- Key Laboratory of Molecular and Nano Probes, Ministry of Education, Shandong Provincial Key Laboratory of Clean Production of Fine Chemicals, College of Chemistry, Chemical Engineering and Materials Science, Collaborative Innovation Centre of Functionalized Probes for Chemical Imaging in Universities of Shandong, Shandong Normal University, Jinan, China
In this work, a micron-sized three-way nitrogen-doped carbon tube covered with MoS2 nanosheets (TNCT@MoS2) was synthesized and applied in photocatalytic water splitting without any sacrificial agents for the first time. The micron-sized three-way nitrogen-doped carbon tube (TNCT) was facilely synthesized by the calcination of commercial sponge. The MoS2 nanosheets were assembled on the carbon tubes by a hydrothermal method. Compared with MoS2, the TNCT@MoS2 heterostructures showed higher H2 evolution rate, which was ascribed to the improved charge separation efficiency and the increased active sites afforded by the TNCT.
Introduction
Photocatalytic water splitting is one of the promising strategies to address the global energy and environmental problems (Hinnemann et al., 2005; Dong et al., 2013; Jiang et al., 2013; Chang et al., 2014; Pan et al., 2016; Wang et al., 2016; Anna et al., 2018; Chen et al., 2018; Zeng et al., 2018). TiO2 is the most investigated material in the semiconductor photocatalysis research field. However, due to the wide bandgap, it is only responsive to UV light, which greatly limits its photocatalytic efficiency (Cui et al., 2013; Shi et al., 2018). In recent years, many visible-light-driven semiconductors have been designed and applied in solar energy conversion research fields (Wang et al., 2014; Pan, 2016; Shao et al., 2017, 2018; Cheng et al., 2018, 2019; Marchal et al., 2018; Wolff et al., 2018; Yang et al., 2019). Molybdenum disulfide (MoS2) is one of the most attractive materials. As a graphene-like hexagonal material with close-packed layered structure, MoS2 has a sandwich architecture, in which the strong covalent bonds among S-Mo-S atoms lies in the layer while the weak van der Waals force exists between the layers (Cheiwchanchamnangij and Lambrecht, 2012). With the variable atomic coordination and the electronic structure, MoS2 exhibits extremely fast carriers mobility (over 200 cm2·V−1·s−1). Furthermore, the band gap is adjustable from 1.19 to 1.80 eV through the variation of layer thickness, nanometer size and ion doping. Hence, MoS2 is an excellent light absorbing material and has high utilization of sunlight. Additionally, the nano-scale molybdenum disulfide has a complicated edge structure with high unsaturation and high reactivity (Wang et al., 2018). In a word, MoS2 has optimal band gap, high reactive spots and fast mobility of charge carriers, which is beneficial for the photocatalysis. However, the photocatalytic efficiency of pure MoS2 is still limited by the fast recombination of photogenerated carriers. The construction of heterostructure of nano-sized MoS2 coupled with other semiconductor or carbon materials has attracted great interest (Xiang et al., 2012; Jia et al., 2013; Guo et al., 2015; Lang et al., 2015; Pan et al., 2016). It was proposed that the hybrids could provide appropriate band structure for water splitting and improve the separation efficiency of photogenerated carriers.
Herein, MoS2 nanosheets assembled on a micron-sized three-way nitrogen-doped carbon tube (TNCT@MoS2) was synthesized and applied in the photocatalytic water splitting for the first time. As illustrated in Scheme 1, the micron-sized three-way nitrogen-doped carbon tube (TNCT) was first prepared by a calcination method. Then, the MoS2 nanosheets were loaded on the TNCT by a hydrothermal method. The as-prepared TNCT@MoS2 composite exhibits much higher photocatalytic activities than pure MoS2, which is ascribed to the improved charge separation and transfer efficiency afforded by the TNCT.
Experimental Section
Materials
Ammonium molybdate tetrahydrate, thiourea, ethanol, and terephthalic acid (TA) were supplied by China National Pharmaceutical Group Chemical Testing Co., Ltd. Melamine sponge is purchased from Zhengzhou Fengtai nanomaterial Co., Ltd. The water used in the experiment is secondary deionized water.
Preparation of TNCT
The commercial melamine sponge was calcined at 450°C for 3 h in vacuum, and the sponge changed from white to black. At this time, the required three-way nitrogen-doped carbon tube (TNCT) was initially formed.
Fabrication of TNCT@MoS2
First, 1.24 g ammonium molybdate tetrahydrate, 2.26 g thiourea and 35 ml deionized water was mixed and stirred at room temperature for 20 min. Then, the mixture and the as-prepared black TNCT were transferred to a 100 mL polytetrafluoroethylene reactor. The reactor was heated in an oven at 220°C for 24 h. After the reaction, the supernatant is poured off, and the sponge block is clamped out. After being mashed, the sponge block was centrifuged and washed with distilled water and ethanol for several times. Then, it was dried in a vacuum drying box at 60°C for 12 h, and a black powder was obtained. Finally, the black powder was calcined at 800°C in N2 atmosphere for 4 h.
Instruments
Scanning Electron Microscope (SEM) equipped with a field-emission gun operated at 5.0 kV was used to characterize the morphology of the as-obtained product. High-Resolution Transmission Electron Microscopy (HRTEM) was taken on JEM-2100F instrument at an accelerating voltage of 200 kV. X–ray diffraction (XRD) analyses carried out on a Bruker D8 Advance Diffractmeter with Cu Kα radiation (1.5418 Å). X-ray photoelectron spectroscopy (XPS) was carried on Thermo Scientific Escalab 250Xi with Al Kα as the excitation source. Photoelectrochemical performance measurements were performed in a standard three-electrode PEC cell, with three-way nitrogen-doped carbon tube@MoS2, saturated calomel electrode, and Pt wire as the working electrode, reference electrode, and counter electrode, respectively. The fluorescence spectrum was carried out with an Edinburgh FLS920 spectrofluorimeter (Edinburgh Instruments Ltd, England) equipped with a xenon lamp. Raman spectra were measured by LabRAM HR800 confocal microscope Raman spectrometer from Horiba Jobin-Yvon, France. The UV/Vis diffuse reflection spectra (DRS) were taken on a Shimadzu UV-2550 spectrophotometer with an integrated sphere attachment and BaSO4 used as the reference sample.
Photocatalytic Activity for Water Splitting
In a typical process, 20 mg of the as-prepared photocatalysts and 10 mL aqueous solution in a 20 mL Quartz bottle sealed with silicone rubber septum. Prior to photocatalysis experiment, the sample solutions were thoroughly deaerated by evacuation and purged with nitrogen for 10 min. Then it was irradiated by a 1,000 W Xenon lamp (the light intensity irradiating the photocatalysts was 0.17 W) at room temperature under constant stirring. The produced gas was analyzed by gas chromatography (FULI 9750, TCD, Nitrogen as the carrier gas, and 5 Å molecular sieve column).
Because there was no O2 detected in this photocatalytic water splitting system, to confirm the photocatalytic water splitting process, the intermediate OH directly photogenerated from water was determined as following (Yang et al., 2009). A fluorescence probe named terephthalic acid (TA) was added into the above-mentioned photocatalytic H2 evolution measurement system to capture OH photogenerated from water, in a result of producing TAOH. Typically, 0.01M TA, 20 mg TNCT@MoS2, and 10 mL water were mixed in a 20 mL Pyrex bottle at ambient temperature and atmospheric pressure, sealed with silicone rubber septum. The sample solutions were thoroughly deaerated by evacuation and argon bubbling for 2 h prior to photocatalysis experiment. Then it was irradiated by a 1,000-W Xe lamp under ambient conditions and magnetic stirring for certain time. The produced solutions containing TAOH were analyzed by fluorescence spectra.
Results and Discussion
As shown in Figures 1a,b, most of these nitrogen-doped carbon tubes have three-way branches, each of which is about 20 microns in length and 3 micron in diameter. The EDS-mapping pictures show that the doped nitrogen elements are uniformly dispersed in the carbon tubes (Figures 1c,d). The SEM image of TNCT@MoS2 heterostructures is shown in Figures 1e–h. It can be seen that the MoS2 nanosheets layer with thickness of 500 nm are uniformly coated on the TNCTs. The MoS2 nanosheets are interconnected with each other, forming the 2D nanosheets networks. Additionally, the EDS mapping pictures show that MoS2 nanosheets are uniformly dispersed on the surface of TNCT (Figures 1j,k).
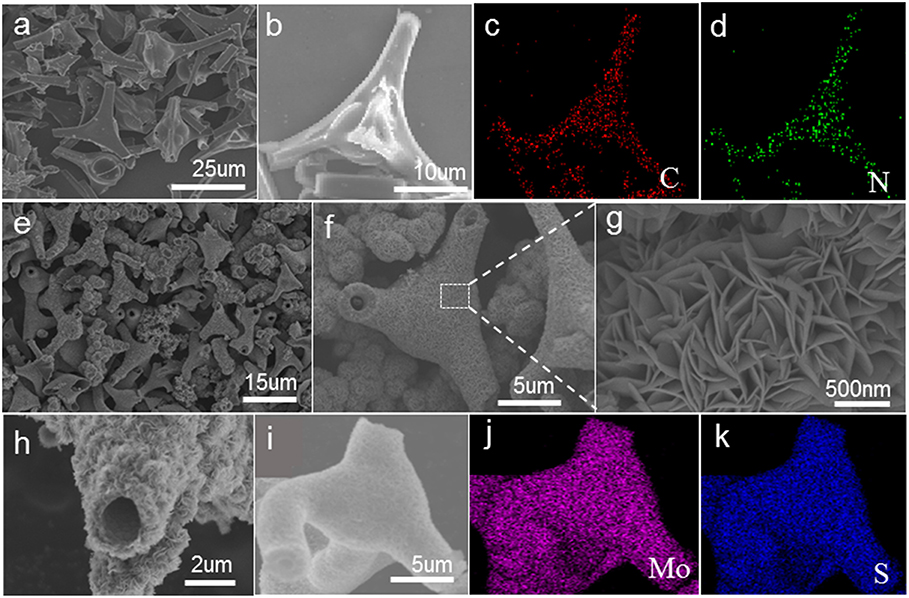
Figure 1. SEM images of TNCT (a,b) and TNCT@MoS2 (e–i). The down two panels shows the element mapping images of C,N of TNCT (c,d) and Mo, S of TNCT@MoS2 (j,k).
XRD peaks of TNCT@MoS2 localized at 2θ values of 13.8, 33.5, 39.8, and 58.8° are ascribed to MoS2 with typical hexagonal phase(JCPDS:00-037-1492, Figure 2a). The (002) crystal plane diffraction peak is stronger than the diffraction peaks on other crystal planes, indicating that MoS2 has a good layered packing structure (Zong et al., 2008). An obvious reflection located at 2θ = 26.5°is ascribed to the TNCT (002) crystal plane (Figure 2a; Tang et al., 2015). The heterogeneous lattice interfaces of MoS2 and TNCT were clearly observed on the HRTEM images (Figures 2b,d). The lattice fringe spacing of 0.65 nm is ascribed to hexagonal phase MoS2 (Choi et al., 2017). The lattice fringes of TNCT are not significant maybe due to the doping of nitrogen atoms, a dimly visible lattice spacing of 0.22 nm was ascribed to the (100) facet of graphite (Baker and Baker, 2010). The strong wide peaks located at 1,379 cm−1 (D-band) in the Raman spectra showed that the presence and partial graphitization of carbon (Supplementary Figure 1; Matthews et al., 1999) in agreement with the results observed from the HRTEM images.
The chemical state of surface species of the samples was further determined by X-ray photoelectron spectroscopy (XPS). The survey XPS spectra showed that Mo, S, N, C, and O elements existed in the surface layer of the as-papered nanostructures (Supplementary Figure 2). Two peaks located at 228.62 and 231.79 eV are attributed to Mo 3d5/2 and Mo 3d3/2, respectively (Figure 3B; Wang et al., 2017). The peak located at 161.8 eV corresponds to the S 2p3/2 and another one located at 162.7 eV was assigned to S 2p1/2 (Figure 3C; Hu et al., 2014). Three peaks were observed in the C 1s binding region peaks centered at 284.2, 285.4 and 289.2 eV, which were ascribed to graphite-C, -C*-C = O and -C* = O groups, respectively (Figure 3D; Christie et al., 1983; Beamson and Briggs, 1993; Witek et al., 1996). Two peaks were observed in the N1s binding region peaks (Figure 3A). The peak centered at 394.5 eV indicated that the doped nitrogen in TNCT was connected with the carbon frame by -N* = N*-C bond (Kudo et al., 1986). It is worth noting that another peak for N1s centered at 396.8 eV was ascribed to Mo(-N* = N-)2-C (George and Kwarcinski, 1995), which indicated that the MoS2 could closely bond with TNCT by the surface -N=N- group.
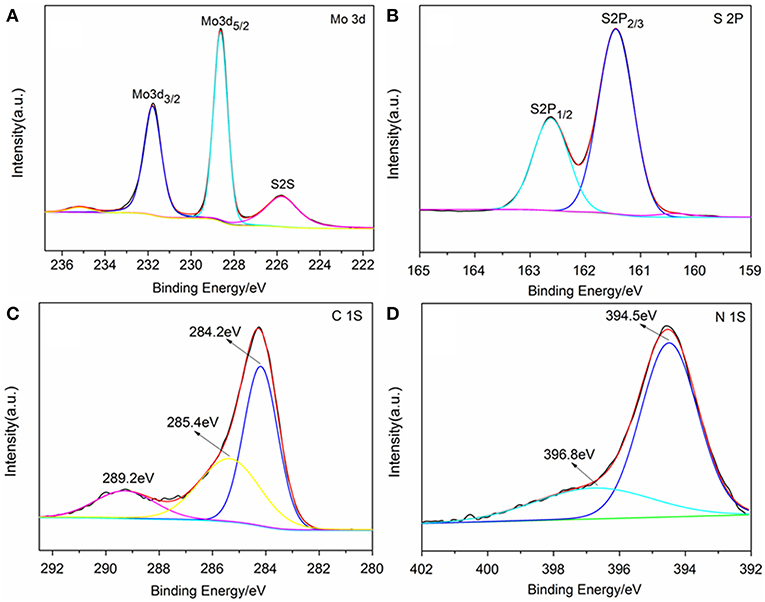
Figure 3. XPS spectrum of TNCT@MoS2. Mo 3d spectrum (A), S 2p spectrum (B), C 1s spectrum (C), and N 1S spectrum (D).
The UV-Vis-IR absorbance spectrum shows that TNCT@MoS2 exhibits higher and broader spectral absorption including IR light than that of TNCT and MoS2 (Figure 4A). As shown in Figure 4B, TNCT@MoS2 obviously shows higher photocurrent density than that of MoS2. It indicates that TNCT@ MoS2 maybe has better photocatalytic activity.
The photocatalytic activity of the as-prepared catalyst was investigated for the photocatalytic water splitting process without addition of any sacrificial agents, which was performed by a 1,000 W xenon lamp source. It was found that the catalyst showed almost the same photocatalytic activity in the wide pH range of 5-11 and pure water (Figure 5A). The optimal hydrogen production rate of TNCT@MoS2 was 120 μmol/g·h, which is about 9 times faster than that of pure MoS2 (Figure 5B).
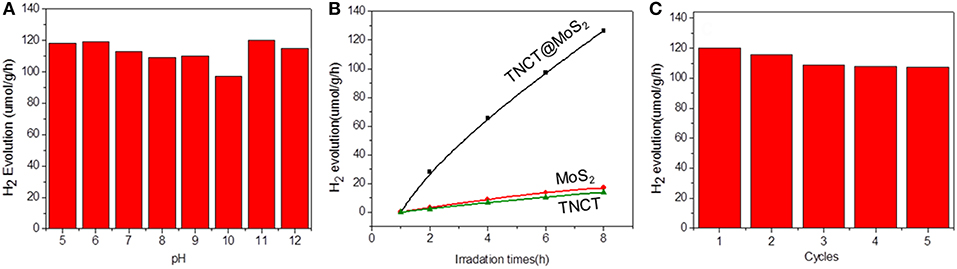
Figure 5. Photocatalytic hydrogen production activity at different pH values (A), hydrogen production data for different samples (B), and recycling performance for TNCT@MoS2 (C).
For a typical photocatalytic water splitting process, both H2 and O2 are usually produced from water. However, in this work, whereas this system readily produces hydrogen under illumination, the simultaneous liberation of oxygen is not observed. This phenomenon has been observed in much water splitting systems (Duonghong and Grätzel, 1984; Liu et al., 2012; Zhang et al., 2014). It is because the produced O2 or intermediate oxygen species such as OH are readily absorbed by the metal elements such as Mo and W to form steady peroxide complexes, in a result of inhibiting the release of O2 (Cui et al., 2015). Herein, although there was no O2 determined in this photocatalytic water splitting system, intermediate OH directly photogenerated from water was detected by the fluorescence probe TA (Yang et al., 2009). The fluorescence spectra of TAOH, which is produced through the oxidation of TA by OH, exhibited a peak at 426 nm, indicating the presence of OH (Supplementary Figure 3). Although the surface of molybdenum sulfide suffer from the produced intermediate active oxygen, the XRD (Figure 2a) and Raman spectroscopy (Supplementary Figure 4) showed that the crystal structure of the material did not change fundamentally and maintain the same photoactivity for five photocatalytic reaction runs (Figure 5C).
The excellent photocatalytic performance of TNCT@MoS2 is ascribed to the effective separation and transfer of photogenerated carriers. Herein, it was verified by the photoluminescence spectroscopy and photoelectrochemical performance measurements. The photoluminescence (PL) of semiconductors was caused by recombination of photoinduced electrons and holes. If the recombination was suppressed, the PL emission of semiconductors would be quenched.As shown in Supplementary Figure 5, after being combined with TNCT, the PL intensity of MoS2 emission peak obviously quenched. It indicated that the photoinduced charge separation efficiency of TNCT@MoS2 would improve. The photogenerated carriers' transfer capacity of the as prepared materials was confirmed by electrochemical impedance tests. As shown in Figure 6, TNCT, MoS2, and TNCT@MoS2 all showed classical semicircular Nyquist diagram. The arc radius of TNCT@MoS2 was obviously smaller than that of TNCT and MoS2, which indicated that the photogenerated charges in TNCT@MoS2 would suffer less resistance. It is ascribed to the fast charge transfer from MoS2 nanosheets to carbon tube under full-light irradiation. The formed Mo(-N* = N-)2-C bonding connection between MoS2 and TNCT maybe acts as carrier transfer bridge (Figure 3D), which will improve the photogenerated carriers separation and transfer efficiency of TNCT@MoS2. The detailed mechanism and remained questions would be studied in our future work.
Conclusions
In summary, a micron-sized three-way nitrogen-doped carbon tube covered with MoS2 nanosheets was synthesized and applied in the photocatalytic water splitting without any sacrificial agents for the first time. The micron-sized three-way nitrogen-doped carbon tube is facilely synthesized by the calcination of sponge at 450°C in vacuum. And then the MoS2 nanosheets are deposited on the three-way nitrogen-doped carbon tubes by a simple hydrothermal method. Compared with MoS2, the TNCT@MoS2 heterostructures showed higher H2 evolution rate, which may be ascribed to the improved charge separation and transfer efficiency caused by the three-way nitrogen-doped carbon tube. This work may provide a new design idea for the design of low-cost and efficient photocatalysts.
Author Contributions
GC and BT conceived and designed the experiments. YuZ, YL, PC, HC, and YF performed the experiments. WG, XS, and YiZ analyzed the data. GC and YuZ co-wrote the paper.
Funding
This work was supported by the National Natural Science Foundation of China (21535004, 91753111, 21575082, 21775092, 21507074) and the Key Research and Development Program of Shandong Province (2018YFJH0502), Development plan of science and technology for Shandong Province of China (2013GGX10706), Shandong Province Natural Science Foundation (ZR2015BM023, ZR2018JL008), and the Project of Shandong Province Higher Educational Science and Technology Program (J13LD06).
Conflict of Interest Statement
The authors declare that the research was conducted in the absence of any commercial or financial relationships that could be construed as a potential conflict of interest.
Supplementary Material
The Supplementary Material for this article can be found online at: https://www.frontiersin.org/articles/10.3389/fchem.2019.00325/full#supplementary-material
References
Anna, M.-M., Lluís, S., Miquel, T., Pol, S., Jordi, L., and Anna, R. (2018). Fast and Simple Microwave synthesis of TiO2/Au nanoparticles for gas-phase photocatalytic hydrogen generation. Front Chem. 6:110. doi: 10.3389/fchem.2018.00110
Baker, S. N., and Baker, G. A. (2010). Luminescent carbon nanodots: emergent nanolights. Angew. Chem. Int. Ed. 49, 6726–6744. doi: 10.1002/chin.201103270
Beamson, G., and Briggs, D. (1993). High resolution XPS of organic polymers: the scienta ESCA300 database. J. Chem. Educ. 70:A25 doi: 10.1021/ed070pA25.5
Chang, K., Mei, Z. W., Wang, T., Kang, Q., Ouyang, S. X., and Xe, J. H. (2014). MoS2/graphene cocatalyst for efficient photocatalytic H2 evolution under visible light irradiation. ACS Nano. 8, 7078–7087. doi: 10.1021/nn5019945
Cheiwchanchamnangij, T., and Lambrecht, W. R. L. (2012). Quasiparticle band structure calculation of monolayer, bilayer, and bulk MoS2. Phys. Rev. B. 85:205302. doi: 10.1103/PhysRevB.85.205302
Chen, X., Zhang, W. J., Tang, J. T., Pan, C. Y., and Yu, G. P. (2018). Porous organic polymers: an emerged platform for photocatalytic water splitting. Front Chem. 6:592. doi: 10.3389/fchem.2018.00592
Cheng, J. S., Zhao, H., Kangle, L., Wu, X. F., Li, Q., and Li, Y. H., et al. (2018). Drastic promoting the visible photoreactivity of layered carbon nitride by polymerization of dicyandiamide at high pressure. Appl. Cataly. B Environ. 232, 330–339. doi: 10.1016/j.apcatb.2018.03.066
Cheng, J. S., Zhao, H., Li, Q., Li, X. F., Fang, S., and Wu, X. F., et al. (2019). Fabrication of high photoreactive carbon nitride nanosheets by polymerization of amidinourea for hydrogen production. Appl. Cataly. B Environ. 245, 197–206. doi: 10.1016/j.apcatb.2018.12.044
Choi, J., Reddy, D. A., Han, N. S., Jeong, S., Hong, S., and Kumar, D. P., et al. (2017). Modulation of charge carrier pathways in CdS nanospheres by integrating MoS2 and Ni2P for improved migration and separation toward enhanced photocatalytic hydrogen evolution. Catal. Sci. Technol. 7, 641–649. doi: 10.1039/c6cy02145j
Christie, A. B., Lee, J., Sutherland, I., and Walls, J. M. (1983). An XPS study of ion-induced compositional changes with group II and group IV compounds. Appl. Surf. Sci. 15, 224–237. doi: 10.1016/0378-5963(83)90018-1
Cui, G.-W., Wang, W.-L., Ma, M.-Y., Zhang, M., Xia, X.-Y., and Han, F.-Y., et al. (2013). Rational design of carbon and TiO2 assembly materials: covered or strewn, which is better for photocatalysis? Chem. Commun. 49, 6415–6417. doi: 10.1039/c3cc42500b
Cui, G. W., Wang, W., Ma, M. Y., Xie, J. F., Shi, X. F., and Deng, N., et al. (2015). IR-driven photocatalytic water splitting with WO2-NaxWO3 hybrid conductor material. Nano Lett. 15, 7199–7203. doi: 10.1021/acs.nanolett.5b01581
Dong, S., Sun, J., Li, Y., Yu, C., Li, Y., and Sun, J. (2013). ZnSnO3 hollow nanospheres/reduced graphene oxide nanocomposites as high-performance photocatalysts for degradation of metronidazole. Appl. Catal. B 144, 386–393. doi: 10.1016/j.apcatb.2013.07.043
Duonghong, D., and Grätzel, M. (1984). Colloidal TiO2 particles as oxygen carriers in photochemical water cleavage systems. J. Chem. Soc. Chem. Commun. 1597–1599. doi: 10.1039/C39840001597
George, T. A., and Kwarcinski, M. E. (1995). PreparAtion and properties of diazenido, hydrazido(2-), and hydrido-hydrazido(2-) complexes of tungsten. x-rayphotoelectron spectroscopy of dinitrogen and hydrazido(2-) complexes of molybdenum and tungsten. J. Coord. Chem. 35, 349–357. doi: 10.1080/00958979508024047
Guo, X., Cao, G.-L., Ding, F., Li, X. Y., Zhen, S. Y., and Xue, Y.-F., et al. (2015). A bulky and flexible electrocatalyst for efficient hydrogen evolution based on the growth of MoS2 nanoparticles on carbon nanofiber foam. J. Mater. Chem. A. 3, 5041–5046. doi: 10.1039/c5ta00087d
Hinnemann, B., Moses, P. G., Bonde, J., Jørgensen, K. P., Nielsen, J. H., and Horch, S., et al. (2005). Biomimetic hydrogen evolution: MoS2 nanoparticles as catalyst for hydrogen evolution. J. Am. Chem. Soc. 127, 5308–5309. doi: 10.1021/ja0504690
Hu, S., Chen, W., Zhou, J., Yin, Y., Uchaker, E., and Zhang, Q. F., et al. (2014). Preparation of carbon coated MoS2 flower-like nanostructure with self-assembled nanosheets as high-performance lithium-ion battery anodes. J. Mater. Chem. A. 2, 7862–7872. doi: 10.1039/c4ta01247j
Jia, T. T., Kolpin, A., Ma, C. S., Chan, R. C.-T., Kwok, W. M., and Tsang, S. C. E. (2013). A graphene dispersed CdS–MoS2 nanocrystal ensemble for cooperative photocatalytic hydrogen production from water. Chem. Commun. 50, 1185–1188. doi: 10.1039/c3cc47301e
Jiang, Y.-R., Lee, W., Chen, K.-T., Wang, M.-C., Chang, K.-H., and Chen, C.-C. (2013). Hydrothermal synthesis of b-ZnMoO4 crystals and their photocatalytic degradation of Victoria Blue R and phenol. J. Taiwan Inst. Chem. Eng. 45, 207–218. doi: 10.1016/j.jtice.2013.05.007
Kudo, Y., Yoshida, N., Fujimoto, M., Tanaka, K., and Toyoshima, I. (1986). Acid-dissociation behavior of para-hydroxyl group in the N,N,O-terdentate ligand, 4-(4-methyl-2-pyridylazo)resorcinol, coordinated to a transition metal ion. Bull. Chem. Soc. Jpn. 59, 1481–1486. doi: 10.1246/bcsj.59.1481
Lang, D., Shen, T. T., and Xiang, Q. J. (2015). Roles of MoS2 and graphene as cocatalysts in the enhanced visible-Light photocatalytic H2 production activity of multiarmed CdS nanorods. Chemcatchem 7, 943–951. doi: 10.1002/cctc.201403062
Liu, J. H., Zhang, Y. W., Lu, L. H., Wu, G., and Wei, C. (2012). Self-regenerated solar-driven photocatalytic water-splitting by urea derived graphitic carbon nitride with platinum nanoparticles. Chem. Commun. 48, 8826–8828. doi: 10.1039/C2CC33644H
Marchal, C., Cottineau, T., Méndez-Medrano, M. G., Colbeau-Justin, C., Caps, V., and Keller, V. (2018), Au/TiO2-gC3N4 nanocomposites for enhanced photocatalytic H2 production from water under visible light irradiation with very low quantities of sacrificial agents. Adv. Energy Mater. 8:1702142. doi: 10.1002/aenm.201702142
Matthews, M. J., Pimenta, M. A., and Dresselhaus, G., et al. (1999). Origin of dispersive effects of the raman d band in carbon materials. Physical Review B 59:R6585. doi: 10.1103/PhysRevB.59.R6585
Pan, H. (2016). Principles on design and fabrication of nanomaterials as photocatalysts for water-splitting. Renew. Sustain. Energy Rev. 57, 584–601. doi: 10.1016/j.rser.2015.12.117
Pan, Y., Lin, Y., Liu, Y. Q., and Liu, C. G. (2016). A novel CoP/MoS2-CNTs hybrid catalyst with Ptlike activity for hydrogen evolution. Catal. Sci. Technol. 6, 1611–1615. doi: 10.1039/c5cy02299a
Shao, M. M., Shao, Y. F., Chai, J. W., Qu, Y. J., Yang, M. Y., and Wang, Z. L., et al. (2017). Synergistic effect of 2D Ti2C and g-C3N4 for efficient photocatalytic hydrogen production. J. Mater. Chem. A. 5, 16748–16756. doi: 10.1039/c7ta04122e
Shao, M. M., Shao, Y. F., Ding, S. J., Wang, J. W., Xu, J. C., and Qu, Y. J., et al. (2018). Vanadium disulfide decorated graphitic carbon nitride for super-efficient solar-driven hydrogen evolution. Appl. Cataly. B Environ. 237, 295–301. doi: 10.1016/j.apcatb.2018.05.084
Shi, T., Duan, Y., Lv, K., Hu, Z., Li, Q., and Li, M., et al. (2018). Photocatalytic oxidation of acetone over high thermally stable TiO2 nanosheets with exposed (001) facets. Front Chem. 6:175. doi: 10.3389/fchem.2018.00175
Tang, J., Salunkhe, R. R., Liu, J., Torad, N. L., Imura, M., and Furukawa, S., et al. (2015). Thermal conversion of core–Shell metal–organic frameworks: a new method for selectively functionalized nanoporous hybrid carbon. J. Am. Chem. Soc. 137, 1572–1580. doi: 10.1021/ja511539a
Wang, J. Z., Dong, S. Y., Guo, T., Jin, J., and Sun, J. H. (2017). pH-dictated synthesis of novel flower-like MoS2 with augmented natural sunlight photocatalytic activity. Mater. Lett. 191, 22–25. doi: 10.1016/j.matlet.2016.12.090
Wang, L. J., Fan, J., Cao, Z., Zheng, Y., Yao, Z., and Shao, G., et al. (2014). Fabrication of predominantly Mn4+-doped TiO2 nanoparticles under equilibrium conditions and their application as visible-light photocatalyts. Chem Asian. J. 9, 1904–1912. doi: 10.1002/asia.201402114
Wang, L. J., Zhang, X., Gao, H. Q., Hu, J. H., Mao, J., and Liang, C. H., et al. (2016). 3D CuO network supported TiO2 nanosheets with applications for energy storage and water splitting. Sci. Adv. Mater. 8, 1256–1262. doi: 10.1166/sam.2016.2714
Wang, Q. S., Wen, Y., Cai, K. M., Cheng, R. Q., Yin, L., and Zhang, Y., et al. (2018). Nonvolatile infrared memory in MoS2/PbS van der Waals heterostructures. Sci. Adv. 4:eaap7916. doi: 10.1126/sciadv.aap7916
Witek, G., Noeske, M., Mestl, G., Shaikhutdinov, S., and Behm, R. J. (1996). Interaction of platinum colloids with single crystalline oxide and graphite substrates: a combined AFM, STM and XPS study. Catal. Lett. 37, 35–39. doi: 10.1007/BF00813516
Wolff, C. M., Frischmann, P. D., Schulze, M., Bohn, B. J., Wein, B., and Livadas, P., et al. (2018). All-in-one visible-light-driven water splitting by combining nanoparticulate and molecular co-catalysts on CdS nanorods. Nat. Energy. 3, 862–869. doi: 10.1038/s41560-018-0229-6
Xiang, Q. J., Yu, J. G., and Jaroniec, M. (2012). Synergetic effect of MoS2 and graphene as cocatalysts for enhanced photocatalytic H2 production activity of TiO2 nanoparticles. J. Am. Chem. Soc. 134,6575–6578. doi: 10.1021/ja302846n
Yang, C., Li, Q., Xia, Y., Lv, K., and Li, M. (2019). Enhanced visible-light photocatalytic CO2 reduction performance of ZnIn2S4 microspheres by using CeO2 as cocatalyst. Appl. Surf. Sci. 464, 388–395. doi: 10.1016/j.apsusc.2018.09.099
Yang, H. G., Liu, G., Qiao, S. Z., Sun, C. H., Jin, Y. G., and Smith, S. C., et al. (2009). Solvothermal synthesis and photoreactivity of anatase TiO2 nanosheets with dominant {001} facets. J. Am. Chem. Soc. 131, 4078–4083. doi: 10.1021/ja808790p
Zeng, D., Xu, W., Ong, W.-J., Xu, J., Ren, H., and Chen, Y., et al. (2018). Toward noble-metal-free visible-light-driven photocatalytic hydrogen evolution: monodisperse sub−15 nm Ni2P nanoparticles anchored on porous g-C3N4 nanosheets to engineer 0D-2D heterojunction interfaces. Appl. Catal. B. 221, 47–55. doi: 10.1016/j.apcatb.2017.08.041
Zhang, M., Respinis, M. D., and Frei, H. (2014). Time-resolved observations of water oxidation intermediates on a cobalt oxide nanoparticle catalyst. Nat. Chem. 6, 362–367. doi: 10.1038/nchem.1874
Keywords: photocatalysis, MoS2, three-way nitrogen-doped carbon tubes, hydrogen evolution, water splitting
Citation: Zhang Y, Liu Y, Gao W, Chen P, Cui H, Fan Y, Shi X, Zhao Y, Cui G and Tang B (2019) MoS2 Nanosheets Assembled on Three-Way Nitrogen-Doped Carbon Tubes for Photocatalytic Water Splitting. Front. Chem. 7:325. doi: 10.3389/fchem.2019.00325
Received: 24 January 2019; Accepted: 23 April 2019;
Published: 17 May 2019.
Edited by:
Wee-Jun Ong, Xiamen University, Malaysia, MalaysiaReviewed by:
Kangle Lv, South-Central University for Nationalities, ChinaHui Pan, University of Macau, China
Junhua Hu, Zhengzhou University, China
Copyright © 2019 Zhang, Liu, Gao, Chen, Cui, Fan, Shi, Zhao, Cui and Tang. This is an open-access article distributed under the terms of the Creative Commons Attribution License (CC BY). The use, distribution or reproduction in other forums is permitted, provided the original author(s) and the copyright owner(s) are credited and that the original publication in this journal is cited, in accordance with accepted academic practice. No use, distribution or reproduction is permitted which does not comply with these terms.
*Correspondence: Guanwei Cui, Y3VpZ3VhbndlaTc3QDE2My5jb20=
Bo Tang, dGFuZ2JAc2RudS5lZHUuY24=
†These authors have contributed equally to this work