8440–8443. doi: 10.1021/jacs.7b05176
You, Y., Dolocan, A., Li, W., and Manthiram, A. (2018). Understanding the air-exposure degradation chemistry at a nanoscale of layered oxide cathodes for sodium-ion batteries. Nano Lett. 19, 182–188 doi: 10.1021/acs.nanolett.8b03637
You, Y., Kim, S. O., and Manthiram, A. (2017). A Honeycomb-layered oxide cathode for sodium-ion batteries with suppressed P3–O1 phase transition. Adv. Energy Mater. 7:1601698. doi: 10.1002/aenm.201601698
Yu, C.-Y., Park, J.-S., Jung, H.-G., Chung, K.-Y., Aurbach, D., Sun, Y.-K., et al. (2015). NaCrO2 cathode for high-rate sodium-ion batteries. Energy Environ. Sci. 8, 2019–2026. doi: 10.1039/C5EE00695C
Yue, J.-L., Yin, W.-W., Cao, M.-H., Zulipiya, S., Zhou, Y.-N., and Fu, Z.-W. (2015). A quinary layer transition metal oxide of NaNi1/4Co1/4Fe1/4Mn1/8Ti1/8O2 as a high-rate-capability and long-cycle-life cathode material for rechargeable sodium ion batteries. Chem. Commun. 51, 15712–15715. doi: 10.1039/C5CC06585B
Zhang, X.-H., Pang, W.-L., Wan, F., Guo, J.-Z., Lu, H.-Y., Li, J.-Y., et al. (2016). P2-Na2/3Ni1/3Mn5/9Al1/9O2 microparticles as superior cathode material for sodium-ion batteries: enhanced properties and mechanisam via graphene connection. ACS Appl. Mater. Interfaces 8, 20650–20659. doi: 10.1021/acsami.6b03944
Zhang, Y., Zhao, H., and Du, Y. (2016). Symmetric full cells assembled by using self-supporting Na3V2(PO4)3 bipolar electrodes for superior sodium energy storage. J. Mater. Chem. A 4, 7155–7159. doi: 10.1039/C6TA02218A
Zheng, C., Radhakrishnan, B., Chu, I.-H., Wang, Z., and Ong, S. P. (2017). Effects of transition-metal mixing on Na ordering and kinetics in layered P2 oxides. Phys. Rev. Appl. 7:064003. doi: 10.1103/PhysRevApplied.7.064003
Zheng, L., Li, J., and Obrovac, M. N. (2017). Crystal structures and electrochemical performance of air-stable Na2/3Ni1/3−xCuxMn2/3O2 in sodium cells. Chem. Mater. 29, 1623–1631. doi: 10.1021/acs.chemmater.6b04769
Zheng, L., and Obrovac, M. N. (2017). Investigation of O3-type Na0.9Ni0.45MnxTi0.55−xO2 (0 ≤ x ≤ 0.55) as positive electrode materials for sodium-ion batteries. Electrochim. Acta 233, 284–291. doi: 10.1016/j.electacta.2017.03.033
Zhu, G., Zhang, F., Li, X., Luo, W., Li, L., Zhang, H., et al. (2019). Engineering the distribution of carbon in silicon oxide nanospheres at atomic level for highly stable anodes. Angew Chem Int Ed Engl 58, 1–6. doi: 10.1002/anie.201902083
Zhu, H., Lee, K. T., Hitz, G. T., Han, X., Li, Y., Wan, J., et al. (2014). Free-standing Na2/3Fe1/2Mn1/2O2@graphene film for a sodium-ion battery cathode. ACS Appl. Mater. Interfaces 6, 4242–4247. doi: 10.1021/am405970s
Keywords: layered transition metal oxides, air-stable, cathode, sodium-ion battery, water insertion, H ion exchange
Citation: Zhang Y, Zhang R and Huang Y (2019) Air-Stable NaxTMO2 Cathodes for Sodium Storage. Front. Chem. 7:335. doi: 10.3389/fchem.2019.00335
Received: 17 February 2019; Accepted: 25 April 2019;
Published: 14 May 2019.
Edited by:
Fan Zhang, Fudan University, ChinaReviewed by:
Juchen Guo, University of California, Riverside, United StatesCopyright © 2019 Zhang, Zhang and Huang. This is an open-access article distributed under the terms of the Creative Commons Attribution License (CC BY). The use, distribution or reproduction in other forums is permitted, provided the original author(s) and the copyright owner(s) are credited and that the original publication in this journal is cited, in accordance with accepted academic practice. No use, distribution or reproduction is permitted which does not comply with these terms.
*Correspondence: Renyuan Zhang, cnl6aGFuZ0B0b25namkuZWR1LmNu
Disclaimer: All claims expressed in this article are solely those of the authors and do not necessarily represent those of their affiliated organizations, or those of the publisher, the editors and the reviewers. Any product that may be evaluated in this article or claim that may be made by its manufacturer is not guaranteed or endorsed by the publisher.
Research integrity at Frontiers
Learn more about the work of our research integrity team to safeguard the quality of each article we publish.