- 1Department of Pharmaceutical Sciences (DISFARM), University of Milan, Milan, Italy
- 2Department of Chemistry, University of Padova, Padova, Italy
- 3CNRS UMR 7025, Génie Enzymatique et Cellulaire, Centre de Recherche de Royallieu, Compiègne, France
- 4Génie Enzymatique et Cellulaire, Centre de Recherche de Royallieu, Sorbonne Universités, Université de Technologie de Compiègne, Compiègne, France
α,β2,3-Disteroisomeric foldamers of general formula Boc(S-Ala-β-2R,3R-Fpg)nOMe or Boc(S-Ala-β-2S,3S-Fpg)nOMe were prepared from both enantiomers of syn H-2-(2-F-Phe)-h-PheGly-OH (named β-Fpg) and S-alanine. Our peptides show two appealing features for biomedical applications: the presence of fluorine, attractive for non-covalent interactions, and aryl groups, crucial for π-stacking. A conformational study was performed, using IR, NMR and computational studies of diastereoisomeric tetra- and hexapeptides containing the β2,3-amino acid in the R,R- and S,S-stereochemistry, respectively. We found that the stability of peptide conformation is dependent on the stereochemistry of the β-amino acid. Combining S-Ala with β-2R,3R-Fpg, a stable extended β-strand conformation was obtained. Furthermore, β-2R,3R-Fpg containing hexapeptide self-assembles to form antiparallel β-sheet structure stabilized by intermolecular H-bonds and π,π-interactions. These features make peptides containing the β2,3-fluoro amino acid very appealing for the development of bioactive proteolytically stable foldameric β-sheets as modulators of protein-protein interaction (PPI).
Introduction
Amino acids are small molecules able to induce high molecular complexity, promoting discrete three-dimensional folded structures in peptides. α-Amino acid-based peptides show some drawbacks such as the low stability to proteases, and, in the case of short peptides, the lack of a stable secondary structure. The use of oligomers with unnatural backbones is a powerful strategy to overcome those issues, while maintaining the desired fragment structure.
In particular, β-peptide foldamers (Seebach et al., 1996, 2004; Gellman, 1998) have been extensively studied in the last two decades. These molecules can adopt specific compact conformations despite the increased conformational space due to the additional methylene group of the β-amino acid. Furthermore, their substitution pattern can influence the conformation of β-peptides, as reported in several experimental (Cheng et al., 2001; Seebach et al., 2006; Seebach and Gardiner, 2008; Pilsl and Reiser, 2011; Vasudev et al., 2011; Berlicki et al., 2012; Johnson and Gellman, 2013; Basuroy et al., 2014; Lee et al., 2014; Wang and Schepartz, 2016; Bucci et al., 2017a) and molecular modeling studies (Wu et al., 2008; Zhu et al., 2010; Baldauf and Hofmann, 2012). An even larger pool of secondary structure motifs could be obtained, by the combination of α- and both acyclic and cyclic β-amino acids (αβ; ααβ; αββ; etc). Inspired by the diversity of folded structures and functions manifested by peptides and proteins, β-peptide mimics and foldamers have been thus exploited for different applications ranging from biomedicine (Horne, 2011; Cabrele et al., 2014; Checco and Gellman, 2016) to material science (Gopalan et al., 2015; Clerici et al., 2016; Del Borgo et al., 2017).
Only few examples are reported related to α,β-repeating sequences containing acyclic amino acids (Sharma et al., 2005; Srinivasulu et al., 2006; Angelici et al., 2007; Balamurugan and Muraleedharan, 2012; Basuroy et al., 2014), the majority of them giving helix constructs.
Our interest toward the preparation of non-natural amino acids (Pellegrino et al., 2008; Penso et al., 2012; Ruffoni et al., 2015) and their use for peptidomimetic synthesis for different applications (Pellegrino et al., 2015, 2016; Ruffoni et al., 2016; Bucci et al., 2018, 2019; Tonali et al., 2018) is well-documented. Recently, our research group reported on a diastreoselective synthesis of a new class of β-amino acids, syn-S*, S*-β2,3-diarylamino acids, differently substituted on the aromatic ring (Bonetti et al., 2014).
Due to the lack of information related to α,β2,3-amino acids repeating sequences, here we report on the preparation and conformational studies of α,β2,3-peptides, containing syn H-2-(2-F-Phe)-h-PheGly-OH, named β-Fpg, and helicogenic S-alanine (Figure 1). This scaffold shows two features that can be appealing for biomedical applications: the presence of a fluorine, useful for non-covalent interactions, and two aryl groups, crucial for π-stacking and hydrophobic interactions. Taking advantage of the use of fluorine-substituted β-2S,3S-Fpg (Figure 1), in combination with S-Ala or S-Arg-S-Ala, we recently prepared short peptides able to generate proteolytically stable nanotubes (Bonetti et al., 2015) and spherical aggregates (Bucci et al., 2017b) as drug delivery systems.
Here we report on a conformational study on diastereoisomeric tetra- and hexapeptides α,β2,3-foldamers of general formula Boc(S-Ala-β-2R,3R-Fpg)nOMe and Boc(S-Ala-β-2S,3S-Fpg)nOMe (Figure 1).
Our results showed that the stability of peptide conformation is dependent on the stereochemistry of the β-amino acid. Combining S-Ala with β-2R,3R-Fpg, a stable extended β-strand conformation was obtained. Moreover, it was found that hexapeptide 11 (Figure 1) self-assembles to form antiparallel β-sheet structure stabilized by intermolecular H-bonds and π,π-interactions. This result is important since, to the best of our knowledge, no examples of extended α,β2,3-foldamers are reported. Furthermore, there is a need of the development of bioactive proteolytically stable foldameric β-sheets that might be useful as modulators of protein-protein interactions (PPI) (Hegedüs et al., 2016). The design of this secondary structure is indeed not trivial, although β-sheet interfaces frequently occurred in PPI.
Materials and Methods
Chemistry
Experimental procedures, compound characterization data for newly synthesized peptides (3-5 and 7-12; for 1H, 13C, and 19F NMR spectra see Figures S10–S39) are reported in the Supplementary Material. Dipeptide 1, 2 (Bonetti et al., 2015) and 6 (Bucci et al., 2017b) are known compounds.
Melting points were determined with a Stuart Scientific melting point apparatus in open capillary tubes and are uncorrected. Chemicals were purchased from Sigma Aldrich and were used without further purification. ESI MS were recorded on a LCQ Advantage spectrometer from Thermo Finningan and a LCQ Fleet spectrometer from Thermo Scientific. The FT-IR absorption spectra were recorded with a Perkin-Elmer 1720X spectrophotometer, nitrogen flushed, equipped with a sample-shuttle device, at 2 cm−1 nominal resolution, averaging 100 scans. Solvent (base-line) spectra were recorded under the same conditions. Cells with path lengths of 1.0 and 10 mm (with CaF2 windows) were used. Spectrograde CDCl3 (99.8% D) was purchased from Fluka. The NMR spectroscopic experiments were carried out either on a Varian MERCURY 200 MHz (200 and 50 MHz for 1H and 13C, respectively), Varian MERCURY 300 MHz (300, 75, 282 MHz for 1H, 13C, and 19F, respectively), or Bruker Avance I 500 MHz spectrometers (500 and 125 MHz for 1H and 13C, respectively). Chemical shifts (δ) are given in ppm relative to the CHCl3 internal standard, and the coupling constants J are reported in Hertz (Hz). Optical rotations were measured on a Perkin–Elmer 343 polarimeter at 20°C (concentration in g/100 mL).
Computational Methods
The non-natural β-amino acids, capped respectively with an acetyl (Ac) and an OMe group at the N- and C-termini, were designed using MOE (Molecular Operating Environment, 2013) and submitted to a “low mode” conformational search (MMFF94x force field, Born solvation, iteration limit = 40 000, MM iteration limit = 2,500, rejection limit = 500). The two lowest energy conformations having ϕ, θ, and ψ dihedrals corresponding to two different secondary structures, namely the extended one and a β-turn, were selected to derive partial charges with the R.E.D.III.52 software (Dupradeau et al., 2010). Each geometry was optimized at the HF/6-31G(d) level, and two different spatial orientations were used to derive orientation- and conformation-independent RESP-A1 charges.
The tetra- (m7 and m8) and hexapeptides (m11 and m12) of general formula 8 were built with the tleap module of Amber14 (Case et al., 2014) by imposing an extended conformation (ϕ = ψ = ω = θ = 180°), and solvated with an octahedral box of CHCl3. All the systems were consequently submitted to a preliminary equilibration with the pmemd module of Amber14 package, using the ff14SB (Maier et al., 2015) force field. In detail, the systems were relaxed by minimizing hydrogens and solvent (2,000 cycles of steepest descent and 5,000 cycles of conjugated gradient). The solvent box was equilibrated at 300 K by 100 ps of NVT and 100 ps of NPT simulation using a Langevin thermostat with a collision frequency of 2.0 ps−1. Successively, a minimization of side chains and CHCl3 with restraints on backbone atoms of 25 kcal/mol and a total minimization (2,500 cycles of steepest descent and 5,000 cycles of conjugated gradient) were performed. The systems were then heated up to 300 K in 6 steps of 5 ps each (ΔT = 50 K), where backbone restraints were reduced from 10.0 to 5 kcal/mol. Full equilibration was performed in the NVT ensemble (100 ps, backbone restraints = 5.0 kcal/mol) and in the NPT ensemble (1 step of 200 ps, backbone restraints = 5 kcal/mol; 3 steps of 100 ps each, reducing the backbone restraints from 5.0 to 1.0 kcal/mol, and 1 step 1 ns with 1.0 kcal/mol of nd backbone restraints). Finally, unrestrained production runs were run at 300 K for 15 ns. An electrostatic cutoff of 8.0 Å was applied to all the calculations. The equilibrated geometries and the average dihedral energies and potential obtained through the accurate systems equilibrations were used as starting point for accelerated MD (aMD) simulations, where the α value was set to 0.2 and 0.16, for the dihedral and potential factors, respectively.
The aMD simulations of each peptide were run until convergence, for a total of 500 ns for the tetrapeptides m7 and m8, and 800 ns for hexapeptides m11 and m12, respectively. The root mean square displacement of backbone heavy atoms from the extended conformation was used as a metric of convergence (Figure S1). All the analyses were conducted on the last 200 ns of trajectory. Cluster analyses were performed with the cpptraj module of Amber14 by sampling one of every 8 frames, using the average-linkage algorithm and requesting ten clusters; the pairwise mass-weighted RMSD on backbone Cα atoms was used as a metric. H-bonds were computed with cpptraj by setting a donor-acceptor distance threshold of 4.0 Å and an angle cutoff of 110°. Only H-bonds with an occupancy >20% were considered. Cpptraj was used also for computing the radius of gyration of investigated peptides.
Results and Discussion
Synthesis
Dipeptides 1 and 2 were efficiently synthesized in gram scale starting from a racemic mixture of amino acid β-Fpg, according to a known procedure (Bonetti et al., 2015). Pure dipeptides were used for the preparation of α,β2,3-tetra- and hexa-peptide sequences (Scheme 1).
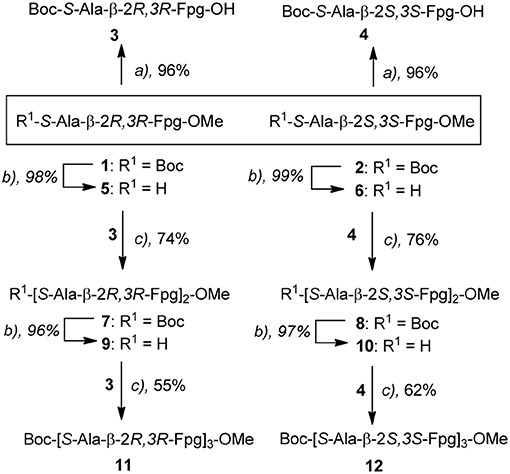
Scheme 1. a) i) 1 M HCl, 80°C, 12 h; ii) (Boc2O, DCM, TEA, 25°C, 12 h; b) TFA, CH2Cl2, 0°C, 1 h; c) EDC (1.1 equiv.)/EtCN-oxime(1.1 equiv.), CH2Cl2, 25°C, 3 h.
The deprotection of the carboxyl group of dipeptides 1 and 2 with LiOH or KOH gave a partial epimerization of the benzylic-Cα position of the β-amino acid, even at low temperature. To avoid this problem, we moved to the deprotection of both C- and N-termini operating in 1 M HCl (80 °C, 12 h, 99%), followed by Boc-protection of N-terminus (Boc2O, DCM, TEA, 25 °C, 12 h, 96%). Dipeptide 3 and 4 were obtained in excellent overall yields. The deprotection of nitrogen atom was performed in standard conditions (TFA in CH2Cl2, 0°C, 1 h) affording dipeptides 5 and 6 from 1 and 2 (99%), respectively. Good yields in the coupling reactions between 3 with 5 and 4 with 6 affording 7 (74%) and 8 (76%), respectively, were achieved using EDC (1.1 equiv.)/EtCN-oxime/(1.1 equiv.) in CH2Cl2 (25°C, 3h).
Using similar N-deprotection/condensation protocols, hexapeptides 11 and 12 were prepared, via esters 9 and 10.
IR Analysis
In our FTIR absorption investigation we focused on the N-H stretching (amide A) region (3,470–3,240 cm−1). Absorptions in this region are highly dependent on H-bond formation and, consequently, they are conformationally informative. To get insights into the modes of folding and self-association we chose as solvent CDCl3. It has a relatively low polarity and it is known to support ordered secondary structures in peptides.
As the peptide chain is elongated, the relative intensity of the N-H stretching band at about 3,330 cm−1 markedly increases as compared to that of the band at about 3,430 cm−1 (Figure S9). The first band is assigned to H-bonded NH groups, while the second is due to free (solvated) NH groups (Palumbo et al., 1976; Toniolo et al., 1985).
In the absence of aggregation, this behavior can be ascribed to the formation of ordered secondary structures, stabilized by intramolecular H-bonds. From our analysis we conclude that the chirality of the two stereogenic centers of the fluoro amino acid remarkably affects the peptide 3D-structure. In particular, the maximum of the bonded N-H band is located at 3,320 cm−1 for peptide 12, but at higher energy, 3,330 cm−1, for peptide 11 (Figure 2). This latter observation implies that the H-bonds in the 11 hexapeptide are weaker. Indeed, an inspection to the behavior of the two hexapeptides at two concentrations (0.1 and 1 mM) is highly informative (Figure 2). For peptide 12 the relative intensities of the bands centered at 3,430 cm−1 (free NHs) and at 3,320 cm−1 (H-bonded NHs) do not significantly change upon diluting 10 times (Figure 2B). Therefore, we assume that the H-bonded NHs originate mainly from intramolecular interactions in 12. Conversely, a clear dilution effect is observed for 11 (Figure 2A) highlighting an important contribution from intermolecular H-bonds. The onset of a β-sheet conformation can thus be hypothesized for 11 based on this FTIR absorption analysis.
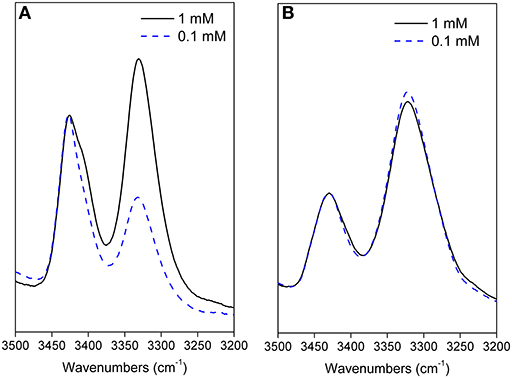
Figure 2. Amide A portion of the FTIR spectra acquired for 11 (A) and 12 (B) in CDCl3 solution at 0.1 and 1 mM peptide concentrations (cell path lengths of 10 and 1 mm were used, respectively).
In conclusion, this IR absorption analysis indicates that the longer oligomers adopt secondary structures strongly dependent on the chirality of the fluoro-β2,3-diarylamino acid.
NMR Characterization
All synthesized peptides were fully characterized by NMR (1H, 13C, COSY, TOCSY, HMBC, HMQC, NOESY, ROESY) in CDCl3. In many cases broad CH and NH signals were detected, thus preventing a clear information on spatial proximities. Detailed data are reported in the Supporting Material (7: Table TS1; 8: Table TS2; 11: Tables TS3, TS4; 12: Table TS5). Finally, fluorine NMR was performed for all peptides. In all cases, fluorine atoms resonate in δ −118 - −117 region, except for fluorine of Arβ2 and Arβ4 of 11 that resonate at lower fields (δ −116.1, −114.8, broad signals; not assigned).
As reported in the literature (Cheng et al., 2001; Balamurugan and Muraleedharan, 2012; March et al., 2012), J2,3 and JNH−CHβ values help to predict the secondary structure of peptides containing β-amino acids. Large values (10–12 Hz) indicate the antiperiplanar arrangement of Cα/Cβ substituents and of NH-CHβ and are consistent with an extended conformation. Smaller values (3–4 Hz) indicate a gauche conformation corresponding to turn/helix constructs. Since we started from a syn-amino acid, an extended conformation could be expected. On the other hand, the presence of helicogenic alanine could influence the secondary conformation of the peptide. As shown in Table 1, large J2,3 and JNH−CHβ values were detected for peptides containing β-2R,3R-Fpg amino acid indicating their extended conformation. Lower J values were detected for peptides containing β-2S,3S-Fpg amino acid, mostly for J2,3 of amino acids at position 2 and 4 of peptide 12. On the other hand, these J values are higher with respect to those reported for helix constructs containing β-amino acids (J 3–4 Hz), suggesting that for 12 more than one preferred conformation is present (Seebach et al., 1999).
A further main difference between the two series is the MeAla resonances. According to an extended conformation, MeAla3 of 7 (see Figure 7A) is located between Phβ2 and ArFβ4 possessing the same orientation. This induces a strong shielding effect on the methyl group that resonates at higher field (δ 0.48). Similarly, 11 is characterized by two shielded methyl groups [MeAla3 (δ 0.32); MeAla5 (δ 0.35)], in agreement with an extended conformation (Figure 8A). MeAla3 (δ 0.97) in 8, as well as all three MeAla [MeAla1(δ 1.36); MeAla3 (δ 1.20); MeAla5 (δ 1.01)] of 12, not affected by this electronic effect, resonate at lower field. Furthermore, differences of NH chemical shift are mostly found for hexapeptides 11 and 12, being the NH resonances of this last at lower field.
The temperature dependence experiments of the NH proton chemical shifts variation, giving insights on NH inaccessibility or on NH-bond network (Augspurger et al., 1995) were performed for peptides 7, 8, and 12 (NHs proximity to aromatic protons prevented a clear overview of their behavior in peptide 11).
Very high Δδ/ΔT values (>-9.4 ppb K−1; this value is not detectable for NHβ2, overlapped signal) were found for 7 and 8, indicating absence of H-bonds. As exception, a medium value (−4.3 ppb K−1) was detected for NHAla1 of 8, indicating an equilibrium between a H-bonded and non-bonded status or a shielded environmental (Figure 3).
These results agree with IR data indicating, for both peptides, a low intensity of the bond at about 3,320 cm−1 and a lower area for 7 with respect to 8 (Figure 2).
Smaller Δδ/ΔT values were found for NHs of 12 with respect to 8, mostly for NHAla1, NHβ6, and NHAla3 (−2.9, −3.01, −4.4 ppb K−1, respectively; Figure 4). These data are consistent with IR analyses (Figure 2B) indicating the tendency of 12 to give strong/medium intramolecular C = O···H-N H-bonds.
A complete set of CHα/NHβ (i, i+1) and Hβ-2/NHα (i,i+1) NOEs characterizes peptides 7 (Figure S2), 8 (Figure S3), and 12 (Figure S8). A peculiar NOE for 8 is between H-5 of Arβ4 (δ 7.11) with Phβ2 (δ 7.31, Figure 5). The resonances of Ph protons in both Ph-3β2 and Ph-3β4 range in δ 7.31–7.22 region. On the other hand, the antiperiplanar position of Ph with ArF of β-4 amino acid (J2,3 10.3 Hz) excludes that the detected spatial proximity between ArF and Ph derives from the aromatic moieties of the same amino acid. The formation of a turn in β-2/Ala3/β-4 region is suggested, as confirmed by computational data (Figure 7C), that is consistent with the deviation of JNH−CHα values characterizing the extended peptides (Table 1).
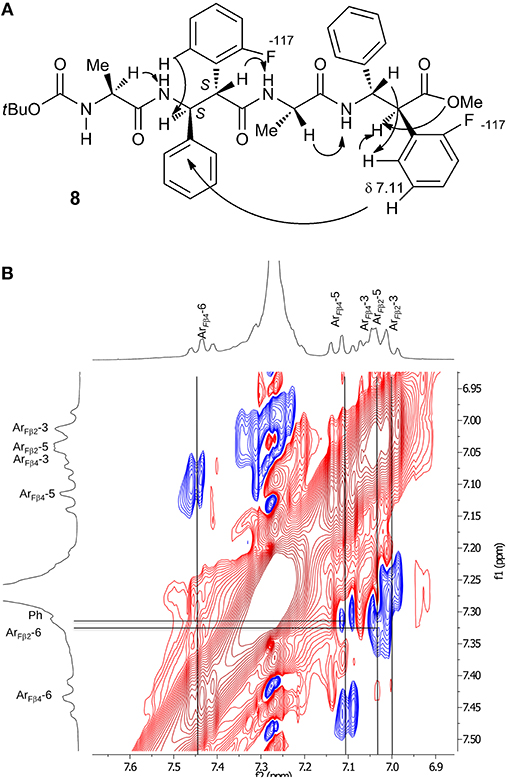
Figure 5. (A) NOEs of peptide 8. (B) Zoom of aromatic region spectrum (CDCl3, 10 mM, 293 K, 400 MHz, 300 ms).
Considering peptide 12, apart the above-mentioned NOEs, only a weak spatial proximity was detected between NH-2 and NH-3 (Figure S8). Detection of possible NOEs between non-sequential Ph/Ar protons, as shown for 8, were prevented because of the presence of several overlapped aryl protons.
Focusing on 11, only some CHα/NHβ (i, i+1) and Hβ-2/NHα (i,i+1) NOEs were detected, because several signals are overlapped preventing certain NOEs. Interestingly, the formation of an antiparallel pleated sheet arrangement in which the C-terminus of one strand is faced on the N-terminus of a second strand is supported by Noesy/Roesy experiments. Different inter-strand spatial proximities are present that are MeAla1 with both MeAla5(m) and OMe (vw) and Boc with both CHβ6-2(m) and OMe(w) (Figure 6). 1H NMR studies at variable concentration (1.15–9.10 mM; Figure S32) showed concentration dependence chemical shift changes indicating aggregation. Noesy/Roesy experiments confirmed this tendency also at very low concentration (1.5 mM in CDCl3, Figure S5). DMSO-solvent titration of 11 was performed that was matched with Tocsy experiments (0%, 8% and 20% v/v of DMSO in CDCl3) to ensure the correct correlation between NH with the corresponding amino acid. A strong NH downshift was detected for NHβ6, NHβ4, and NHAla−3 (Δδ > 1, 0.89, and 0.54, respectively) indicating their solvent exposure. A medium-weak H-bond (Δδ 0.35) was detected for NH-2. Instead, NH-1 and NH-5 are strongly involved in a H-bond (Δδ < 0.05) (Figure S7). As a result, our hypothesis is that this antiparallel sheet is stabilized by intermolecular H-bond.
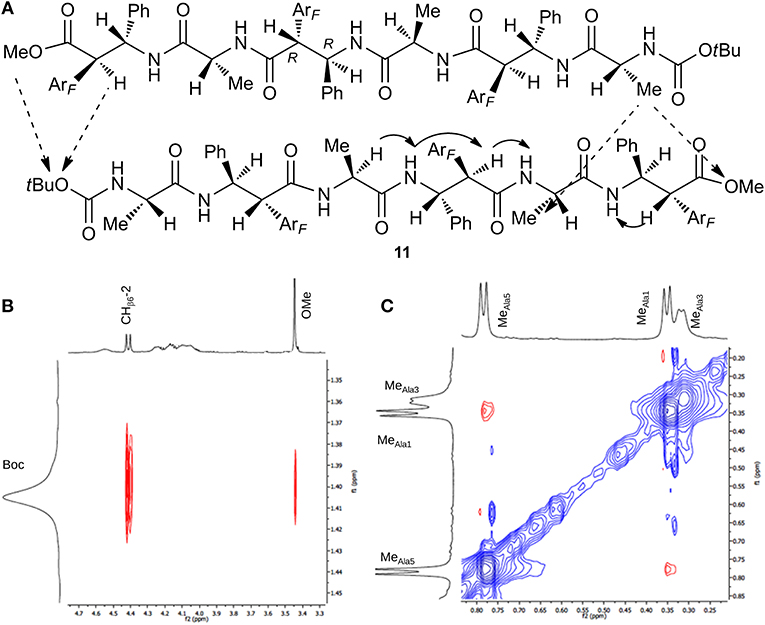
Figure 6. (A) Intra- (continuous arrow) and inter- (dotted arrow) strand NOEs for peptide 11. (B) ROESY zoom of Boc region. (C) ROESY zoom of Me region spectrum (CDCl3, 10 mM, 293 K, 400 MHz, 200 ms).
The NMR analysis of 11 is also performed in DMSO-d6 (10 mM), that is known to be a dissociating solvent. A similar 1H NMR spectrum was detected concerning protons multiplicity, indicating an extended architecture, except for the NH resonances that are at lower field (Table TS4). This allowed to detect a complete set of CHα/NHβ and Hβ-2/NHα (i,i+1) spatial proximities. As expected, inter-strand spatial proximities were absent in the ROESY experiment (Figure S6).
Computational Studies
To gain additional insight on the conformational behavior of both tetra (m7 and m8) and hexapeptides (m11 and m12), we performed accelerated molecular dynamics (aMD) simulations in explicit CHCl3 solvent (Pierce et al., 2012).
Concerning the tetrapeptides, both clustering and hydrogen bond (H-bond) analyses suggested a significantly different conformational behavior for m7 and m8. The analyses of the aMD trajectories of both peptides only evidenced two potential H-bonds, one between the acetyl carbonyl and NHβ2, the other between C = Oβ2 and NHβ4. However, the occupancy is about 20% higher in the case of m8 (Table 2), suggesting that m8 can adopt a more compact conformation. This behavior is also confirmed by the analysis of the ϕ, ψ, and θ dihedrals of the representative structures of the most populated clusters (Table 3).
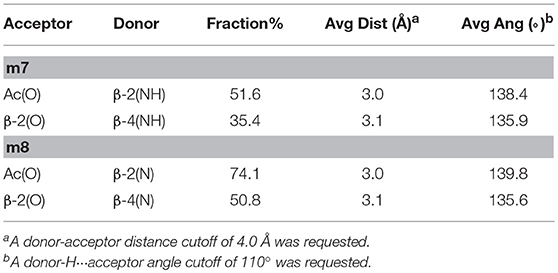
Table 2. H-bonds analysis for tetrapeptideds m7 and m8 performed on the last 200 ns of the aMD trajectories.
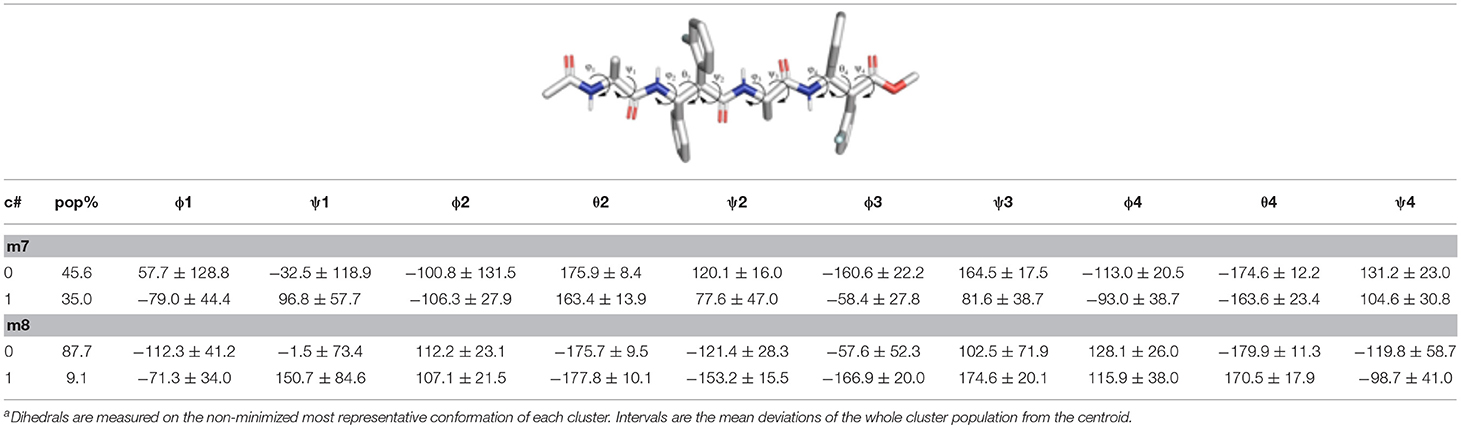
Table 3. Structural analysisa of the top clusters for the m7 and m8 tetrapeptides obtained from the analysis of the last 200 ns of the aMD simulations.
Indeed, m7 prefers an almost completely extended conformation (Figure 7A), except for a γ-turn involving the acetyl cap at the N-terminus. This conformation is consistent with the high chemical shift observed for MeAla3 (see above). At a minor extent, a geometry characterized by two γ-turns, the first involving the acetyl cap and the NHβ2, the second between the C = Oβ2 carbonyl and NHβ4, is also observed as the secondary cluster (Figure 7B). This conformation is possibly stabilized by a π-π interaction between the Phβ2 and the ArFβ4. However, the low H-bond population, the relatively long donor-acceptor distances and narrow donor-H···angles (Table 2) suggest that these γ-turns are not stable. Overall, these data suggest that m7 can switch from a completely extended conformation to a partially folded conformation. However, this last is only marginally stabilized by π-π interactions between the two β-amino acids and by γ-turn H-bonds. The equilibrium is consequently shifted toward the extended conformation, coherently with NMR observations.
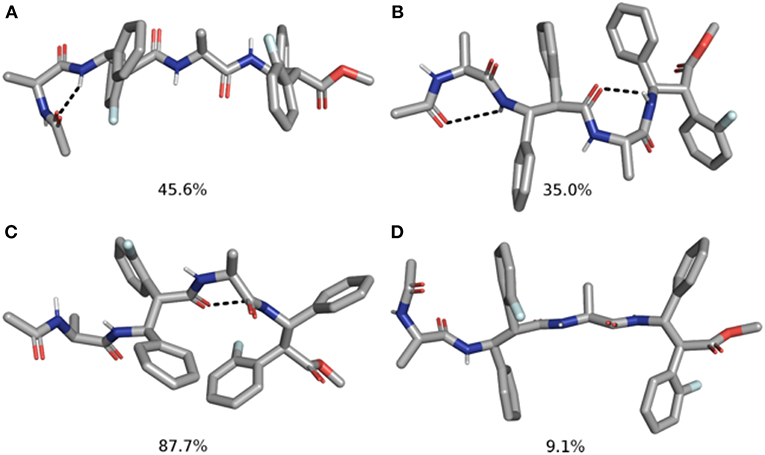
Figure 7. Top 2 clusters representative structures of m7 (A,B) and m8 (C,D) tetrapeptides. The populations of each cluster are indicated as a percentage. Hydrogen bonds are indicated as dashed black lines.
Conversely, m8 tetrapeptide showed an opposite behavior. A highly populated primary cluster (pop = 87.7%; Figure 7C) is indeed characterized by a relatively compact geometry, showing a γ-turn between β-2 and β-4 and a π-π interaction between the Phβ2 and ArFβ4. This interaction is consistent with Noesy experiments (see above). A poorly sampled fully extended conformation (pop = 9.1%) was also found as a secondary cluster (Figure 7D).
The analysis of the aMD simulations performed on m11 and m12 hexapeptides led to similar conclusions, compared to the tetrapeptide series. Indeed, m11 most populated cluster (pop = 43.3%) corresponds to an extended conformation, as showed by the ϕ, ψ, and θ dihedrals of the corresponding representative structure (Table 4 and Figure 8A). The second most populated cluster (pop = 29.5%) represents conformations showing π-π interactions involving the ArF of both β-2 and β-4, while the C-terminus remains extended (Table 4 and Figure 8B). Only the third cluster (pop = 19.8%) presented the ArF/Ph π-π interactions between the aryl groups of β-2/β-4 and of β-4/β-6, similarly to what observed in m7. We could also observe γ-turns between the backbone C = O of Ac capping group with NHβ2, and carbonyls of β-2 and β-4 with NHβ4 and NHβ6 (Table 5).
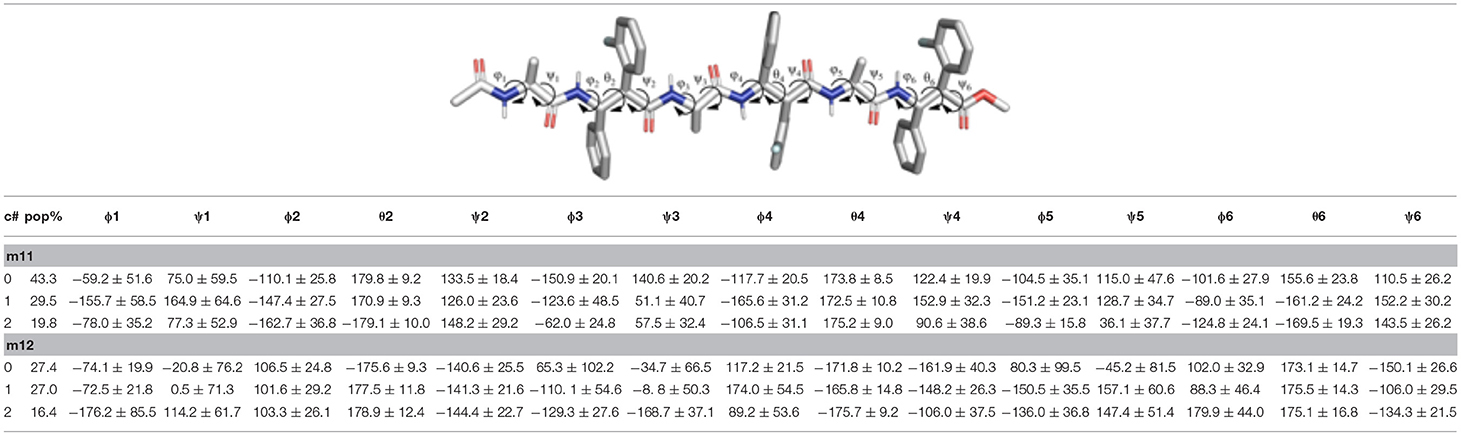
Table 4. Structural analysis of the top 3 clusters for the m11 and m12 hexapeptides obtained from the analysis of the last 200 ns of the aMD simulations.
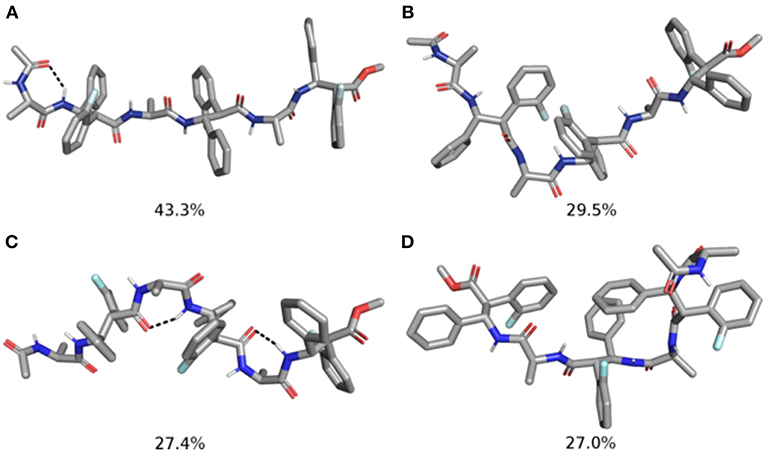
Figure 8. Representative structures of the two most populated clusters of m11 (A,B) and m12 (C,D) hexapeptides. The population of each cluster is indicated as a percentage. Hydrogen bonds are depicted as dashed black lines.
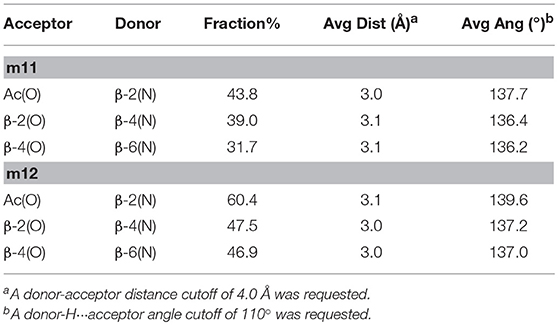
Table 5. H-bonds Analysis for Hexapeptides m11 and m12 Performed on the Last 200 ns of the aMD Trajectory.
The top two clusters of the m12 hexapeptide are relatively low populated (pop = 27.4 and 27.0%, respectively). However, they both show a folded conformation, with π-π interactions between the aromatic rings of the β-amino acids, and the above described γ-turns (Figures 8C,D; Table 4). This behavior resembles what previously observed for the tetrapeptides series, where m8 principal geometry was more compact, compared to m7. A similar behavior between the hexa- and tetrapeptide series was also observed in terms of H-bonds. Indeed, the occupancies of the H-bonds defining the γ-turns are about 15% higher in m12, compared to m11 (Table 5). In the hexapeptide series, also a relatively populated third cluster (pop = 16.4%) showed a partially folded representative structure. Indeed, computed ϕ, ψ, and θ dihedrals (ψ3, ϕ4, and θ4, in particular), assume values that are compatible with interactions between the Phβ2 and the ArFβ4, as well as between the Phβ4 and ArFβ6.
Overall, the computational analysis shows a coherent influence of the stereochemical configuration of α and β carbons on the investigated β-amino acids inserted in model Ac(S-Ala-β-Fpg)nOMe peptides. Indeed, our observation suggest that β-2R,3R-Fpg helps to stabilize an extended conformation. Conversely, β-2S,3S-Fpg seems to induce more compact conformations, possibly stabilized by inter-residue π-π interactions and/or by γ-turns. This behavior is also supported by JNH−CHβ and J2,3 coupling constants obtained by 1H NMR experiments. Indeed, larger J values were obtained for both 7 and 11, compared to 8 and 12, suggesting a more folded conformation for these latter peptides (Li et al., 2015). To provide additional evidences of this behavior, we compared the frequency of the radius of gyrations (RoG) sampled in the last 200 ns of simulation (Figure 9). For both tetra- and hexapeptides, it can be observed that a larger RoG is sampled more frequently for peptides containing β-2R,3R-Fpg, compared to peptides containing β-2S,3S-Fpg. Figure 9 also shows that two peaks are found for m11, centered at 6.5 and 8.0 Å. This is coherent with the results of the clustering analysis, which evidence the possibility of a “bending” of the extended geometry at the Ala3/β4 level (Figure 8). m7 instead shows a “spiked” peak centered at 5.0 Å, corresponding to the representative geometry shown in Figure 7A. The two “spikes” at 4.8 and 5.4 Å correspond to the breaking of the N-terminal γ-turn (involving the acetyl cap) and to the forming of the additional γ-turn (involving β-2 and β-4), respectively. Conversely, both m8 and m12 show a main peak (sharper, for m8) that is centered onto a RoG value that is significantly lower to that computed for the corresponding peptides containing β-2R,3R-Fpg.
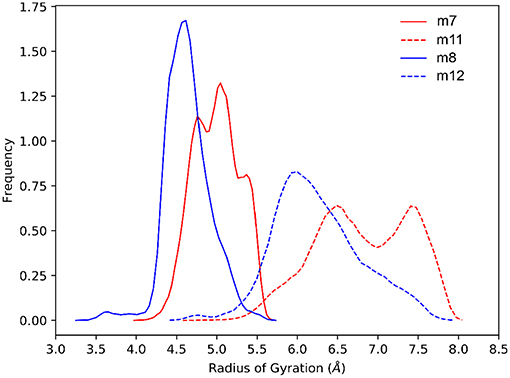
Figure 9. Comparison of the normalized sampling frequencies of RoG calculated from the last 200 ns of the aMD trajectories of m7, m8, m11, and m12.
Conclusions
Taken together these data, we can conclude that the architecture of the above peptides depends on the balance between the features of the selected amino acids, i.e., the syn β-amino acid, favoring an extended conformation, and alanine, inducing turns. The chirality of the two stereogenic centers of β-amino acid remarkably affects the peptide 3D-structures. A preferred extended conformation was found matching the β-R,R-amino acid with S-alanine. This is confirmed by J values of both tetra- and hexapeptides containing β-2R,3R-Fpg as well as by computational analysis. The extended conformation of this peptide induces the formation of an antiparallel β-sheet, also at low concentration, as documented by NMR data of 11. Our hypothesis is that the formation of this pleated sheet is favored by π,π-interactions of aromatic moieties and is stabilized by H-bonds. IR analysis of this peptide indicates the involvement of NHs in H-bonds, confirmed by the titration experiment (NH-1 and NH-6 involvement). The NH-network proposed by computational experiments does not fit with NMR data. On the other hand, computational data are consistent with a 11 single strand and not with pleated sheet architecture. In this case the formation of H-bonds is driven by γ-turn formation.
β-S,S-amino acid with S-alanine gives more compact folded structures that are probably driven by alanine moieties. In fact, peptides containing β-2S,3S-Fpg display a higher stability and a larger content of an ordered secondary structure as compared to the peptides containing β-2R,3R-Fpg. Both NMR and computational data support the formation of a turn for 8 stabilized by π-π interactions between Phβ2 and ArFβ4. IR absorption analysis and experiments at variable temperature confirm that the longer oligomer 12 is stabilized by intramolecular C = O···H-N bonds. On the other hand, J values indicate that an equilibrium occurred between multiple compact conformations.
Author Contributions
MG conceived the research. RB and SP carried out change with RB, EB, and SP carried out the synthesis. MG, FC, and FF carried out the spectroscopic analyses. AC and IM carried out the computational studies. MG and AC supervised the work. MG, AC, and RB wrote the paper. All the authors revised the manuscript.
Funding
We are grateful to MIUR for funding (2015 PRIN project Prot. 20157WW5EH) and to European Union's Horizon 2020 research and innovation programme (Marie Skłodowska-Curie grant agreement No. 675527).
Conflict of Interest Statement
The authors declare that the research was conducted in the absence of any commercial or financial relationships that could be construed as a potential conflict of interest.
Supplementary Material
The Supplementary Material for this article can be found online at: https://www.frontiersin.org/articles/10.3389/fchem.2019.00192/full#supplementary-material
References
Angelici, G., Luppi, G., Kaptein, B., Broxterman, Q. B., Hofmann, H. J., and Tomasini, C. (2007). Synthesis and secondary structure of alternate α,β-hybrid peptides containing oxazolidin-2-one moieties. Eur. J. Org. Chem. 2007, 2713–2721. doi: 10.1002/ejoc.200700134
Augspurger, J. D., Bindra, V. A., Scheraga, H. A., and Kuki, A. (1995). Helical stability of de Novo designed α-aminoisobutyric acid-rich peptides at high temperatures. Biochemistry 34, 2566–2576. doi: 10.1021/bi00008a022
Balamurugan, D., and Muraleedharan, K. M. (2012). Unprecedented torsional preferences in trans-β2,3-amino acid residues and formation of 11-helices in α,β2,3-hybrid peptides. Chem. A Eur. J. 18, 9516–9520. doi: 10.1002/chem.201201415
Baldauf, C., and Hofmann, H. J. (2012). Ab initio MO theory—An important tool in foldamer research: prediction of helices in oligomers of ω-amino acids. Helv. Chim. Acta 95, 2348–2383. doi: 10.1002/hlca.201200436
Basuroy, K., Karuppiah, V., and Balaram, P. (2014). C11/C9 Helices in crystals of αβ hybrid peptides and switching structures between helix types by variation in the α-residue. Org. Lett. 16, 4614–4617. doi: 10.1021/ol5021866
Berlicki, Ł., Pilsl, L., Wéber, E., Mándity, I. M., Cabrele, C., Martinek, T. A., et al. (2012). Unique α,β- and α,α,β,β-peptide foldamers based on cis-β-aminocyclopentanecarboxylic acid. Angew. Chemie Int. Ed. 51, 2208–2212. doi: 10.1002/anie.201107702
Bonetti, A., Clerici, F., Foschi, F., Nava, D., Pellegrino, S., Penso, M., et al. (2014). syn/anti Switching by specific heteroatom–titanium coordination in the mannich-like synthesis of 2,3-Diaryl-β-amino acid derivatives. Eur. J. Org. Chem. 15, 3203–3209. doi: 10.1002/ejoc.201400142
Bonetti, A., Pellegrino, S., Das, P., Yuran, S., Bucci, R., Ferri, N., et al. (2015). Dipeptide nanotubes containing unnatural fluorine-substituted β2,3-diarylamino acid and L-alanine as candidates for biomedical applications. Org. Lett. 17, 4468–4471. doi: 10.1021/acs.orglett.5b02132
Bucci, R., Bonetti, A., Clerici, F., Contini, A., Nava, D., Pellegrino, S., et al. (2017a). Tandem tetrahydroisoquinoline-4-carboxylic acid/β-alanine as a new construct able to induce a flexible turn. Chem. A Eur. J. 23, 10822–10831. doi: 10.1002/chem.201701045
Bucci, R., Contini, A., Clerici, F., Pellegrino, S., and Gelmi, M. L. (2019). From glucose to enantiopure morpholino β-amino acid: a new tool for stabilizing γ-turns in peptides. Org. Chem. Front. doi: 10.1039/C8QO01116H
Bucci, R., Das, P., Iannuzzi, F., Feligioni, M., Gandolfi, R., Gelmi, M. L., et al. (2017b). Self-assembly of an amphipathic ααβ-tripeptide into cationic spherical particles for intracellular delivery. Org. Biomol. Chem. 15, 6773–6779. doi: 10.1039/c7ob01693j
Bucci, R., Giofré, S., Clerici, F., Contini, A., Pinto, A., Erba, E., et al. (2018). Tetrahydro-4H-(pyrrolo[3,4-d]isoxazol-3-yl)methanamine: a bicyclic diamino scaffold stabilizing parallel turn conformations. J. Org. Chem. 83, 11493–11501. doi: 10.1021/acs.joc.8b01299
Cabrele, C., Martinek, T. A., Reiser, O., and Berlicki, Ł. (2014). Peptides containing β-amino acid patterns: challenges and successes in medicinal chemistry. J. Med. Chem. 57, 9718–9739. doi: 10.1021/jm5010896
Case, D. A., Babin, V., Berryman, J. T., Betz, R. M., Cai, Q., Cerutti, D. S., et al. (2014). AMBER 14. San Francisco: University of California.
Checco, J. W., and Gellman, S. H. (2016). Targeting recognition surfaces on natural proteins with peptidic foldamers. Curr. Opin. Struct. Biol. 39, 96–105. doi: 10.1016/j.sbi.2016.06.014
Cheng, R. P., Gellman, S. H., and DeGrado, W. F. (2001). β-Peptides: from structure to function. Chem. Rev. 101:3219–3232. doi: 10.1021/cr000045i
Clerici, F., Erba, E., Gelmi, M. L., and Pellegrino, S. (2016). Non-standard amino acids and peptides: from self-assembly to nanomaterials. Tetrahedron Lett. 57, 5540–5550. doi: 10.1016/j.tetlet.2016.11.022
Del Borgo, M. P., Kulkarni, K., and Aguilar, M.-I. (2017). Using β-amino acids and β-peptide templates to create bioactive ligands and biomaterials. Curr. Pharm. Des. 23, 3772–3785. doi: 10.2174/1381612823666170616083031
Dupradeau, F.-Y., Pigache, A., Zaffran, T., Savineau, C., Lelong, R., Grivel, N., et al. (2010). The R.E.D. tools: advances in RESP and ESP charge derivation and force field library building. Phys. Chem. Chem. Phys. 12:7821. doi: 10.1039/c0cp00111b
Gopalan, R. D., Borgo, M. P., Del Mechler, A. I., Perlmutter, P., and Aguilar, M.-I. (2015). Geometrically precise building blocks: the self-assembly of β-peptides. Chem. Biol. 22, 1417–1423. doi: 10.1016/j.chembiol.2015.10.005
Hegedüs, Z., Makra, I., Imre, N., Hetényi, A., Mándity, I. M., Monostori, É., et al. (2016). Foldameric probes for membrane interactions by induced β-sheet folding. Chem. Commun. 52, 1891–1894. doi: 10.1039/c5cc09257d
Horne, W. S. (2011). Peptide and peptoid foldamers in medicinal chemistry. Expert Opin. Drug Discov. 6:1247. doi: 10.1517/17460441.2011.632002
Johnson, L. M., and Gellman, S. H. (2013). “Chapter nineteen—α-helix mimicry with α/β-peptides,” in Methods in Protein Design Methods in Enzymology, ed A. E. Keating (Amsterdam: Academic Press), 407–429.
Lee, W., Kwon, S., Kang, P., Guzei, I. A., and Choi, S. H. (2014). Helical folding of α,β-peptides containing β-amino acids with an eight-membered ring constraint. Org. Biomol. Chem. 12, 2641–2644. doi: 10.1039/c4ob00266k
Li, F., Lee, J. H., Grishaev, A., Ying, J., and Bax, A. (2015). High accuracy of karplus equations for relating three-bond j couplings to protein backbone torsion angles. Chem. Phys. Chem. 16, 572–578. doi: 10.1002/cphc.201402704
Maier, J. A., Martinez, C., Kasavajhala, K., Wickstrom, L., Hauser, K. E., and Simmerling, C. (2015). ff14SB: improving the accuracy of protein side chain and backbone parameters from ff99SB. J. Chem. Theory Comput. 11, 3696–3713. doi: 10.1021/acs.jctc.5b00255
March, T. L., Johnston, M. R., Duggan, P. J., and Gardiner, J. (2012). Synthesis, structure, and biological applications of α-fluorinated β-amino acids and derivatives. Chem. Biodivers. 9, 2410–2441. doi: 10.1002/cbdv.201200307
Molecular Operating Environment (2013). Chemical Computing Group Releases MOE Version 2013.08. Montreal, QC: Chemical Computing Group Inc.
Palumbo, M., da Rin, S., Bonora, G. M., and Toniolo, C. (1976). Linear oligopeptides, 29. Infrared conformational analysis of homo-oligopeptides in the solid state and in solution. Die Makromol. Chem. 177, 1477–1492. doi: 10.1002/macp.1976.021770519
Pellegrino, S., Bonetti, A., Clerici, F., Contini, A., Moretto, A., Soave, R., et al. (2015). 1 H -Azepine-2-oxo-5-amino-5-carboxylic acid: a 3.10 helix inducer and an effective tool for functionalized gold nanoparticles. J. Org. Chem. 80, 5507–5516. doi: 10.1021/acs.joc.5b00396
Pellegrino, S., Clerici, F., and Gelmi, M. L. (2008). β-Hydroxynorbornane amino acid derivatives: valuable synthons for the diastereoselective preparation of substituted cyclopentylglycine derivatives. Tetrahedron 64, 5657–5665. doi: 10.1016/j.tet.2008.04.038
Pellegrino, S., Facchetti, G., Contini, A., Gelmi, M. L., Erba, E., Gandolfi, R., et al. (2016). Ctr-1 Mets7 motif inspiring new peptide ligands for Cu(I)-catalyzed asymmetric Henry reactions under green conditions. RSC Adv. 6, 71529–71533. doi: 10.1039/c6ra16255j
Penso, M., Foschi, F., Pellegrino, S., Testa, A., and Gelmi, M. L. (2012). Diastereoselective protocols for the synthesis of 2,3-trans- nd 2,3-cis-6-methoxy-morpholine-2-carboxylic acid derivatives. J. Org. Chem. 77, 3454–3461. doi: 10.1021/jo300221y
Pierce, L. C. T., Salomon-Ferrer, R., Augusto, F., de Oliveira, C., McCammon, J. A., and Walker, R. C. (2012). Routine access to millisecond time scale events with accelerated molecular dynamics. J. Chem. Theory Comput. 8, 2997–3002. doi: 10.1021/ct300284c
Pilsl, L. K. A., and Reiser, O. (2011). α/β-Peptide foldamers: state of the art. Amino Acids 41, 709–718. doi: 10.1007/s00726-011-0894-2
Ruffoni, A., Cavanna, M. V., Argentiere, S., Locarno, S., Pellegrino, S., Gelmi, M. L., et al. (2016). Aqueous self-assembly of short hydrophobic peptides containing norbornene amino acid into supramolecular structures with spherical shape. RSC Adv. 6, 90754–90759. doi: 10.1039/c6ra17116h
Ruffoni, A., Contini, A., Soave, R., Lo Presti, L., Esposto, I., Maffucci, I., et al. (2015). Model peptides containing the 3-sulfanyl-norbornene amino acid, a conformationally constrained cysteine analogue effective inducer of 3.10-helix secondary structures. RSC Adv. 5, 32643–32656. doi: 10.1039/c5ra03805g
Seebach, D., Abele, S., Gademann, K., and Jaun, B. (1999). Pleated sheets and turns of β-peptides with proteinogenic side chains. Angew. Chem. Int. Ed. 38:1595.
Seebach, D., Beck, A. K., and Bierbaum, D. J. (2004). The World of β- and γ-peptides comprised of homologated proteinogenic amino acids and other components. Chem. Biodivers. 1, 1111–1239. doi: 10.1002/cbdv.200490087
Seebach, D., and Gardiner, J. (2008). β-peptidic peptidomimetics. ACC. Chem. Res. 41, 1366–1375. doi: 10.1021/ar700263g
Seebach, D., Hook, D. F., and Glättli, A. (2006). Helices and other secondary structures of β- and γ-peptides. Pept. Sci. 84, 23–37. doi: 10.1002/bip.20391
Seebach, D., Overhand, M., Kühnle, F. N. M., Martinoni, B., Oberer, L., Hommel, U., et al. (1996). β-Peptides: synthesis by Arndt-Eistert homologation with concomitant peptide coupling. Structure determination by NMR and CD spectroscopy and by X-ray crystallography. Helical secondary structure of a β-hexapeptide in solution and its stability towards pepsin. Helv. Chim. Acta 79, 913–941. doi: 10.1002/hlca.19960790402
Sharma, G. V. M., Nagendar, P., Jayaprakash, P., Radha Krishna, P., Ramakrishna, K. V. S., and Kunwar, A. C. (2005). 9/11 Mixed helices in α/β peptides derived from C-linked carbo-β-amino acid and L-Ala repeats. Angew. Chem. Int. Ed. 44, 5878–5882. doi: 10.1002/anie.200501247
Srinivasulu, G., Kiran Kumar, S., Sharma, G. V. M., and Kunwar, A. C. (2006). 11/9-Mixed helices in the e α/β-peptides derived from alternating α- and β-amino acids with proteinogenic side chains. J. Org. Chem. 71, 8395–8400. doi: 10.1021/jo0612980
Tonali, N., Kaffy, J., Soulier, J. L., Gelmi, M. L., Erba, E., Taverna, M., et al. (2018). Structure-activity relationships of β-hairpin mimics as modulators of amyloid β-peptide aggregation. Eur. J. Med. Chem. 154, 280–293. doi: 10.1016/j.ejmech.2018.05.018
Toniolo, C., Bonora, G. M., Barone, V., Bavoso, A., Benedetti, E., Di Blasio, B., et al. (1985). Conformation of pleionomers of α-aminoisobutyric acid. Macromolecules 18, 895–902. doi: 10.1021/ma00147a013
Vasudev, P. G., Chatterjee, S., Shamala, N., and Balaram, P. (2011). Structural chemistry of peptides containing backbone expanded amino acid residues: conformational features of β, γ, and hybrid peptides. Chem. Rev. 111, 657–687. doi: 10.1021/cr100100x
Wang, P. S. P., and Schepartz, A. (2016). β-peptide bundles: design. build. analyze. biosynthesize. Chem. Commun. 52, 7420–7432. doi: 10.1039/C6CC01546H
Wu, Y., Han, W. E. I., Wang, D., Gao, Y. I., and Zhao, Y. (2008). Theoretical analysis of secondary structures of β-peptides. ACC. Chem. Res. 41, 1418–1427. doi: 10.1021/ar800070b
Keywords: β2,3-diaryl-amino acid, α, β2,3-peptide, extended peptide, antiparallel β-sheet, conformational analyses, foldamers
Citation: Bucci R, Contini A, Clerici F, Beccalli EM, Formaggio F, Maffucci I, Pellegrino S and Gelmi ML (2019) Fluoro-Aryl Substituted α,β2,3-Peptides in the Development of Foldameric Antiparallel β-Sheets: A Conformational Study. Front. Chem. 7:192. doi: 10.3389/fchem.2019.00192
Received: 31 January 2019; Accepted: 13 March 2019;
Published: 02 April 2019.
Edited by:
Assaf Friedler, Hebrew University of Jerusalem, IsraelReviewed by:
Vladimir Torbeev, Université de Strasbourg, FranceJames Gardiner, Commonwealth Scientific and Industrial Research Organisation (CSIRO), Australia
Copyright © 2019 Bucci, Contini, Clerici, Beccalli, Formaggio, Maffucci, Pellegrino and Gelmi. This is an open-access article distributed under the terms of the Creative Commons Attribution License (CC BY). The use, distribution or reproduction in other forums is permitted, provided the original author(s) and the copyright owner(s) are credited and that the original publication in this journal is cited, in accordance with accepted academic practice. No use, distribution or reproduction is permitted which does not comply with these terms.
*Correspondence: Maria Luisa Gelmi, bWFyaWFsdWlzYS5nZWxtaUB1bmltaS5pdA==