Corrigendum: A Molecular CO2 Reduction Catalyst Based on Giant Polyoxometalate {Mo368}
- 1Eco-Friendly Applied Materials Laboratory, College of Chemistry, Central China Normal University, Wuhan, China
- 2Eco-Friendly Applied Materials Laboratory, Department of Chemical Sciences, Materials Science Centre, Mohanpur, Indian Institute of Science Education & Research, Kolkata, India
Photocatalytic CO2 reduction in water is one of the most attractive research pursuits of our time. In this article we report a giant polyoxometalate {Mo368} based homogeneous catalytic system, which efficiently reduces CO2 to formic acid with a maximum turnover number (TON) of 27,666, turnover frequency (TOF) of 4,611 h−1 and external quantum efficiency of the reaction is 0.6%. The catalytic system oxidizes water and releases electrons, and these electrons are further utilized for the reduction of CO2 to formic acid. A maximum of 8.3 mmol of formic acid was observed with the loading of 0.3 μmol of the catalyst. Our catalyst material is also stable throughout the reaction. The starting materials for this experiment are CO2 and H2O and the end products are HCOOH and O2. The formic acid formed in this reaction is an important H2 gas carrier and thus significant in renewable energy research.
Introduction
The CO2 concentration in environment is ever increasing. Thus, to find out a suitable pathway to recycle CO2 to an energy rich material is a crucial challenge nowadays (Hoffert et al., 2002; Crabtree and Lewis, 2007; Meinshausen et al., 2009; Mikkelsen et al., 2010; Garai et al., 2012; Bandeira et al., 2015; Twidell and Weir, 2015). The depletion of fossil fuel during production of energy increases the CO2 level in the environment (Hoel and Kverndokk, 1996; Höök and Tang, 2013). It is also known that abundance of fossil fuel is limited. Thus, it is necessary to find out a new pathway which can produce energy without hampering the environment and burning fossil fuels. With this end in view if we convert a greenhouse gas like CO2 to energy rich material it would be very interesting and helpful for a sustainable environment and economy (Khenkin et al., 2010; Rankin and Cummins, 2010; Wang W. et al., 2011; Bontemps et al., 2012; Kuhl et al., 2012; Ohtsu and Tanaka, 2012; Wesselbaum et al., 2012; Xi et al., 2012; Arai et al., 2013; Costentin et al., 2013; Asadi et al., 2014; Blondiaux et al., 2014; Herrero et al., 2014; Kim et al., 2014; Kou et al., 2014; Lu et al., 2014; Studt et al., 2014; Zhang et al., 2014; Gao et al., 2015; Kornienko et al., 2015; Liu et al., 2015; Marszewski et al., 2015; Matlachowski and Schwalbe, 2015; Roberts et al., 2015; Sypaseuth et al., 2015; Iwase et al., 2016; Kuriki et al., 2017). However, the challenge is as CO2 is a very stable oxide of carbon at its stable oxidation state, a large amount of energy is required to activate CO2. Activation of CO2 is a challenge. Here this challenge is addressed via photochemical reduction of CO2 to HCOOH using an unusually large giant POM cluster, first synthesized by Müller group in Bielefeld {Mo368} (Müller et al., 2002, 2004; Müller and Roy, 2003). The TON (27,666) and TOF (4,611 h−1) of this conversion is quite high as compared to other reported molecular catalysts (Tamaki et al., 2015). In photosynthesis, nature continuously activates CO2 with ease where CO2 from environment is converted into carbohydrates through photosensitization using sun as the source of light. The process happens in nature such that first water gets oxidized and releases electrons which further reduce CO2 to carbohydrate in a long catalytic cycle (Hatch, 1976). Drawing inspiration from this process, use of a proper catalytic system can lead to the conversion of CO2 to different high energy carbon material under light. Many potential catalytic systems have been developed over the last few decades for the synthesis of different fuel and organic materials from CO2. Some of the catalysts initially bind with CO2 and further reduce it to different reduced materials (Castro-Rodriguez and Meyer, 2005; Laitar et al., 2005; Sakakura et al., 2007; Sadique et al., 2008; Cokoja et al., 2011; Langer et al., 2011; Mandal and Roesky, 2011; Sato et al., 2011; Schmeier et al., 2011). Some catalysts convert CO2 following electrochemical methods (Amatore and Saveant, 1981; Hori et al., 1989; Whipple and Kenis, 2010; Agarwal et al., 2011; Finn et al., 2012; Kuhl et al., 2012; Sullivan et al., 2012; Costentin et al., 2013; Zhang et al., 2014; Kornienko et al., 2015; Lin et al., 2015). Electrochemically CO2 can be reduced to different alkane, ethylene, CO, and HCOOH. The major problem associated with this process is the selectivity in the reduction of CO2 to different reduced products. Recently Yaghi group showed that electrochemically CO2 can be reduced to CO selectively using Co-porphyrin based COF and MOF catalysts in water (Kornienko et al., 2015; Lin et al., 2015). Imminent challenge lies in the photochemical reduction of CO2 (Matsuoka et al., 1993; Daniel and Astruc, 2004; Schwartzberg and Zhang, 2008; Takeda et al., 2008; Li and Zhang, 2009; Morris et al., 2009; Dhakshinamoorthy et al., 2012; Tornow et al., 2012; Sato et al., 2013; Sekizawa et al., 2013; Zhu et al., 2013; Wang et al., 2014; Kim et al., 2015; Li et al., 2015; Low et al., 2015). Among various proposed technologies, photocatalytic CO2 reduction has been known as one of the most important strategies for solving both global energy and environmental problems due to its low cost, cleanliness, and environmental friendliness (Maginn, 2010; Iizuka et al., 2011; Sato et al., 2011; Yu et al., 2014). Lehn (Hawecker et al., 1984) and Sauvage (Beley et al., 1984) started photochemical and photoelectrochemical CO2 reduction in 1980 using different rhenium, ruthenium, nickel, and cobalt complexes of different macrocycles as catalysts (Fisher and Eisenberg, 1980; Beley et al., 1984). Many hybrid nano materials are potential catalysts for this purpose. Also, some ruthenium and rhenium-based metal complexes can reduce CO2 in presence of light. Photo-electrochemical method is another important tool in this regard (Halmann, 1978; Barton et al., 2008). The major problem associated with photochemical CO2 reduction is the use of a sacrificial electron donor, an organic amine, which cannot be recovered from the reaction (Takeda et al., 2008; Morris et al., 2009). One of the interesting solution of this problem is using water as a sacrificial electron donor (Wang C. et al., 2011; Kim et al., 2014). However, all such avenues for CO2 reduction suffers from low yield of the reduced product. Moreover, the catalyst materials are also expensive. Thus, it is necessary to develop a catalyst which is inexpensive, easy to synthesize and can reduce CO2 in water with promising yield. Till date the photo catalyst materials used for CO2 reduction in water are majorly heterogeneous (Barton et al., 2008; Xi et al., 2012; Kuriki et al., 2016). Homogeneous photochemical CO2 reduction in water is also reported (Nakada et al., 2016).
A wide variety of catalysts both homogenous and heterogenous have been reported for the reduction of CO2 to formic acid ranging from macrocycles (Chen et al., 2015; Ikeyama and Amao, 2018), hybrid materials (Yadav et al., 2012; Sekizawa et al., 2013; Yoshitomi et al., 2015), ionic liquids (Lu et al., 2017), nanoparticles (Kortlever et al., 2015), B doped nanodiamonds (Ikemiya et al., 2018) to alloys (Bai et al., 2017) with some of them reaching selectivity as high as 100%. On the other hand, reports on photochemical CO2 reduction to HCOOH using molecular catalysts mainly employs Ru based complexes as the active catalyst (Boston et al., 2013). Use of bipyridine based Ru(II) complexes with an external light sensitizer have been reported for the selective reduction of CO2 to formic acid (80%) with a TON = 526 (Rosas-Hernández et al., 2015). By employing Ru based supramolecular photocatalysts which acts as both light sensitizer and catalyst, Ishitani et al. have showed selective reduction of CO2 to formic acid in the presence of an external reductant (Tamaki et al., 2012). Further they have tuned the catalytic activity of Ru(II)-Ru(II) supramolecular photocatalyst [Ru2-Ru(CO)] by employing a suitable reductant to increase formic acid selectivity (87%) and TONHCOOH = 2,766 (Tamaki et al., 2015). Here a synergistic interaction between the reductant employed and the photocatalyst is dictating the outcome of the reaction. These catalysts have an advantage of visible light absorption but require a need for an external electron donor, which was either added externally or added as a part of the solvent. Also, a similar RuReCl photocatalyst was investigated in aqueous solution but the efficiency of formic acid production was low due to the degradation of photocatalyst owing to the back-electron transfer from one electron reduced species to the photosensitizer unit (Nakada et al., 2015). In contrast, in this work a giant {Mo368} POM based homogenous photocatalyst system is used to achieve higher selectivity and TON toward formic acid production by employing water solvent as the electron donor.
Recently our group has reported molybdenum based heterogeneous Soft-Oxometalate materials as efficient catalysts for CO2 reduction reaction (Das et al., 2016). To achieve the same in homogenous realm, an oxo-molybdate based catalyst material which is completely soluble in water is chosen. In this work a molecular catalyst based on giant molybdenum polyoxometalate {Mo368} is synthesized, which can reduce CO2 to formic acid in water with a good yield. The mixed valent molybdenum based giant polyoxometalate {Mo368}is synthesized following the literature procedure (Müller et al., 2002). Due to the presence of MoV and MoVI centers in the cluster, intra-valance charge transfer bands are observed in the polyoxometalate. This band imparts deep blue color to the solution. The cluster, a member of molybdenum blue family, is also photoactive and thus there is no need of an addition of any photosensitizer (Das et al., 2016a). Photoactivity of polyoxometalates is known and has been used by us as a catalyst in photo-polymerization (Chen et al., 2013; Das and Roy, 2015, 2016), as well as in photochemical water oxidation reaction (Roy et al., 2007a,b, 2008; Das et al., 2016b; Barman et al., 2018) without addition of any sensitizer and other reactions (Roy et al., 2013). The cluster {Mo368} is extremely efficient as a catalyst and 8.3 mmol of formic acid is obtained with a loading of 0.3 μmol of catalyst. Here the catalyst acts with a maximum turnover number (TON) of 27,666 and turnover frequency (TOF) of 4,611 h−1.
Materials and Methods
All the materials and reagents were purchased from commercially available source and used without further purification. Only water is used as a solvent which was distilled twice before starting any reaction. Before use, all the glass apparatus were first boiled in acid bath then cleaned first with tap water then with double distilled water and finally rinsed with acetone and dried in hot air oven overnight, the temperature of which was set at 90°C. A Luzchem UV photoreactor operating at a power of 64 W (8 × 8 W) with UVA lamp is used for photochemical CO2 reduction reaction in water.
Synthesis of Na48[HXMo368O1032(H2O)240(So4)48]. ca. 1,000H2O
{Mo368} is synthesized by following literature procedure. To a solution of Na2MoO4·2H2O (3 g, 12.4 mmol) in water Na2S2O4 (0.15 g, 0.86 mmol) is added as a reducing agent. The reaction mixture is acidified with 0.5 M H2SO4 (35 mL; immediate color change to blue). The solution is then stored in a closed flask for 2 weeks and after 2 weeks the precipitated deep-blue crystals of {Mo368} are obtained by filtration, Yield: 135 mg.
General Reaction Procedure for Photo Catalytic CO2 Reduction
Photo catalytic carbon dioxide reduction reactions are carried out as follows. Desired amount of {Mo368} is taken in 10 ml of oxygen free double distilled deionized water. The reaction mixture is closed in a two neck round bottom flask and CO2 gas is purged for 2.5 h. Then the reaction mixture is kept in the photo reactor under UV-light (eight 8 W lamps, λ = 373 nm) for different intervals of time. 20 μL of reaction mixture is taken out and further diluted with 10 ml HPLC grade water, which is used for carrying out MALDI-MS experiment. For MALDI-MS experiments the reaction mixture is co-crystallized with HCCA matrix and then the mass spectrum was recorded. Mass spectrum gives a molecular ion peak of formic acid (m/z = 46; Figure S1). Further to detect formic acid, HPLC measurement was performed by injecting the diluted reaction mixture in carbohydrate column with an external standard, 0.1 M formic acid solution. and all the quantitative measurements of formic acid is done by using HPLC experiments. CV measurements with the reaction mixture are done using 0.1 M KCl as an electrolyte, in a potential range of +0 to −2 V with respect to saturated Ag/AgCl reference electrode in a standard 3-electrode system and a peak around −0.59 V in cyclic voltammogram further confirms the formation of formic acid from carbon dioxide in our reaction mixture. To further prove formic acid is formed in our reaction mixture, a coupling reaction is performed with our reaction solution using the following method. A solution of aniline (100 μL) in 2 ml acetonitrile along with 20 mg HATU is added to the reaction mixture as a coupling reagent. The reaction mixture is stirred for 2 h at room temperature. The N-phenylformamide is formed in the reaction mixture. Organic components are extracted with EtOAc (3 × 15 ml) and the EtOAc is evaporated in vacuum. The extracted organic component is dissolved in acetonitrile to perform GC-MS and MALDIMS analysis by following above mentioned procedures. Also we have taken the proton NMR spectrum of amide in CDCl3 solution to further confirm the formation of amide (Figure S1).
Determination of Oxygen Using YSI Dissolve Oxygen Meter
Oxygen formed in the reaction is detected by YSI dissolved oxygen meter. YSI dissolved oxygen meter is first calibrated by degassed water. For that purpose, Nitrogen gas is first bubbled through double distilled water for an hour and then put YSI dissolved oxygen meter into this water and recorded the amount of oxygen present. Next, dissolved oxygen meter is dipped into the reaction system i.e., photo illuminated sample and amount of oxygen present in the reaction system is recorded. Now from the difference of oxygen reading in YSI dissolved oxygen meter the total oxygen formed in the reaction is calculated.
pH Dependent Reaction
These experiments are performed by following the previous procedure using different buffer solutions in the range of pH 5 to 9. Measurement of the formic acid is carried out by similar methods as mentioned above.
Characterization Techniques
Fourier Transform Infra-Red Spectroscopy (FT-IR)
FTIR spectrum of {Mo368} is performed by KBr pallet technique. Initially a pellet is prepared from the mixture of KBr and {Mo368}. FTIR spectrum is recorded by using Perkin Elmer Spectrum RX1 spectrophotometer with FTTR facility in the range 2,000–450 cm−1.
Electronic Absorption Spectroscopy (EAS)
A stable solution of {Mo368} is taken in a quartz cuvette and the electronic absorption spectrum is recorded on U-4100 Spectrophotometer (Liquid).
Cyclic Voltammetry
PAR model 273 potentiostat is used for CV experiment. A platinum wire auxiliary electrode, a glassy carbon working electrode with surface area of 0.002826 cm2 and an aqueous Ag/Ag+ reference electrode which is filled with saturated KCl solution, is used in a three-electrode configuration. All the measurements were performed at 298 K in an inert atmosphere.
Raman Spectroscopy
A LABRAM HR800 Raman spectrometer is employed using a He–Ne ion laser (λ = 1,024 nm) as the excitation source to analyse the sample.
HPLC
All reaction samples were monitored by HITACHI- HPLC system equipped with binary 2,130 pumps, a manual sampler, and 2,490 refractive index detector, maintained at 50°C. The products were separated in sugar ion-exclusion column (250 × 4.8 mm), maintained at 60°C using water as mobile phase with 0.8 mL/min flow rate. The HPLC system is controlled and processed by Inkarp software. Standard Formic acid and Formaldehyde solution were prepared and calibrated. Each time the product obtained is diluted with a known volume of milliq water before analysis to prevent the overloading of the column. All experiments were done in triplicates and the average values were reported within the standard deviations of <2.0%.
Gas Chromatography-Mass Spectrometry (GC-MS)
The products were identified and analyzed using a GCMS-QP-2010 Ultra (M/s. Shimadzu Instruments, Japan) with a HB-5 capillary column (20 m × 0.18 mm) supplied by M/s. J&W Scientific, USA and Trace 1300 GC and ISQ qd single quadruple Mass spectrometer with a TG-5MS capillary column (30 m × 0.32 mm × 0.25 μm) supplied by Thermo Fisher Scientific, India. Gas samples were detected by Molecular sieve 5Å packed column. Thermal Conductivity Detector (TCD) was used for gas samples and Mass Detector (MSD) was used for formic acid measurement.
NMR
1H NMR and 13C NMR spectrum is recorded on 500 MHz Bruker and 400 MHz Jeol NMR machine. For detection of formic acid by 1H NMR, solvent suppression (water) method is used.
Matrix Assisted Laser Desorption Ionization Mass Spectroscopy (MALDI-MS)
All samples were prepared in HPLC grade acetonitrile, by dissolving very minute amount of sample on 2 ml acetonitrile, and then filtering the sample using 0.2 micron syringe filter. Then the sample is cocrystalized with HCCA matrix.
Result and Discussion
Deep blue crystals of {Mo368} with I4mm space group are isolated from the mother liquor in a week by following the literature procedure (Müller et al., 2002). The structure was first determined by A. Müller group using single crystal X-ray diffraction. In the structure, the unit cell contains two hedgehogs like anions which possess D4 symmetry (Figure 1A). The complete cluster contains a central ball shaped unit {Mo288O784(H2O)192(SO4)32} and two other capping units {Mo40O124(H2O)24(SO4)8}. These two units also possess local symmetry, D8d, and C4V, respectively. The molecule has large cavity in which 400 water molecules can be encapsulated. Further this structure can be considered as a hybrid of ring shaped {Mo176} structure and ball shaped {Mo102} structure. The cluster shows typical peaks in the FTIR spectrum which are as follows (Figure 1B), 1,635 (δH2O), 1,123 (νas), 976 (νMo = Ot), 912, 618 cm−1, respectively. Raman spectrum (λ = 1,024 nm) shows peak at 989, 908 (broad peak), 837, and 703 cm−1, respectively (Figure 1C). Electronic absorption spectrum shows maximum absorbance at 747 nm which is observed due to the IVCT transition between MoV to MoVI which is characteristic for molybdenum blue species (Figure 1D). From the above all characterization details, it confirms that {Mo368}is formed in our reaction. Further cyclic voltammogram of {Mo368} under Ar atmosphere is recorded at different scan rates (Figure 1E), which gave three peaks at −0.7, 1.01, and 2.663 V, respectively vs. NHE. From the cyclic voltammogram of the material the position of HOMO and LUMO of the catalyst material was calculated. From the data, the position of HOMO is 2.63 V vs. NHE and the position of LUMO is −0.7 V vs. NHE (Figure 1F). The band gap of the catalyst material is 3.33 eV. Thus, from the band position it can be proposed that {Mo368} is able to reduce CO2 as well as oxidize water simultaneously under UV-light source of 373 nm (Figure S3). The external quantum efficiency (EQE) of the system is measured by irradiating monochromatic LED light source of wavelength 365 nm (I = 67 mW/cm2) and 745 nm (I = 32 mW/cm2) where we find the EQE of 0.6 and 1.54 × 10−3%, respectively (SI).
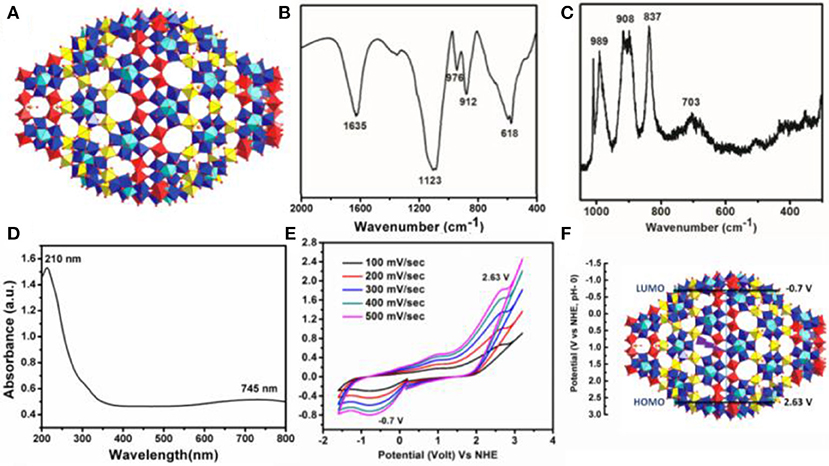
Figure 1. (A) Single crystal structure of {Mo368} where Red polyhedra denotes Oxygen, yellow denotes {Mo1} and blue with blue-turquoise pentagonal bipyramids denotes {Mo(Mo5)}, (B) FT-IR spectrum, (C) Raman Spectrum, (D) EAS spectrum, (E) Cyclic Voltammogram (Na2SO4 was used as electrolyte at a scan rate 100 mV/s), (F) HOMO and LUMO band position of {Mo368}.
To describe the detailed catalytic process of CO2 reduction in water, CO2 reduction under UV-light without addition of any photosensitizer as well as any organic sacrificial electron donor is performed. The reaction is carried out in an air tight quartz tube which is first purged with nitrogen to remove trace amount of oxygen from the reaction system. Later CO2 is purged for 3 h. The quartz tube is sealed and kept in a photo reactor for different intervals of time. CO2 undergoes reduction under aforementioned reaction condition, the reduced product is characterized first by GC-MS and further confirmed from 1H-NMR by solvent suppression method (water peak suppression; Figure 2A). These above results confirm that formic acid is formed from CO2 in our reaction condition. Further formic acid is characterized and quantified by HPLC against a standard 0.1 M formic acid solution. A peak at −0.6 V in cyclic voltammogram is also observed using saturated Ag/AgCl as a reference electrode (Figure 3A), which further confirms the formation of formic acid in the reaction mixture. Besides formic acid, trace amount of formaldehyde which is characterized by HPLC against 0.1 M formaldehyde solution is also found. To further check whether any gaseous reduced product is formed in the reaction, GC-MS is performed by injecting the gases from the reaction chamber and no CO, CH4, or any other reduced gaseous product is observed from the reaction mixture. Thus, in our present reaction we get formic acid selectively over other reduced product obtained from the reduction of CO2. Formic acid is formed from CO2 only and not from any other carbon impurity. To further prove the source of carbon in formic acid, same reaction is performed using 13CO2 as a reactant yielding H13COOH. The product is characterized by 13C-NMR (Figure 2B) taken before and after reaction and Raman spectroscopy (Figure S4). Prior to UV-light irradiation 13C-NMR gave strong signal at 125.5 ppm corresponding to 13CO2. After the completion of 6 h of photoreduction, 13CO2 signal is found to decrease and a new peak at 166.6 ppm corresponding to H13COOH is obtained (Tamaki et al., 2015). We also performed 1H-NMR after reducing 13CO2 enriched solution where a doublet (1JCH = 216 Hz) is found at 7.9 ppm which coupled with 13C atom (Figure 2D). These results indicate that formic acid is formed from CO2 and not from any other carbon source. This can be proved in a control experiment by recording HPLC of the light illuminated sample without purging of CO2. In that case, no trace of formic acid or formaldehyde is found in the reaction mixture. This indicates that formic acid and formaldehyde is obtained from CO2 only. During the photo catalytic CO2 reduction water also gets oxidized to oxygen simultaneously. Formation of oxygen is characterized by the YSI dissolved oxygen meter and GC-MS. The experiment is further repeated using mixture of O (isotopic purity = 97%) and O (1:8) where we found the corresponding masses at m/z = 32.01, 34.04 and 35.98. The mass ca. 32 corresponds to 16O16O whereas m/z = 34 and 36 resemble to 16O18O and 18O18O, respectively (Figure 2C) which confirms that oxygen is produced from oxidation of solvent water molecule. Besides, deuterium-labeling experiment was conducted using D2O (isotopic purity = 99.9%) and H2O as a solvent (3:2). We found that the deuterium was incorporated into formate moiety (m/z = 75), which was confirmed from mass spectrometry (Figures 2E,F). As exchangeable D is found in the reaction medium, it got incorporated during the formation of formic acid and later deuterium incorporated ethylformate is obtained after reaction with ethanol.
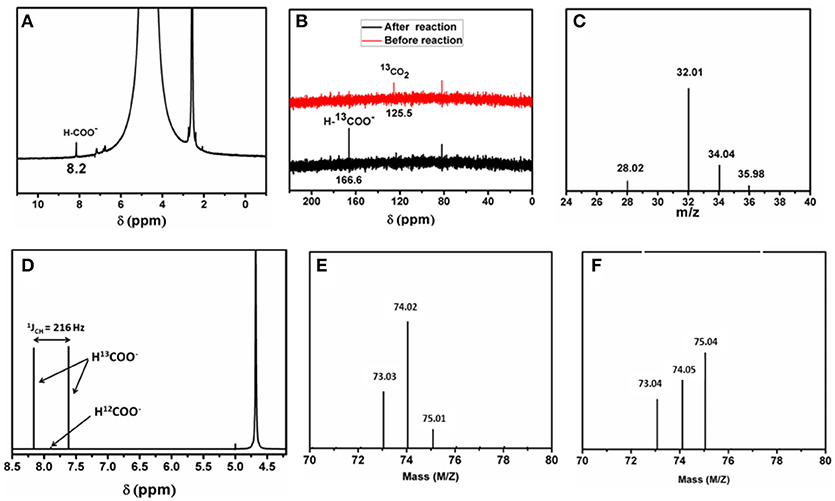
Figure 2. (A) Representative 1H-NMR of the product from photochemical CO2 reduction. (B) 13C-NMR spectrum of the reaction mixture before (red) and after (black) the reaction. (C) Full mass spectrum representing all the isotopes of the gaseous products (O2, 16O18O, 18O18O and N2). (D) 1H-NMR spectrum of the reaction mixture using 13CO2. (E) Mass of ethylformate (molecular peak) after reaction with H2O and (F) with D2Oand H2O mixture.
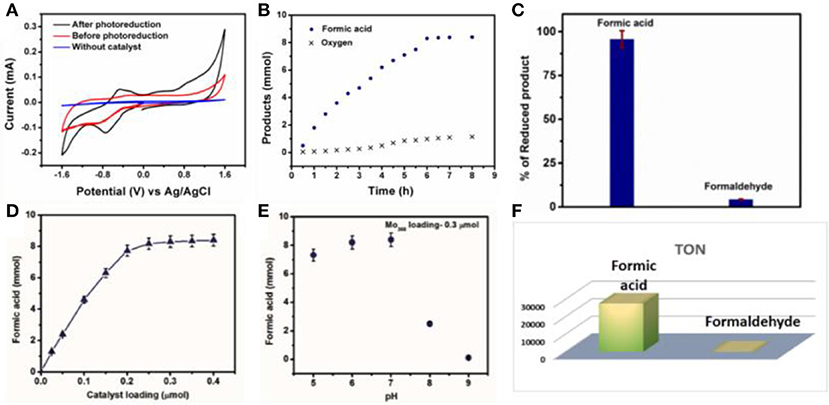
Figure 3. (A) Cyclic voltammogram of the reaction mixture, blue line corresponds to blank where there is neither CO2 nor catalyst, red line corresponds to CO2 addition without UV light illumination and black line corresponds to photo CO2 reduction in water using {Mo368} as a catalyst. (B) Time dependent formic acid and oxygen formation by photochemical carbon dioxide reduction using {Mo368} as a catalyst. (C) Formation of different reduced product using {Mo368}, and their respective percentage yield in total CO2 reduced product. (D) Effect of variation of {Mo368} loading on formation of formic acid. (E) pH dependent formic acid formation using {Mo368} as a catalyst. (F) TON of the formic acid and formaldehyde [Amount of product formed (mol)/amount of catalyst taken (mol)].
Further time dependent study reveals, 6 h is needed to complete the reduction of CO2 to formic acid. The TOF of the reaction is quite high 4,611 h−1 in water. The yield of the formic acid initially increases rapidly with time (Figure 3B). It increases almost linearly with time. After certain time of reaction, the rate of the increment of the formic acid formation with time decreases and reaction yield does not increase further after 6 h of the reaction. This indicates that the CO2 reduction reaction is complete within 6 h and same trend can be observed for oxygen evolution too (Figure 3B). Both the processes i.e., CO2 reduction and water oxidation are coupled which can be confirmed from Figure 3A where current increase occurs at 0.9 V vs. Ag/AgCl only after photoreduction. Furthermore, loading of the catalyst is varied in photochemical CO2 reduction in a controlled fashion (Figure 3D). Here, initially the yield of the formic acid increases almost linearly with the increasing loading of the catalyst and further after certain range of the loading of the catalyst the reaction yield becomes independent of the catalyst loading (Figure 3D). We have observed a maximum yield of 8.3 mmol of formic acid with a loading of 0.3 μmol of the catalyst with maximum turnover number of 27,666 (Figure 3F). This clearly indicates high reactivity of the {Mo368} unit. Other than formic acid, 37 μmol of formaldehyde is also obtained at the same loading of the catalyst under the same reaction condition. The selectivity of the formation of the formic acid with respect to the total CO2 reduced product is around 95.73% for formic acid and 4.27% for formaldehyde (Figure 3C). To see the effect of the proton concentration in the photo catalytic CO2 reduction reaction, pH is varied in the range from 5 to 9 in the course of the reaction. To do so, different buffer solutions are used (Acetate buffer was used to regulate the pH). pH dependent study reveals that the yield of formic acid is maximum at pH 7 (Figure 3E). The yield of the reaction is almost identical on moving toward acidic pH. But when we move toward the basic pH the yield of the reaction decreases drastically. This is due to the dissociation of {Mo368} in basic medium. Also, this is may be due to the fact that CO2 reduction is a proton coupled electron transfer (PCET) reaction, the reaction in basic pH, protons are quenched from the reaction medium therefore the yield of the reaction also decreases.
For carbon dioxide reduction reaction two major constituents, i.e., electrons and protons are required. As no sacrificial proton donor or electron donor is used in this case, there is a possibility that water acts as a source of both protons as well as electron in the reaction. To prove this observation, different sets of experiment were performed. Initially, when the same reaction was performed in dry DMF, due to lack of availability of protons, no trace of formic acid was detected indicating that water is playing a crucial role in carbon dioxide reduction reaction under the prevalent reaction conditions (Figure 4A). Further to prove the role of the water in the photo catalytic condition, different set of reactions with varying ratio of water and DMF in the reaction mixture was carried out keeping the total volume of the reaction mixture constant (Figure 4A). From the experimental result it can be concluded that with increasing loading of water in the reaction the yield of formic acid increases linearly, which supports the role of water as proton donor.
The dependency of water on CO2 reduction reaction is further obtained from pH dependent study. As, carbon dioxide reduction is proton dependent process (Equation 3), the yield of the reaction should increase on increasing the proton concentration in the reaction medium. But in our present case we observe a decrease in reduced product concentration upon going from neutral pH to acidic pH. This result indicates that another component of CO2 reduction reaction equilibrium i.e., electron concentration may vary with the change in the pH of the reaction. There are generally two electron sources present in reaction system: one is the catalyst material itself and another is the water. Now if the cluster were to act as electron donor in the reaction then it would degrade in the solution. If it were the situation then a decrease in the absorbance of the catalyst with increasing time of illumination may be observed. But the absorption spectrum of cluster remains unchanged throughout the reaction (Figure 4B). Moreover, in all pH variation reactions the same amount of catalyst was used resulting in a constant electronic concentration in the reaction. On the other hand, if the reaction equilibrium totally depended on proton concentration of the reaction system then the yield should have increased at lower pH. However, this does not happen in our reaction system. Hence from the above two observations it can be concluded that the catalyst material does not act as a source of electrons in the photo chemical CO2 reduction reaction and water must act as an electron source in the reaction.
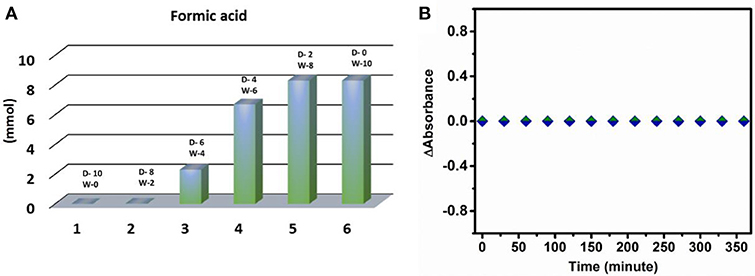
Figure 4. (A) Variation of DMF and water content in reaction mixture (D-DMF, W-water, all values given in ml unit). (B) Plot of change of absorbance of {Mo368} with time vs. reaction time.
As already discussed, oxygen formation was detected in the system during CO2 reduction which proves that the oxidation of water releases electrons into the system. As it is known that the water oxidation reaction depends on the pH of the medium and it increases with increasing pH of the reaction i.e., ongoing from the acidic to basic pH water oxidation increases. As water oxidation increases at higher pH electron concentration also increases which will facilitate the reduction process. Thus, CO2 reduction should increase at higher pH. It is also observed in our present reaction system that CO2 reduction increases with increasing pH (Up to pH 7, as at basic pH the catalyst dissociates). Thus, from the above two observations we can conclude that water only acts as a sacrificial electron donor in the reaction and photo chemical CO2 reduction depends on photo-chemical water oxidation reaction which also takes place parallelly in the reaction system. Thus, a maximum yield of formic acid is obtained in neutral pH as compared to acidic pH.
To investigate the active catalyst species in the reaction, the reaction is repeated with the precursor of {Mo368} i.e., with sodium molybdate and no reduced product is found in the reaction. Thus, from this result, it can be concluded that the giant cluster {Mo368} is only responsible for CO2 reduction reaction and not a single molybdenum unit. Cluster cage thus plays a crucial role for the reaction. It is already mentioned that {Mo368} is a photoactive materialand it absorbs UV-light of 373 nm wave length to generate holes (h+) and electrons (e−) in the system. These holes can oxidize water to generate electrons, protons and oxygen in the medium. The electrons and protons so generated reduce CO2 to formic acid and formaldehyde. This also explains the high photo catalytic activity of {Mo368}. {Mo368} comprises of different small molybdenum based units i.e., {MoVI()}, {}, {}, respectively. We believe that upon photo excitation, {MoVI()} goes to excited state and forms {MoVI()}*. This species has potential to oxidize water to liberate oxygen and release protons and electrons. On the other hand, the {Mo2} unit and {Mo1} unit plays a crucial role for photochemical CO2 reduction. It has been already shown by Müller group that CO2 can coordinate with {Mo2} units of the giant molybdenum based polyoxometalates (Garai et al., 2012; Bandeira et al., 2015). Thus, in our present case it is also reasonable to postulate that CO2 can co-ordinate with {Mo2} unit. It is also observed that there is change in cyclic voltammogram of {Mo368} upon purging of CO2 in {Mo368} solution (Figure S2). Further this coordinated CO2 unit can be reduced by electrons and protons present in the reaction medium. Possibly CO2 also can coordinate with {Mo1} unit and reduction of coordinated CO2 takes place in a synergistic fashion. We believe that adsorbed CO2 converted to C. The activation barrier of this reduction is lowered by the cluster. Later this species accept proton to generate carboxyhydroxyl intermediate species which participates in PCET process to form formic acid. Due to direct attachment, a large number of CO2 molecules are reduced for every molecule of {Mo368}, and hence the cluster shows such high photocatalytic activity.
The stability of the catalyst is discussed under prevalent photo catalytic conditions. After reaction the catalyst is recovered by slow evaporation of solvent. In this present case it is shown that the {Mo368} is stable under the photocatalytic condition using different techniques. FT-IR spectrum of {Mo368} after completion of the reaction shows peaks at 1632, 1124, 985, 916, 615 cm−1, respectively which are almost similar to that of the solid catalyst. This indicates that the cluster is stable under the reaction conditions (Figure 5A). Moreover, the IVCT bands responsible for the Mo(V)->Mo(VI) transitions do not change with the reaction and it further indicates that there is no change in the catalyst composition and that the catalyst is stable under the reaction conditions. Similar result is also observed from Raman spectrum of catalyst which also shows the catalyst remains intact after the photochemical CO2 reduction (Figure 5B). Also note that no presence of particulate matter is detected from DLS in this system during catalysis which indicates that the catalyst is homogeneous. Hence our catalyst is a stable homogeneous catalyst which reduces CO2 to formic acid in water.
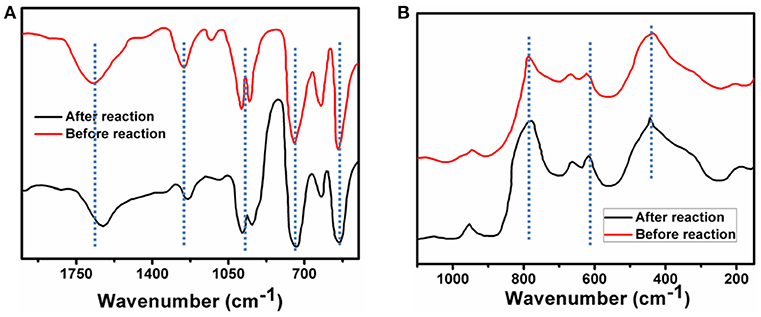
Figure 5. (A) FT-IR spectrum of {Mo368}, black line corresponds to FT-IR spectrum of before reaction and brown line corresponds to FT-IR spectrum of after reaction. (B) Raman spectrum of {Mo368}, black line corresponds to Raman spectrum of before reaction and brown line corresponds to Raman spectrum of after reaction.
Conclusion
Photochemical reduction of CO2 to formic acid in water using molybdenum based giant polyoxometalate {Mo368} molecular catalyst is reported. The photocatalytic system shows excellent selectivity for formic acid production (95.73%) with high TON (27,666) and TOF (4,419 h−1); which is quite high in its class. The presence of different Mo based sub units in the cluster is responsible for the exceptional activity of the catalyst. As the system is photoactive, no external photosensitizer is added and water solvent acts as electron donor making the whole process environmentally benign and self-sustained. We believe that this work can lead to the development of a class of highly efficient homogeneous CO2 reduction catalysts based on water soluble polyoxometalates.
Author Contributions
SD, TB and SB contributed equally. SD, TB and SB performed the experiments. SS assisted in those experiments. RP carried out GC-MS and HPLC experiments. SR designed the project, analyzed the results and wrote the paper with inputs from all other co-authors.
Conflict of Interest Statement
The authors declare that the research was conducted in the absence of any commercial or financial relationships that could be construed as a potential conflict of interest.
Acknowledgments
The authors thank Prof. Dr. Abhishek Dey, of IACS Kolkata, for his help with 13CO2 experiments. SR gratefully acknowledges the start-up grant and FIRE and PRIS grant from IISER Kolkata, India, and grants from CCNU, and NSFC, P. R. China. TB acknowledges IISER Kolkata for his fellowship. SS thanks SERB for financial assistance (PDF/2017/000676) and SB acknowledges UGC for fellowship.
Supplementary Material
The Supplementary Material for this article can be found online at: https://www.frontiersin.org/articles/10.3389/fchem.2018.00514/full#supplementary-material
References
Agarwal, A. S., Zhai, Y., Hill, D., and Sridhar, N. (2011). The electrochemical reduction of carbon dioxide to formate/formic acid: engineering and economic feasibility. ChemSusChem 4, 1301–1310. doi: 10.1002/cssc.201100220
Amatore, C., and Saveant, J. M. (1981). Mechanism and kinetic characteristics of the electrochemical reduction of carbon dioxide in media of low proton availability. J. Am. Chem. Soc. 103, 5021–5023. doi: 10.1021/ja00407a008
Arai, T., Sato, S., Kajino, T., and Morikawa, T. (2013). Solar CO2 reduction using H2O by a semiconductor/metal-complex hybrid photocatalyst: enhanced efficiency and demonstration of a wireless system using SrTiO3 photoanodes. Energy Environ. Sci. 6, 1274–1282. doi: 10.1039/c3ee24317f
Asadi, M., Kumar, B., Behranginia, A., Rosen, B. A., Baskin, A., Repnin, N., et al. (2014). Robust carbon dioxide reduction on molybdenum disulphide edges. Nat. Commun. 5, 4470. doi: 10.1038/ncomms5470
Bai, X., Chen, W., Zhao, C., Li, S., Song, Y., Ge, R., et al. (2017). Exclusive formation of formic acid from CO2 electroreduction by tunable Pd-Sn alloy. Angew. Chem. 129, 12387–12391. doi: 10.1002/ange.201707098
Bandeira, N. A., Garai, S., Müller, A., and Bo, C. (2015). The mechanism of CO2 hydration: a porous metal oxide nanocapsule catalyst can mimic the biological carbonic anhydrase role. Chem. Commun. 51, 15596–15599. doi: 10.1039/C5CC06423F
Barman, S., Das, S., Sreejith, S., Garai, S., Pochamoni, R., and Roy, S. (2018). Selective light driven reduction of CO2 to HCOOH in water using a {MoV9}n (n = 1332–3600) based soft-oxometalate (SOM). Chem. Commun. 54, 2369–2372. doi: 10.1039/C7CC09520A
Barton, E. E., Rampulla, D. M., and Bocarsly, A. B. (2008). Selective solar-driven reduction of CO2 to methanol using a catalyzed p-GaP based photoelectrochemical cell. J. Am. Chem. Soc. 130, 6342–6344. doi: 10.1021/ja0776327
Beley, M., Collin, J.-P., Ruppert, R., and Sauvage, J.-P. (1984). Nickel (II)-cyclam: an extremely selective electrocatalyst for reduction of CO2 in water. J. Chem. Soc. Chem. Commun. 1315–1316. doi: 10.1039/c39840001315
Blondiaux, E., Pouessel, J., and Cantat, T. (2014). Carbon dioxide reduction to methylamines under metal-free conditions. Angew. Chem. Int. Ed. 53, 12186–12190. doi: 10.1002/anie.201407357
Bontemps, S., Vendier, L., and Sabo-Etienne, S. (2012). Borane-mediated carbon dioxide reduction at ruthenium: formation of C1 and C2 Compounds. Angew. Chem. 124, 1703–1706. doi: 10.1002/ange.201107352
Boston, D. J., Xu, C., Armstrong, D. W., and MacDonnell, F. M. (2013). Photochemical reduction of carbon dioxide to methanol and formate in a homogeneous system with pyridinium catalysts. J. Am. Chem. Soc. 135, 16252–16255. doi: 10.1021/ja406074w
Castro-Rodriguez, I., and Meyer, K. (2005). Carbon dioxide reduction and carbon monoxide activation employing a reactive uranium (III) complex. J. Am. Chem. Soc. 127, 11242–11243. doi: 10.1021/ja053497r
Chen, D., Sahasrabudhe, A., Wang, P., Dasgupta, A., Yuan, R., and Roy, S. (2013). Synthesis and properties of a novel quarternerized imidazolium [α-PW12O40]3− salt as a recoverable photo-polymerization catalyst. Dalton Trans. 42, 10587–10596. doi: 10.1039/c3dt32916j
Chen, L., Guo, Z., Wei, X.-G., Gallenkamp, C., Bonin, J., Anxolabéhére-Mallart, E, et al. (2015). Molecular catalysis of the electrochemical and photochemical reduction of CO2 with earth-abundant metal complexes. Selective production of CO vs HCOOH by switching of the metal center. J. Am. Chem. Soc. 137, 10918–10921. doi: 10.1021/jacs.5b06535
Cokoja, M., Bruckmeier, C., Rieger, B., Herrmann, W. A., and Kühn, F. E. (2011). Transformation of carbon dioxide with homogeneous transition-metal catalysts: a molecular solution to a global challenge? Angew. Chem. Int. Ed. 50, 8510–8537. doi: 10.1002/anie.201102010
Costentin, C., Robert, M., and Savéant, J.-M. (2013). Catalysis of the electrochemical reduction of carbon dioxide. Chem. Soc. Rev. 42, 2423–2436. doi: 10.1039/C2CS35360A
Crabtree, G. W., and Lewis, N. S. (2007). Solar energy conversion. Phys. Today 60, 37–42. doi: 10.1063/1.2718755
Daniel, M. C., and Astruc, D. (2004). Gold nanoparticles: assembly, supramolecular chemistry, quantum-size-related properties, and applications toward biology, catalysis, and nanotechnology. Chem. Rev. 104, 293–346. doi: 10.1021/cr030698+
Das, K., and Roy, S. (2015). Direct synthesis of controlled-size nanospheres inside nanocavities of self-organized photopolymerizing soft oxometalates [PW12O40]n (n = 1100–7500). Chem. Asian J. 10, 1884–1891. doi: 10.1002/asia.201500336
Das, S., Biswas, S., Balaraju, T., Barman, S., Pochamoni, R., and Roy, S. (2016). Photochemical reduction of carbon dioxide coupled with water oxidation using various soft-oxometalate (SOM) based catalytic systems. J. Mater. Chem. A 4, 8875–8887. doi: 10.1039/C6TA02825J
Das, S., Lai, D., Mallick, A., and Roy, S. (2016a). Photo redox mediated inexpensive one-pot synthesis of 1,4-diphenyl substituted butane-1,4-dione from styrene using polyoxometalate as a catalyst. ChemistrySelect 1, 691–695. doi: 10.1002/slct.201500052
Das, S., Misra, A., and Roy, S. (2016b). Enhancement of photochemical heterogeneous water oxidation by a manganese based soft oxometalate immobilized on a graphene oxide matrix. New J. Chem. 40, 994–1003. doi: 10.1039/C5NJ01099C
Das, S., and Roy, S. (2016). A newly designed softoxometalate [Bmim]2[Dmim][α-PW12O40]@ hydrocalumite that controls the chain length of polyacrylic acid in presence of light. RSC Adv. 6, 37586–37590. doi: 10.1039/C6RA02685K
Dhakshinamoorthy, A., Navalon, S., Corma, A., and Garcia, H. (2012). Photocatalytic CO2 reduction by TiO2 and related titanium containing solids. Energy Environ. Sci. 5, 9217–9233. doi: 10.1039/c2ee21948d
Finn, C., Schnittger, S., Yellowlees, L. J., and Love, J. B. (2012). Molecular approaches to the electrochemical reduction of carbon dioxide. Chem. Commun. 48, 1392–1399. doi: 10.1039/C1CC15393E
Fisher, B. J., and Eisenberg, R. (1980). Electrocatalytic reduction of carbon dioxide by using macrocycles of nickel and cobalt. J. Am. Chem. Soc. 102, 7361–7363. doi: 10.1021/ja00544a035
Gao, D., Zhou, H., Wang, J., Miao, S., Yang, F., Wang, G., et al. (2015). Size-dependent electrocatalytic reduction of CO2 over Pd nanoparticles. J. Am. Chem. Soc. 137, 4288–4291. doi: 10.1021/jacs.5b00046
Garai, S., Haupt, E. T., Bögge, H., Merca, A., and Müller, A. (2012). Picking up 30 CO2 molecules by a porous metal oxide capsule based on the same number of receptors. Angew. Chem. 124, 10680–10683. doi: 10.1002/ange.201204089
Halmann, M. (1978). Photoelectrochemical reduction of aqueous carbon dioxide on p-type gallium phosphide in liquid junction solar cells. Nature 275, 115–116.
Hatch, M. D. (1976). The C4 Pathway of Photosynthesis: Mechanism and Function. Baltimore, MD: University Park Press.
Hawecker, J., Lehn, J.-M., and Ziessel, R. (1984). Electrocatalytic reduction of carbon dioxide mediated by Re (bipy)(CO)3Cl (bipy = 2,2′-bipyridine). J. Chem. Soc. Chem. Commun. 328–330. doi: 10.1039/C39840000328
Herrero, C., Quaranta, A., El Ghachtouli, S., Vauzeilles, B., Leibl, W., and Aukauloo, A. (2014). Carbon dioxide reduction via light activation of a ruthenium–Ni (cyclam) complex. PCCP 16, 12067–12072. doi: 10.1039/c3cp54946a
Hoel, M., and Kverndokk, S. (1996). Depletion of fossil fuels and the impacts of global warming. Res. Energy Econ. 18, 115–136. doi: 10.1016/0928-7655(96)00005-X
Hoffert, M. I., Caldeira, K., Benford, G., Criswell, D. R., Green, C., Herzog, H., et al. (2002). Advanced technology paths to global climate stability: energy for a greenhouse planet. Science 298, 981–987. doi: 10.1126/science.1072357
Höök, M., and Tang, X. (2013). Depletion of fossil fuels and anthropogenic climate change—a review. Energy Policy 52, 797–809. doi: 10.1016/j.enpol.2012.10.046
Hori, Y., Murata, A., and Takahashi, R. (1989). Formation of hydrocarbons in the electrochemical reduction of carbon dioxide at a copper electrode in aqueous solution. J. Chem. Soc. Faraday Trans. 1 85, 2309–2326. doi: 10.1039/f19898502309
Iizuka, K., Wato, T., Miseki, Y., Saito, K., and Kudo, A. (2011). Photocatalytic reduction of carbon dioxide over Ag cocatalyst-loaded ALa4Ti4O15 (A = Ca, Sr, and Ba) using water as a reducing reagent. J. Am. Chem. Soc. 133, 20863–20868. doi: 10.1021/ja207586e
Ikemiya, N., Natsui, K., Nakata, K., and Einaga, Y. (2018). Long-term continuous conversion of CO2 to formic acid using boron-doped diamond electrodes. ACS Sustain. Chem. Eng. 6, 8108–8112. doi: 10.1021/acssuschemeng.8b00793.
Ikeyama, S., and Amao, Y. (2018). The effect of the functional ionic group of the viologen derivative on visible-light driven CO2 reduction to formic acid with the system consisting of water-soluble zinc porphyrin and formate dehydrogenase. Photochem. Photobiol. Sci. 17, 60–68. doi: 10.1039/C7PP00277G
Iwase, A., Yoshino, S., Takayama, T., Ng, Y. H., Amal, R., and Kudo, A. (2016). Water splitting and CO2 reduction under visible light irradiation using Z-scheme systems consisting of metal sulfides, CoOx-Loaded BiVO4, and a reduced graphene oxide electron mediator. J. Am. Chem. Soc. 138, 10260–10264. doi: 10.1021/jacs.6b05304
Khenkin, A. M., Efremenko, I., Weiner, L., Martin, J. M., and Neumann, R. (2010). Photochemical reduction of carbon dioxide catalyzed by a ruthenium-substituted polyoxometalate. Chem. Eur. J. 16, 1356–1364. doi: 10.1002/chem.200901673
Kim, C., Jeon, H. S., Eom, T., Jee, M. S., Kim, H., Friend, C. M., et al. (2015). Achieving selective and efficient electrocatalytic activity for CO2 reduction using immobilized silver nanoparticles. J. Am. Chem. Soc. 137, 13844–13850. doi: 10.1021/jacs.5b06568
Kim, W., Yuan, G., McClure, B. A., and Frei, H. (2014). Light induced carbon dioxide reduction by water at binuclear ZrOCo(II) unit coupled to Ir oxide nanocluster catalyst. J. Am. Chem. Soc. 136, 11034–11042. doi: 10.1021/ja504753g
Kornienko, N., Zhao, Y., Kley, C. S., Zhu, C., Kim, D., Lin, S., et al. (2015). Metal–organic frameworks for electrocatalytic reduction of carbon dioxide. J. Am. Chem. Soc. 137, 14129–14135. doi: 10.1021/jacs.5b08212
Kortlever, R., Peters, I., Koper, S., and Koper, M. T. (2015). Electrochemical CO2 reduction to formic acid at low overpotential and with high faradaic efficiency on carbon-supported bimetallic Pd–Pt nanoparticles. ACS Catal. 5, 3916–3923. doi: 10.1021/acscatal.5b00602
Kou, Y., Nabetani, Y., Masui, D., Shimada, T., Takagi, S., Tachibana, H., et al. (2014). Direct detection of key reaction intermediates in photochemical CO2 reduction sensitized by a rhenium bipyridine complex. J. Am. Chem. Soc. 136, 6021–6030. doi: 10.1021/ja500403e
Kuhl, K. P., Cave, E. R., Abram, D. N., and Jaramillo, T. F. (2012). New insights into the electrochemical reduction of carbon dioxide on metallic copper surfaces. Energy Environ. Sci. 5, 7050–7059. doi: 10.1039/c2ee21234j
Kuriki, R., Matsunaga, H., Nakashima, T., Wada, K., Yamakata, A., Ishitani, O., et al. (2016). Nature-inspired, highly durable CO2 reduction system consisting of a binuclear ruthenium (II) complex and an organic semiconductor using visible light. J. Am. Chem. Soc. 138, 5159–5170. doi: 10.1021/jacs.6b01997
Kuriki, R., Yamamoto, M., Higuchi, K., Yamamoto, Y., Akatsuka, M., Lu, D., et al. (2017). Robust binding between carbon nitride nanosheets and a binuclear ruthenium (II) complex enabling durable, selective CO2 reduction under visible light in aqueous solution. Angew. Chem. Int. Ed. 56, 4867–4871. doi: 10.1002/anie.201701627
Laitar, D. S., Müller, P., and Sadighi, J. P. (2005). Efficient homogeneous catalysis in the reduction of CO2 to CO. J. Am. Chem. Soc. 127, 17196–17197. doi: 10.1021/ja0566679
Langer, R., Diskin-Posner, Y., Leitus, G., Shimon, L. J., Ben-David, Y., and Milstein, D. (2011). Low-pressure hydrogenation of carbon dioxide catalyzed by an iron pincer complex exhibiting noble metal activity. Angew. Chem. Int. Ed. 50, 9948–9952. doi: 10.1002/anie.201104542
Li, F., Zhao, S.-F., Chen, L., Khan, A., MacFarlane, D. R., and Zhang, J. (2015). Polyethylenimine promoted electrocatalytic reduction of CO2 to CO in aqueous medium by graphene-supported amorphous molybdenum sulphide. Energy Environ. Sci. 9, 216–223. doi: 10.1039/C5EE02879E
Li, J., and Zhang, J. Z. (2009). Optical properties and applications of hybrid semiconductor nanomaterials. Coord. Chem. Rev. 253, 3015–3041. doi: 10.1016/j.ccr.2009.07.017
Lin, S., Diercks, C. S., Zhang, Y.-B., Kornienko, N., Nichols, E. M., Zhao, Y., et al. (2015). Covalent organic frameworks comprising cobalt porphyrins for catalytic CO2 reduction in water. Science 349, 1208–1213. doi: 10.1126/science.aac8343
Liu, S., Xia, J., and Yu, J. (2015). Amine-functionalized titanate nanosheet-assembled yolk@ shell microspheres for efficient cocatalyst-free visible-light photocatalytic CO2 reduction. ACS Appl. Mater. Interfaces 7, 8166–8175. doi: 10.1021/acsami.5b00982
Low, J., Yu, J., and Ho, W. (2015). Graphene-based photocatalyst for CO2 reduction to solar fuel. J. Phys. Chem. Lett. 6, 4244–4251. doi: 10.1021/acs.jpclett.5b01610
Lu, Q., Rosen, J., Zhou, Y., Hutchings, G. S., Kimmel, Y. C., Chen, J. G., et al. (2014). A selective and efficient electrocatalyst for carbon dioxide reduction. Nat. Commun. 5, 3242. doi: 10.1038/ncomms4242
Lu, W., Jia, B., Cui, B., Zhang, Y., Yao, K., Zhao, Y., et al. (2017). Efficient photoelectrochemical reduction of CO2 to formic acid with functionalized ionic liquid as absorbent and electrolyte. Angew. Chem. Int. Ed. 129, 12013–12016. doi: 10.1002/ange.201703977
Maginn, E. J. (2010). What to do with CO2. J. Phys. Chem. Lett. 1, 3478–3479. doi: 10.1021/jz101582c
Mandal, S. K., and Roesky, H. W. (2011). Group 14 hydrides with low valent elements for activation of small molecules. Acc. Chem. Res. 45, 298–307. doi: 10.1021/ar2001759
Marszewski, M., Cao, S., Yu, J., and Jaroniec, M. (2015). Semiconductor-based photocatalytic CO2 conversion. Mater. Horiz. 2, 261–278. doi: 10.1039/C4MH00176A
Matlachowski, C., and Schwalbe, M. (2015). Photochemical CO2-reduction catalyzed by mono-and dinuclear phenanthroline-extended tetramesityl porphyrin complexes. Dalton Trans. 44, 6480–6489. doi: 10.1039/C4DT03846K
Matsuoka, S., Yamamoto, K., Ogata, T., Kusaba, M., Nakashima, N., Fujita, E., et al. (1993). Efficient and selective electron mediation of cobalt complexes with cyclam and related macrocycles in the p-terphenyl-catalyzed photoreduction of carbon dioxide. J. Am. Chem. Soc. 115, 601–609. doi: 10.1021/ja00055a032
Meinshausen, M., Meinshausen, N., Hare, W., Raper, S. C., Frieler, K., Knutti, R., et al. (2009). Greenhouse-gas emission targets for limiting global warming to 2°C. Nature 458, 1158–1162. doi: 10.1038/nature08017
Mikkelsen, M., Jørgensen, M., and Krebs, F. C. (2010). The teraton challenge. A review of fixation and transformation of carbon dioxide. Energy Environ. Sci. 3, 43–81. doi: 10.1039/B912904A
Morris, A. J., Meyer, G. J., and Fujita, E. (2009). Molecular approaches to the photocatalytic reduction of carbon dioxide for solar fuels. Acc. Chem. Res. 42, 1983–1994. doi: 10.1021/ar9001679
Müller, A., Beckmann, E., Bögge, H., Schmidtmann, M., and Dress, A. (2002). Inorganic chemistry goes protein size: a Mo368 nano-hedgehog initiating nanochemistry by symmetry breaking. Angew. Chem. Int. Ed. 41, 1162-1167. doi: 10.1002/1521-3773(20020402)41:7<1162::AID-ANIE1162>3.0.CO;2-8
Müller, A., Rehder, D., Haupt, E. T., Merca, A., Bögge, H., Schmidtmann, M., et al. (2004). Artificial cells: temperature-dependent, reversible Li+-ion uptake/release equilibrium at metal oxide nanocontainer pores. Angew. Chem. Int. Ed. 43, 4466–4470. doi: 10.1002/anie.200453762
Müller, A., and Roy, S. (2003). En route from the mystery of molybdenum blue via related manipulatable building blocks to aspects of materials science. Coord. Chem. Rev. 245, 153–166. doi: 10.1016/S0010-8545(03)00110-3
Nakada, A., Koike, K., Maeda, K., and Ishitani, O. (2016). Highly efficient visible-light-driven CO2 reduction to CO using a Ru (ii)–Re (i) supramolecular photocatalyst in an aqueous solution. Green Chem. 18, 139–143. doi: 10.1039/C5GC01720C
Nakada, A., Koike, K., Nakashima, T., Morimoto, T., and Ishitani, O. (2015). Photocatalytic CO2 reduction to formic acid using a Ru (II)–Re (I) supramolecular complex in an aqueous solution. Inorg. Chem. 54, 1800–1807. doi: 10.1021/ic502707t
Ohtsu, H., and Tanaka, K. (2012). An organic hydride transfer reaction of a ruthenium NAD model complex leading to carbon dioxide reduction. Angew. Chem. Int. Ed. 51, 9792–9795. doi: 10.1002/anie.201204348
Rankin, M. A., and Cummins, C. C. (2010). Carbon dioxide reduction by terminal tantalum hydrides: formation and isolation of bridging methylene diolate complexes. J. Am. Chem. Soc. 132, 10021–10023. doi: 10.1021/ja104761n
Roberts, F. S., Kuhl, K. P., and Nilsson, A. (2015). High selectivity for ethylene from carbon dioxide reduction over copper nanocube electrocatalysts. Angew. Chem. 127, 5268–5271. doi: 10.1002/ange.201412214
Rosas-Hernándeza, A., Junge, H., and Beller, M. (2015). Photochemical reduction of carbon dioxide to formic acid using ruthenium (II)-based catalysts and visible light. ChemCatChem 7, 3316–3321. doi: 10.1002/cctc.201500494
Roy, B., Arya, M., Thomas, P., Jürgschat, J. K., Venkata Rao, K., Banerjee, A., et al. (2013). Self-assembly of mesoscopic materials to form controlled and continuous patterns by thermo-optically manipulated laser induced microbubbles. Langmuir 29, 14733–14742. doi: 10.1021/la402777e
Roy, S., Bossers, L. C., Meeldijk, H. J., Kuipers, B. W., and Kegel, W. K. (2008). Directed synthesis of stable large polyoxomolybdate spheres. Langmuir 24, 666–669. doi: 10.1021/la703467d
Roy, S., Mourad, M. C., and Rijneveld-Ockers, M. T. (2007b). Synthesis and characterization of large surface hexagonal polyoxometalate platelets. Langmuir 23, 399–401. doi: 10.1021/la062831+
Roy, S., Rijneveld-Ockers, M. T., Groenewold, J., Kuipers, B. W., Meeldijk, H., and Kegel, W. K. (2007a). Spontaneous formation of micrometer-size inorganic peapods. Langmuir 23, 5292–5295. doi: 10.1021/la700373h
Sadique, A. R., Brennessel, W. W., and Holland, P. L. (2008). Reduction of CO2 to CO using low-coordinate iron: formation of a four-coordinate iron dicarbonyl complex and a bridging carbonate complex. Inorg. Chem. 47, 784–786. doi: 10.1021/ic701914m
Sakakura, T., Choi, J.-C., and Yasuda, H. (2007). Transformation of carbon dioxide. Chem. Rev. 107, 2365–2387. doi: 10.1021/cr068357u
Sato, S., Arai, T., Morikawa, T., Uemura, K., Suzuki, T. M., Tanaka, H., et al. (2011). Selective CO2 conversion to formate conjugated with H2O oxidation utilizing semiconductor/complex hybrid photocatalysts. J. Am. Chem. Soc. 133, 15240–15243. doi: 10.1021/ja204881d
Sato, S., Morikawa, T., Kajino, T., and Ishitani, O. (2013). A highly efficient mononuclear iridium complex photocatalyst for CO2 reduction under visible light. Angew. Chem. 125, 1022–1026. doi: 10.1002/ange.201206137
Schmeier, T. J., Dobereiner, G. E., Crabtree, R. H., and Hazari, N. (2011). Secondary coordination sphere interactions facilitate the insertion step in an iridium (III) CO2 reduction catalyst. J. Am. Chem. Soc. 133, 9274–9277. doi: 10.1021/ja2035514
Schwartzberg, A. M., and Zhang, J. Z. (2008). Novel optical properties and emerging applications of metal nanostructures. J. Phys. Chem. C 112, 10323–10337. doi: 10.1021/jp801770w
Sekizawa, K., Maeda, K., Domen, K., Koike, K., and Ishitani, O. (2013). Artificial Z-scheme constructed with a supramolecular metal complex and semiconductor for the photocatalytic reduction of CO2. J. Am. Chem. Soc. 135, 4596–4599. doi: 10.1021/ja311541a
Studt, F., Sharafutdinov, I., Abild-Pedersen, F., Elkjær, C. F., Hummelshøj, J. S., Dahl, S., et al. (2014). Discovery of a Ni-Ga catalyst for carbon dioxide reduction to methanol. Nat. Chem 6, 320–324. doi: 10.1038/nchem.1873
Sullivan, B. P., Krist, K., and Guard, H. (2012). Electrochemical and Electrocatalytic Reactions of Carbon Dioxide. Amsterdam: Elsevier.
Sypaseuth, F. D., Matlachowski, C., Weber, M., Schwalbe, M., and Tzschucke, C. C. (2015). Electrocatalytic carbon dioxide reduction by using cationic pentamethylcyclopentadienyl–iridium complexes with unsymmetrically substituted bipyridine ligands. Chem. Eur. J. 21, 6564–6571. doi: 10.1002/chem.201404367
Takeda, H., Koike, K., Inoue, H., and Ishitani, O. (2008). Development of an efficient photocatalytic system for CO2 reduction using rhenium (I) complexes based on mechanistic studies. J. Am. Chem. Soc. 130, 2023–2031. doi: 10.1021/ja077752e
Tamaki, Y., Koike, K., and Ishitani, O. (2015). Highly efficient, selective, and durable photocatalytic system for CO2 reduction to formic acid. Chem. Sci. 6, 7213–7221. doi: 10.1039/C5SC02018B
Tamaki, Y., Morimoto, T., Koike, K., and Ishitani, O. (2012). Photocatalytic CO2 reduction with high turnover frequency and selectivity of formic acid formation using Ru (II) multinuclear complexes. Proc. Natl. Acad. Sci. U.S.A. 109, 15673–15678. doi: 10.1073/pnas.1118336109
Tornow, C. E., Thorson, M. R., Ma, S., Gewirth, A. A., and Kenis, P. J. (2012). Nitrogen-based catalysts for the electrochemical reduction of CO2 to CO. J. Am. Chem. Soc. 134, 19520–19523. doi: 10.1021/ja308217w
Wang, C., Xie, Z., deKrafft, K. E., and Lin, W. (2011). Doping metal–organic frameworks for water oxidation, carbon dioxide reduction, and organic photocatalysis. J. Am. Chem. Soc. 133, 13445–13454. doi: 10.1021/ja203564w
Wang, W., Wang, S., Ma, X., and Gong, J. (2011). Recent advances in catalytic hydrogenation of carbon dioxide. Chem. Soc. Rev. 40, 3703–3727. doi: 10.1039/c1cs15008a
Wang, Y., Wang, F., Chen, Y., Zhang, D., Li, B., Kang, S., et al. (2014). Enhanced photocatalytic performance of ordered mesoporous Fe-doped CeO2 catalysts for the reduction of CO2 with H2O under simulated solar irradiation. Appl. Catal. B 147, 602–609. doi: 10.1016/j.apcatb.2013.09.036
Wesselbaum, S., vom Stein, T., Klankermayer, J., and Leitner, W. (2012). Hydrogenation of carbon dioxide to methanol by using a homogeneous ruthenium–phosphine catalyst. Angew. Chem. 124, 7617–7620. doi: 10.1002/ange.201202320
Whipple, D. T., and Kenis, P. J. (2010). Prospects of CO2 utilization via direct heterogeneous electrochemical reduction. J. Phys. Chem. Lett. 1, 3451–3458. doi: 10.1021/jz1012627
Xi, G., Ouyang, S., Li, P., Ye, J., Ma, Q., Su, N., et al. (2012). Ultrathin W18O49 Nanowires with Diameters below 1 nm: synthesis, near-infrared absorption, photoluminescence, and photochemical reduction of carbon dioxide. Angew. Chem. Int. Ed. 51, 2395–2399. doi: 10.1002/anie.201107681
Yadav, R. K., Baeg, J.-O., Oh, G. H., Park, N.-J., Kong, K.-J., Kim, J., et al. (2012). A photocatalyst–enzyme coupled artificial photosynthesis system for solar energy in production of formic acid from CO2. J. Am. Chem. Soc. 134, 11455–11461. doi: 10.1021/ja3009902
Yoshitomi, F., Sekizawa, K., Maeda, K., and Ishitani, O. (2015). Selective formic acid production via CO2 reduction with visible light using a hybrid of a perovskite tantalum oxynitride and a binuclear ruthenium (II) complex. ACS Appl. Mater. Interfaces 7, 13092–13097. doi: 10.1021/acsami.5b03509
Yu, J., Low, J., Xiao, W., Zhou, P., and Jaroniec, M. (2014). Enhanced photocatalytic CO2-reduction activity of anatase TiO2 by coexposed {001} and {101} facets. J. Am. Chem. Soc. 136, 8839–8842. doi: 10.1021/ja5044787
Zhang, S., Kang, P., and Meyer, T. J. (2014). Nanostructured tin catalysts for selective electrochemical reduction of carbon dioxide to formate. J. Am. Chem. Soc. 136, 1734–1737. doi: 10.1021/ja4113885
Keywords: CO2 reduction, polyoxometalate, homogeneous catalysis, water oxidation, photochemistry
Citation: Das S, Balaraju T, Barman S, Sreejith SS, Pochamoni R and Roy S (2018) A Molecular CO2 Reduction Catalyst Based on Giant Polyoxometalate {Mo368}. Front. Chem. 6:514. doi: 10.3389/fchem.2018.00514
Received: 15 January 2018; Accepted: 05 October 2018;
Published: 02 November 2018.
Edited by:
Rajesh Ramanathan, RMIT University, AustraliaReviewed by:
Tatsuki Morimoto, Tokyo University of Technology, JapanEtienne Derat, Université Pierre et Marie Curie, France
Copyright © 2018 Das, Balaraju, Barman, Sreejith, Pochamoni and Roy. This is an open-access article distributed under the terms of the Creative Commons Attribution License (CC BY). The use, distribution or reproduction in other forums is permitted, provided the original author(s) and the copyright owner(s) are credited and that the original publication in this journal is cited, in accordance with accepted academic practice. No use, distribution or reproduction is permitted which does not comply with these terms.
*Correspondence: Soumyajit Roy, cy5yb3lAbWFpbC5jY251LmVkdS5jbg==; cy5yb3lAaWlzZXJrb2wuYWMuaW4=; cm95LnNvdW15YWppdEBnbWFpbC5jb20=