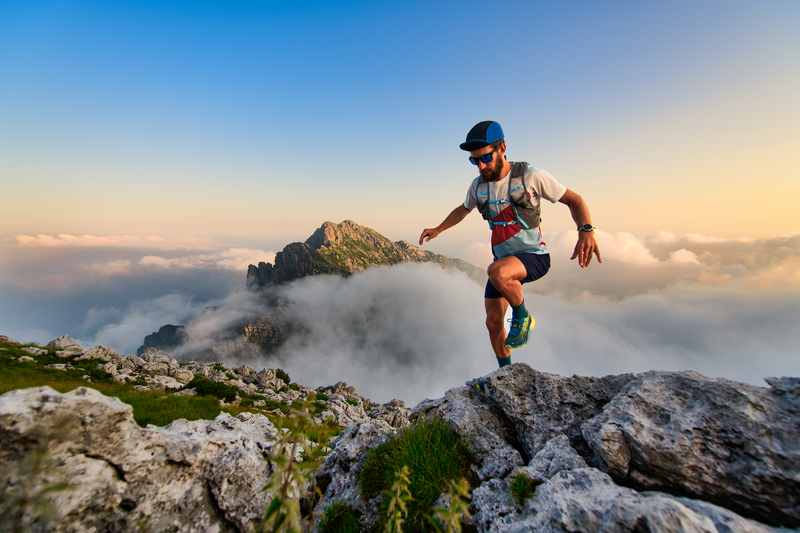
95% of researchers rate our articles as excellent or good
Learn more about the work of our research integrity team to safeguard the quality of each article we publish.
Find out more
MINI REVIEW article
Front. Chem. , 29 August 2018
Sec. Nanoscience
Volume 6 - 2018 | https://doi.org/10.3389/fchem.2018.00364
This article is part of the Research Topic Advanced Nanomaterials for Sensing Applications View all 14 articles
Tin oxide SnO2-based gas sensors have been widely used for detecting typical fault characteristic gases extracted from power transformer oil, namely, H2, CO, CO2, CH4, C2H2, C2H4, and C2H6, due to the remarkable advantages of high sensitivity, fast response, long-term stability, and so on. Herein, we present an overview of the recent significant improvement in fabrication and application of high performance SnO2-based sensors for detecting these fault characteristic gases. Promising materials for the sensitive and selective detection of each kind of fault characteristic gas have been identified. Meanwhile, the corresponding sensing mechanisms of SnO2-based gas sensors of these fault characteristic gases are comprehensively discussed. In the final section of this review, the major challenges and promising developments in this domain are also given.
Power transformers are one of the most important apparatuses in power systems, and their reliability is extremely vital to ensure the stable system operation (Zheng et al., 2018). Thermal and electrical faults in oil-filled power transformers may produce typical fault characteristic gases including hydrogen H2 (David et al., 2018), carbon monoxide CO (Joseph, 1980; Uddin et al., 2016; Zhou et al., 2018b), carbon dioxide CO2 (2015; Dan et al., 2016; Iwata et al., 2017; Zhang et al., 2017), methane CH4 (Sedghi et al., 2010), acetylene C2H2 (Qi et al., 2008), ethylene C2H4, and ethane C2H6. These typical fault characteristic gases could be dissolved in transformer oil or accumulate as free gases if produced rapidly in large quantities. Therefore, detection and analysis of the species, quantities and generation rates of these fault gases presented in the fluid allow for the identification of power transformer fault types such as corona, sparking, overheating, and arcing.
During the past few decades, dissolved gas analysis has been developed to detect the latent faults of oil-immersed power transformers (Morais and Rolim, 2006; Zhan et al., 2015; Uddin et al., 2016; Gong et al., 2018). Gas sensing detection technology is the core of dissolved gas analysis. Different types of sensing technologies have been reported in previous studies for detecting typical fault characteristic gases extracted from transformer oil, such as metal oxide semiconductor gas sensors (Zhu and Zeng, 2017a; Chen et al., 2018), catalytic combustion sensors (Liu et al., 2011), fuel cell sensors (Modjtahedi et al., 2016; Tonezzer et al., 2017), and optical sensors (Trieu-Vuong et al., 2016; Paliwal et al., 2017). Given the remarkable advantages of simple fabrication process, low maintenance cost, fast response and recovery, long service life, and so on, metal oxide semiconductor materials like SnO2 (Choi et al., 2014; Zeng et al., 2014), ZnO (Zhou et al., 2017; Zhu and Zeng, 2017b; Zhu et al., 2017), TiO2 (Zeng and Liu, 2010; Zhang et al., 2018b) and In2O3 (Cao et al., 2015) have received scientific and technological importance for many years and are widely used to detect these gases. Among them, as the most widely used gas-sensitive material, SnO2 is currently the main sensing materials used in experimental research and commercial application for detecting typical fault characteristic gases extracted from power transformer oil (Zhang et al., 2010; Zhou et al., 2018a).
Herein, the first section of this review will briefly outline the preparation of the currently used SnO2 sensing materials, the microstructure morphology, and doping modification of SnO2 sensing materials for detecting typical fault characteristic gases extracted from power transformer oil. The second section addresses the main gas sensing mechanisms of SnO2 gas sensors for these gases. The third section presents the conclusions, focusing on future challenges and potentialities associated with SnO2-based gas sensors for detecting these typical fault characteristic gases.
For detecting and analyzing typical fault characteristic gases extracted from power transformer oil with SnO2-based gas sensors, the most important concerns are the sensor sensitivity, selectivity, and repeatability (Fan et al., 2017). Combined with the research concerns mentioned above, this section briefly summarizes recent progress in the application of SnO2 sensing materials to detect typical fault characteristic gases dissolved in transformer oil. The gas sensing performance of some modified SnO2 gas sensors are listed in Table 1.
Table 1. Comparison of the representative SnO2 based sensors for fault characteristic gases extracted from power transformer oil.
Toper, temperature at which the best sensor performance, usually in terms of the highest response toward target gas, could be obtained.
S, gas sensing response of a SnO2-based sensor to target gas, which is defined as S = Ra/Rg for reducing gas and S = Rg/Ra for oxidizing gas (where Ra and Rg are the resistance of the sensor in air and in the test gas, respectively).
It is known that the metal oxide semiconductor SnO2 is a promising material for sensing the typical fault characteristic gases extracted from power transformer oil. Nevertheless, the sensing performance of the proposed sensors considerably depends on the preparation method and surface structure of the sensing materials and the dopants. The structure of modern SnO2 gas sensors could be classified into two main types including thick/thin films and nanoparticles. The preparation methods of the sensing materials mainly include the hydrothermal method, the sol-gel method, the electrospinning technique, chemical vapor deposition and so on. Different preparation methods could affect the morphology of the sensing materials and further change their gas sensing properties (Long et al., 2018; Zhang et al., 2018a; Zhou et al., 2018c). Noble metals or metal oxide doped on SnO2-based sensors can play an important role in accelerating the sensing process and improving the gas sensor performances.
In power transformers, H2 is generated from the thermal decomposition of oil at high temperatures, which is a serious problem for transformer oil (Uddin et al., 2016). Various high-efficacy and workable SnO2-based sensors have been recently introduced for detecting H2. It can be seen from the data in Table 1 that various metal particles such as Co (Liu et al., 2010), Au (Yin and Tao, 2017), and Pd (Nguyen et al., 2017) have been added to SnO2-based sensors to enhance H2 gas sensing performance. Among them, the Au-loaded SnO2 sensor can detect H2 down to 1 ppm, which is a good property for detecting low concentrations of H2 dissolved in transformer oil (Yin and Tao, 2017). K. Inyawilert reported that a SnO2 sensing film with an optimal Rh-doping level of 0.2 wt% exhibited an ultra-high response of 22,170 and a short response time of 6 s toward 30,000 ppm H2 at an optimum operating temperature of 300°C. In addition, the proposed Rh-doped SnO2 sensor displayed good H2 selectivity against NO2, SO2, C2H4, C3H6O, CH4, H2S, and CO (Inyawilert et al., 2017). Except for nanoparticles embedded in the metal oxide matrix, the morphology of the SnO2 materials could be applied to improve the H2 sensing properties, and low dimensional nanostructures have attracted increasing attention. Nguyen Kien et al. introduced the on-chip growth of SnO2 nanowire-based gas sensors to detect low concentrations of H2 gas, which responded with 2.6 for 20 ppm H2 gas at 400°C (Nguyen et al., 2017). Taken together, these findings suggest that the Au-loaded SnO2 gas sensor is the most promising candidate for detecting low concentration H2 extracted from power transformer oil.
The insulating paper, pressboard and wood blocks in power transformers contain a large number of anhydroglucose rings as well as weak C-O molecular bonds and glycosidic bonds that are thermally less stable than the hydrocarbon bonds in oil. These bonds could decompose to CO and CO2 at lower temperatures when potential faults occur. As shown in Table 1, Pt-doped SnO2 thick film (Chen et al., 2018), ZnO-SnO2 nanoparticles (Chen et al., 2015), and Au-doped SnO2 yolk-shell nanospheres (Wang et al., 2013) could be potential candidates for CO sensors. Among them, Wang et al. compared the gas sensing properties of Au@SnO2 yolk-shell nanospheres with that of hollow SnO2 nanospheres. The sensor fabricated with the Au@SnO2 yolk-shell nanospheres showed lower operating temperature (210°C), lower detection limit (5 ppm), faster response (0.3 s), and better selectivity to CO gas (Wang et al., 2013), which could be attributed to the catalytic effect of Au and enhanced electron depletion at the surface of the Au@SnO2 yolk–shell nanospheres. Moreover, SnO2 loaded with Pd nanoparticles may be another kind of promising material for CO gas sensing (Zhou et al., 2018d). Yuasa et al. prepared PdO-loaded SnO2 nanoparticles by the reverse micelle method and reported that the 0.1 mol% PdO-loaded SnO2 sensor exhibited a high gas response value of 320 to 200 ppm CO gas (Yuasa et al., 2009). Chen et al. synthesized Pd-doped SnO2 nanoparticles using a co-precipitation method, and the 1.5 wt% PdO decorated SnO2 presented the largest gas-sensitive response of 6.59 at 260°C in 400 ppm CO atmosphere (Chen et al., 2018). Another study reported that Pd-modified nanocrystalline SnO2 displayed a fairly high and reversible CO response (2–100 ppm) at room temperature (Marikutsa et al., 2010). Yin et al. prepared Pd-loaded and Fe-doped SnO2 by the sol-gel method. The composite with 10 mol% Fe and 0.2 mol% Pd had the highest sensitivity and selectivity to CO in the range of 200–3,000 ppm at 350°C, and the response value to 2,000 ppm CO was increased 13 times compared with pure SnO2 (Yin and Guo, 2014). Therefore, Pd is a highly effective catalyst for improving the sensing performance of SnO2-based sensors to CO gas (Xiong et al., 2017).
Due to their high chemical stability, conventional binary metal oxides have very low sensitivity to chemically inert gases such as CO2 (Korotcenkov and Cho, 2017). However, it was reported that La-doped SnO2 nanocomposites can be used for CO2 sensing (Kim et al., 2000). And the 8% LaOCl-SnO2 nanofibers exhibited an optimal response of 3.7 toward 1,000 ppm CO2 at 300°C with response/recovery times of 24/92 s (Xiong et al., 2017). Karthik et al. reported the sensing properties of tin oxide (SnO2) and zinc oxide (ZnO) thin films deposited onto macroporous silicon (PS) substrates to CO2 and found that the obtained SnO2/PS films showed the highest sensing response of 19 to 15 ppm CO2 gas (Karthik et al., 2018).
For hydrocarbons, namely, CH4, C2H2, C2H4, and C2H6, when high energy discharge occurs, such as arcing, in transformer oil, the content of C2H2 is relatively high. At low temperature thermal faults (T < 300°C), the contents of CH4 and C2H6 tend to be high. C2H4 is the main content of hydrocarbon while at high temperature thermal faults (T > 300°C) (Fan et al., 2017). For detecting these hydrocarbons, an online monitoring system based on a SnO2-based gas chromatographic detector for assessing the running condition of a power transformer was developed (Fan et al., 2017). Qi et al. fabricated a C2H2 sensor based on 6 wt% Sm2O3-doped SnO2, whose gas response to 1,000 ppm C2H2 could reach 63.8, 16.8 times larger than that of pure SnO2 (Qi et al., 2008). Moreover, Jin et al. reported that reduced graphene oxide (rGO)-loaded SnO2 hybrid nanocomposite showed high sensor response (12.4 toward 50 ppm), fast response-recovery time (54 and 23 s), low detection limit (1.3 ppm), good linearity, excellent selectivity and long-term stability to C2H2 (Jin et al., 2017). These results indicated that rGO would be an effective addition to enhance the sensing properties of SnO2-based sensors to C2H2 and make a contribution to developing a ppm-level gas sensor for on-line monitoring of C2H2 gas extracted from transformer oil.
Sensors for CH4 detection have also been widely studied and are partly summarized in Table 1. The 20 mol% Pt-SnO2 nanofibers exhibited excellent CH4 sensing properties over a temperature range of 100–350°C, and an obvious response of 1.11 to 1 ppm CH4 was measured at 350°C (Lu et al., 2018). Koeck et al. found a device prepared by ultra-long single crystalline SnO2-nanowires, which was able to detect a few ppm of CO and CH4 at the operating temperature of 200–250°C (Koeck et al., 2009). Chen et al. successfully synthesized Co-doped SnO2 nanofibers via an electrospinning method and reported that the Co-doped SnO2 nanofiber sensor exhibited a high response of 30.28 toward 50 ppm CH4 at 300°C (Chen et al., 2013b). For C2H4 and C2H6 detection, only a few sensors including SnO2 thin films and Pd-doped SnO2 nanoparticles are found to be effective. Jadsadapattarakul et al. reported that the sensing response, response and recovery time of SnO2 thin film sensors for selective detecting of C2H4 gas could be improved by coating a layer of [010] highly preferred-orientation silicalite-1 polycrystals (Jadsadapattarakul et al., 2010). Ahn et al. obtained SnO2 thin films by R.F. magnetron sputtering to fabricate high performance C2H4 gas sensors (Ahn et al., 2010). Chen et al. discovered that the 5 wt% Pd-doped SnO2 sensor could detect C2H6 at 400°C, and the sensor exhibited the largest gas-sensitive response of 5.89 toward 100 ppm C2H6 (Chen et al., 2013a).
It is well agreed that the sensing mechanism of SnO2 gas sensors is the change in conductivity of the metal oxide layer caused by the interaction with the surrounding atmosphere (Ducéré et al., 2012; Zeng et al., 2013; Korotcenkov and Cho, 2017). When exposed to air, oxygen molecules would be adsorbed on the surface of the SnO2 nanostructures and capture electrons from the conduction band of SnO2 to generate chemisorbed oxygen species (O−, O2−, and , depending on temperatures) (Shahabuddin et al., 2017). At low temperatures (below 150°C), oxygen molecules exist in the form of molecular ions , which would change to atomic ions O− (150–400°C) and O2− (more than 400°C) as temperatures rising (Punginsang et al., 2017). The chemical adsorption process can be explained by the following reactions:
As the electrons transfer from the conduction band of SnO2 to the chemisorbed oxygen, the electron concentration and electrical conductivity of the SnO2 film decrease. When the SnO2 film is exposed to typical fault characteristic gases, the reducing gas would react with the chemisorbed oxygen species, thereby releasing electrons back to the conduction band with increasing electrical conductivity. The sensing mechanisms of the SnO2 sensor sensing these fault gases can be explained from the following reaction paths, where O− is taken as an example (Samerjai et al., 2012).
Dopants added to SnO2-based gas sensors can accelerate the reaction process mentioned above and improve the sensing performance of gas sensors. The doped catalysts can enhance the sensing performance of a sensor in two ways, namely, chemical sensitization and electronic sensitization (Lin et al., 2017). The high surface area structures such as porous structures and hollow structures could accelerate gas diffusion on the material surface and then shorten the response time.
On the other hand, to understand the sensing mechanism of SnO2-based materials at the atomic and quantum levels, based on the framework of Density Function Theory (DFT), Li et al. established a SnO2 surface model, gas molecular models and adsorption models. A first principles calculation using the Cambridge Sequential Total Energy Package (CASTEP) program was performed, and the total energies, electronic structures and adsorption properties were investigated in detail. Theoretical calculations provided a qualitative explanation of the sensing properties of the fabricated SnO2-based sensor to various fault characteristic gases (CH4, C2H6, C2H4, and C2H2) extracted from power transformers (Li et al., 2017). Zeng et al. performed a first principles calculation to investigate how H2 gas interacts with the SnO2 (110) surface and the effect of metallic ions on the gas response of SnO2. Based on the theoretical calculation, it was reported that the Pd-doped SnO2 (110) surface could adsorb more H2 gas and receive larger electrons from adsorbed H2 molecules (Zeng et al., 2011). Other research involving a possible CO sensing mechanism for Pd-doped SnO2 sensors was investigated with first principles calculations (Chen et al., 2018), and the theoretical results demonstrated that CO molecules can grab O from the pre-adsorbed oxygen on the Pd4 cluster or the PdO cluster on the SnO2 (110) surface. These processes may play an important role in CO sensing for Pd-doped SnO2 (Chen et al., 2018). In particular, theoretical calculations indicated that CO2 molecules cannot be adsorbed onto the stoichiometric SnO2 (110) surface or SnO2 (110) surface pre-adsorbed by O and O− in dry air. However in wet air, CO2 could react with O of pre-adsorbed OH−, bringing about the formation of carbonates containing (CO3)2− and the dissociation/movement of surface OH− groups, accompanying the release of electrons from CO2 to the SnO2 surface (Wang et al., 2016).
In this mini-review, SnO2-based gas sensors for detecting typical fault characteristic gases extracted from power transformer oil have been briefly summarized. The analysis shows that the detection performances of SnO2-based gas sensors can be obviously enhanced with dopant addition or increasing the surface area of the sensing materials. Despite achieving good results, there is still much room for further development. First of all, due to the cross-sensitivity between these fault characteristic gases, selectivity is a large challenge for developing high performance SnO2-based sensors to detect these gases independently. In the future, to promote engineering applications, a combination of SnO2-based gas sensors and gas chromatography should be further studied for multi-component detection of these gases. For further development of the gas-sensing performances, the high surface area structures such as hollow and hierarchical nanostructures could be prepared to obtain more active sites for gas diffusion, and different types of dopant elements should be examined. Furthermore, the gas sensing mechanisms are still imperfect and controversial, which cannot effectively guide development of novel SnO2-based sensors and limit the prevailing application of these sensors to fault characteristic gases extracted from power transformer oil. Further research should focus on determining a satisfying mechanism model of SnO2-based sensors to provide a guide for future work.
All authors listed have made substantial, direct and intellectual contributions to the work and approved it for publication.
The authors declare that the research was conducted in the absence of any commercial or financial relationships that could be construed as a potential conflict of interest.
This work has been supported in part by the National Natural Science Foundation of China (Nos. 51507144, 5127785), the China Postdoctoral Science Foundation Project (Nos. 2015M580771, 2016T90832), and the Chongqing Science and Technology Commission (CSTC) (No. cstc2016jcyjA0400).
Ahn, H., Noh, J. H., Kim, S.-B., Overfelt, R. A., Yoon, Y. S., and Kim, D.-J. (2010). Effect of annealing and argon-to-oxygen ratio on sputtered SnO2 thin film sensor for ethylene gas detection. Mater. Chem. Phys. 124, 563–568. doi: 10.1016/j.matchemphys.2010.07.012
Cao, Y., Zhao, J., Zou, X., Jin, P.-P., Chen, H., Gao, R., et al. (2015). Synthesis of porous In2O3 microspheres as a sensitive material for early warning of hydrocarbon explosions. RSC Adv. 5, 5424–5431. doi: 10.1039/C4RA13763A
Chen, W., Li, Q., Xu, L., and Zeng, W. (2015). Gas sensing properties of ZnO-SnO2 nanostructures. J. Nanosci. Nanotechnol. 15, 1245–1252. doi: 10.1166/jnn.2015.9061
Chen, W., Zhou, Q., Gao, T., Su, X., and Wan, F. (2013a). Pd-doped SnO2-based sensor detecting characteristic fault hydrocarbon gases in transformer oil. J. Nanomater. 2013:127345. doi: 10.1155/2013/127345
Chen, W., Zhou, Q., Xu, L., Wan, F., Peng, S., and Zeng, W. (2013b). Improved methane sensing properties of Co-doped SnO2 electrospun nanofibers. J. Nanomater. 2013:173232. doi: 10.1155/2013/173232
Chen, Y., Qin, H., and Hu, J. (2018). CO sensing properties and mechanism of Pd doped SnO2 thick-films. Appl. Surf. Sci. 428, 207–217. doi: 10.1016/j.apsusc.2017.08.205
Choi, S. W., Katoch, A., Kim, J. H., and Kim, S. S. (2014). Prominent reducing gas-sensing performances of n-SnO2 nanowires by local creation of p-n heterojunctions by functionalization with p-Cr2O3 nanoparticles. ACS Appl. Mater. Inter. 6, 17723–17729. doi: 10.1021/am504164j
Dan, D. W., Yan, P. C., Zhong, X. L., Ling, L., Chang, M. S., Hong, W. Q., et al. (2016). CO2-sensing properties and mechanism of nano-SnO2 thick-film sensor. Sens. Actuat. B 227, 73–84. doi: 10.1016/j.snb.2015.12.025.
David, E. M., Gugu, H. M., Peter, R. M., Baban, P. D., Franscious, R. C., Hendrik, C. S., et al. (2018). Ultra-high sensitive and selective H2 gas sensor manifested by interface of n-n heterostructure of CeO2-SnO2 nanoparticles. Sens. Actuat. B 254, 984–995. doi: 10.1016/j.snb.2017.07.093
Ducéré, J.-M., Hemeryck, A., Esteve, A., Rouhani, M. D., Landa, G., Menini, P., et al. (2012). A computational chemist approach to gas sensors: modeling the response of SnO2 to CO, O2, and H2O Gases. J. Comput. Chem. 33, 247–258. doi: 10.1002/jcc.21959
Fan, J., Wang, F., Sun, Q., Bin, F., Ye, H., and Liu, Y. (2017). An online monitoring system for oil immersed power transformer based on SnO2 GC detector with a new quantification approach. IEEE Sens. J. 17, 6662–6671. doi: 10.1109/JSEN.2017.2734072
Gong, Z., Chen, K., Yang, Y., Zhou, X., and Yu, Q. (2018). Photoacoustic spectroscopy based multi-gas detection using high-sensitivity fiber-optic low-frequency acoustic sensor. Sens. Actuat. B 260, 357–363. doi: 10.1016/j.snb.2018.01.005
Inyawilert, K., Wisitsoraat, A., Tuantranont, A., Phanichphant, S., and Liewhiran, C. (2017). Ultra-sensitive and highly selective H2 sensors based on FSP-made Rh-substituted SnO2 sensing films. Sens. Actuat. B 240, 1141–1152. doi: 10.1016/j.snb.2016.09.094
Iwata, T., Matsuda, K., Takahashi, K., and Sawada, K. (2017). CO2 sensing characteristics of a La2O3/SnO2 stacked structure with micromachined hotplates. Sensors 17:2156. doi: 10.3390/s17092156
Jadsadapattarakul, D., Thanachayanont, C., Nukeaw, J., and Sooknoi, T. (2010). Improved selectivity, response time and recovery time by 010 highly preferred-orientation silicalite-1 layer coated on SnO2 thin film sensor for selective ethylene gas detection. Sens. Actuat. B 144, 73–80. doi: 10.1016/j.snb.2009.10.035
Jin, L., Chen, W., Zhang, H., Xiao, G., Yu, C., and Zhou, Q. (2017). Characterization of reduced graphene oxide (rGO)-loaded SnO2 nanocomposite and applications in C2H2 gas detection. Appl. Sci. 7:19. doi: 10.3390/app7010019
Joseph, J. K. (1980). Transformer fault diagnosis by dissolved-gas analysis. IEEE Trans. Ind. Appl. IA−16, 777–782. doi: 10.1109/TIA.1980.4503871
Karthik, T. V. K., Martinez, L., and Agarwal, V. (2018). Porous silicon ZnO/SnO2 structures for CO2 detection. J. Alloy Compd. 731, 853–863. doi: 10.1016/j.jallcom.2017.10.070
Kim, D. H., Yoon, J. Y., Park, H. C., and Kim, K. H. (2000). CO2-sensing characteristics of SnO2 thick film by coating lanthanum oxide. Sens. Actuat. B 62, 61–66. doi: 10.1016/S0925-4005(99)00305-6
Koeck, A., Tischner, A., Maier, T., Kast, M., Edtmaier, C., Gspan, C., et al. (2009). Atmospheric pressure fabrication of SnO2-nanowires for highly sensitive CO and CH4 detection. Sens. Actuat. B 138, 160–167. doi: 10.1016/j.snb.2009.02.055
Korotcenkov, G., and Cho, B. K. (2017). Metal oxide composites in conductometric gas sensors: achievements and challenges. Sens. Actuat. B 244, 182–210. doi: 10.1016/j.snb.2016.12.117
Li, Z., Jin, L., Liu, B., Shen, Z., Peng, S., and Zhou, Q. (2017). Experiment and simulation study of nano-SnO2 for dissolved fault gases analysis of power transformer. Sci. Adv. Mater. 9, 1888–1894. doi: 10.1166/sam.2017.3243
Lin, T., Lv, X., Li, S., and Wang, Q. (2017). The morphologies of the semiconductor oxides and their gas-sensing properties. Sensors 17:2779. doi: 10.3390/s17122779
Liu, F., Zhang, Y., Yu, Y., Xu, J., Sun, J., and Lu, G. (2011). Enhanced sensing performance of catalytic combustion methane sensor by using Pd nanorod/gamma-Al2O3. Sens. Actuat. B 160, 1091–1097. doi: 10.1016/j.snb.2011.09.032
Liu, L., Guo, C., Li, S., Wang, L., Dong, Q., and Li, W. (2010). Improved H2 sensing properties of Co-doped SnO2 nanofibers. Sens. Actuat. B 150, 806–810. doi: 10.1016/j.snb.2010.07.022
Long, H. W., Zeng, W., Wang, H., Qian, M. M., Liang, Y. H., and Wang, Z. C. (2018). Self-assembled biomolecular 1D nanostructures for aqueous sodium-ion battery. Adv. Sci. 5:1700634. doi: 10.1002/advs.201700634
Lu, W., Ding, D., Xue, Q., Du, Y., Xiong, Y., Zhang, J., et al. (2018). Great enhancement of CH4 sensitivity of SnO2 based nanofibers by heterogeneous sensitization and catalytic effect. Sens. Actuat. B 254, 393–401. doi: 10.1016/j.snb.2017.07.128
Marikutsa, A. V., Rumyantseva, M. N., Yashina, L. V., and Gaskov, A. M. (2010). Role of surface hydroxyl groups in promoting room temperature CO sensing by Pd-modified nanocrystalline SnO2. J. Solid State Chem. 183, 2389–2399. doi: 10.1016/j.jssc.2010.07.017
Min, B.-K., and Choi, S.-D. (2005). Undoped and 0.1wt.% Ca-doped Pt-catalyzed SnO2 sensors for CH4 detection. Sens. Actuat. B 108, 119–124. doi: 10.1016/j.snb.2004.12.112
Modjtahedi, A., Amirfazli, A., and Farhad, S. (2016). Low catalyst loaded ethanol gas fuel cell sensor. Sens. Actuat. B 234, 70–79. doi: 10.1016/j.snb.2016.04.108
Morais, D. R., and Rolim, J. G. (2006). A hybrid tool for detection of incipient faults in transformers based on the dissolved gas analysis of insulating oil. IEEE Trans. Power. Deliv. 21, 673–680. doi: 10.1109/TPWRD.2005.864044
Nguyen, K., Hung, C. M., Ngoc, T. M., Thanh Le, D. T., Nguyen, D. H., Nguyen Van, D., et al. (2017). Low-temperature prototype hydrogen sensors using Pd-decorated SnO2 nanowires for exhaled breath applications. Sens. Actuat. B 253, 156–163. doi: 10.1016/j.snb.2017.06.141
Paliwal, A., Sharma, A., Tomar, M., and Gupta, V. (2017). Carbon monoxide (CO) optical gas sensor based on ZnO thin films. Sens. Actuat. B 250, 679–685. doi: 10.1016/j.snb.2017.05.064
Punginsang, M., Wisitsoraat, A., Sriprachuabwong, C., Phokharatkul, D., Tuantranont, A., Phanichphant, S., et al. (2017). Roles of cobalt doping on ethanol-sensing mechanisms of flame-spray-made SnO2 nanoparticles–electrolytically exfoliated graphene interfaces. Appl. Surf. Sci. 425, 351–366. doi: 10.1016/j.apsusc.2017.06.265
Qi, Q., Tong, Z., Xue, J. Z., Hui, T. F., Li, L., Rui, W., et al. (2008). Electrical response of Sm2O3-doped SnO2 to C2H2 and effect of humidity interference. Sens. Actuat. B 134, 36–42. doi: 10.1016/j.snb.2008.04.011
Samerjai, T., Tamaekong, N., Wetchakun, K., Kruefu, V., Liewhiran, C., Siriwong, C., et al. (2012). Flame-spray-made metal-loaded semiconducting metal oxides thick films for flammable gas sensing. Sens. Actuat. B 171, 43–61. doi: 10.1016/j.snb.2012.05.052
Sedghi, S. M., Mortazavi, Y., and Khodadadi, A. (2010). Low temperature CO and CH4 dual selective gas sensor using SnO2 quantum dots prepared by sonochemical method. Sens. Actuat. B 145, 7–12. doi: 10.1016/j.snb.2009.11.002
Shahabuddin, M., Umar, A., Tomar, M., and Gupta, V. (2017). Custom designed metal anchored SnO2 sensor for H2 detection. Int. J. Hydrogen Energ. 42, 4597–4609. doi: 10.1016/j.ijhydene.2016.12.054
Tonezzer, M., Thi Thanh Le, D., Quang Huy, T., Van Hieu, N., and Iannotta, S. (2017). Selective hydrogen sensor for liquefied petroleum gas steam reforming fuel cell systems. Int. J. Hydrogen Energ. 42, 740–748. doi: 10.1016/j.ijhydene.2016.11.102
Trieu-Vuong, D., Choi, I.-Y., Son, Y.-S., and Kim, J.-C. (2016). A review on non-dispersive infrared gas sensors: improvement of sensor detection limit and interference correction. Sens. Actuat. B 231, 529–538. doi: 10.1016/j.snb.2016.03.040
Uddin, A. S. M. I., Yaqoob, U., and Chung, G.-S. (2016). Dissolved hydrogen gas analysis in transformer oil using Pd catalyst decorated on ZnO nanorod array. Sens. Actuat. B 226, 90–95. doi: 10.1016/j.snb.2015.11.110
Wang, D., Chen, Y., Liu, Z., Li, L., Shi, C., Qin, H., et al. (2016). CO2-sensing properties and mechanism of nano-SnO2 thick-film sensor. Sens. Actuat. B 227, 73–84. doi: 10.1016/j.snb.2015.12.025
Wang, L., Dou, H., Lou, Z., and Zhang, T. (2013). Encapsuled nanoreactors (Au@SnO2): a new sensing material for chemical sensors. Nanoscale 5, 2686–2691. doi: 10.1039/c2nr33088a
Xiong, Y., Xue, Q., Ling, C., Lu, W., Ding, D., Zhu, L., et al. (2017). Effective CO2 detection based on LaOCl-doped SnO2 nanofibers: insight into the role of oxygen in carrier gas. Sens. Actuat. B. 241, 725–734. doi: 10.1016/j.snb.2016.10.143
Yin, X.-T., and Guo, X.-M. (2014). Selectivity and sensitivity of Pd-loaded and Fe-doped SnO2 sensor for CO detection. Sens. Actuat. B 200, 213–218. doi: 10.1016/j.snb.2014.04.026
Yin, X.-T., and Tao, L. (2017). Fabrication and gas sensing properties of Au-loaded SnO2 composite nanoparticles for low concentration hydrogen. J. Alloy Compd. 727, 254–259. doi: 10.1016/j.jallcom.2017.08.122
Yuasa, M., Masaki, T., Kida, T., Shimanoe, K., and Yamazoe, N. (2009). Nano-sized PdO loaded SnO2 nanoparticles by reverse micelle method for highly sensitive CO gas sensor. Sens. Actuat. B 136, 99–104. doi: 10.1016/j.snb.2008.11.022
Zeng, W., He, Q., Pan, K., and Wang, Y. (2013). Synthesis of multifarious hierarchical flower-like SnO2 and their gas-sensing properties. Phys. E 54, 313–318. doi: 10.1016/j.physe.2013.07.014
Zeng, W., Li, Y., Miao, B., Lin, L., and Wang, Z. (2014). Recognition of carbon monoxide with SnO2/Ti thick-film sensor and its gas-sensing mechanism. Sens. Actuat. B 191, 1–8. doi: 10.1016/j.snb.2013.09.092
Zeng, W., Liu, T., Liu, D., and Han, E. (2011). Hydrogen sensing and mechanism of M-doped SnO2 (M = Cr3+, Cu2+ and Pd2+) nanocomposite. Sens. Actuat. B 160, 455–462. doi: 10.1016/j.snb.2011.08.008
Zeng, W., and Liu, T. M. (2010). Hydrogen sensing characteristics and mechanism of nanosize TiO2 dope with metallic ions. Phys. B 405, 564–568. doi: 10.1016/j.physb.2009.09.066
Zhan, W., Goulart, A. E., Falahi, M., and Rondla, P. (2015). Development of a low-cost self-diagnostic module for oil-immerse forced-air cooling transformers. IEEE Trans. Power.Deliv. 30, 129–137. doi: 10.1109/TPWRD.2014.2341454
Zhang, H., Li, Z., Liu, L., Xu, X., Wang, Z., and Wang, W. (2010). Enhancement of hydrogen monitoring properties based on Pd–SnO2 composite nanofibers. Sens. Actuat. B 147, 111–115. doi: 10.1016/j.snb.2010.01.056
Zhang, W., Xie, C., Zhang, G., Zhang, J., Zhang, S., and Zeng, D. (2017). Porous LaFeO3/SnO2 nanocomposite film for CO2 detection with high sensitivity. Mater. Chem. Phys. 186, 228–236. doi: 10.1016/j.matchemphys.2016.10.048
Zhang, Y. X., Zeng, W., and Li, Y. Q. (2018a). The hydrothermal synthesis of 3D hierarchical porous MoS2 microspheres assembled by nanosheets with excellent gas sensing properties. J. Alloy Compd. 749, 355–362. doi: 10.1016/j.jallcom.2018.03.307
Zhang, Y. X., Zeng, W., Ye, H., and Li, Y. Q. (2018b). Enhanced carbon monoxide sensing properties of TiO2 with exposed (001) facet: a combined first-principle and experimental study. Appl. Surf. Sci. 442, 507–516. doi: 10.1016/j.apsusc.2018.02.036
Zheng, H., Zhang, Y., Liu, J., Wei, H., Zhao, J., and Liao, R. (2018). A novel model based on wavelet LS-SVM integrated improved PSO algorithm for forecasting of dissolved gas contents in power transformers. Electr. Power Syst. Res. 155, 196–205. doi: 10.1016/j.epsr.2017.10.010
Zhou, Q., Chen, W., Xu, L., Kumar, R., Gui, Y., Zhao, Z., et al. (2018a). Highly sensitive carbon monoxide (CO) gas sensors based on Ni and Zn doped SnO2 nanomaterials. Ceram. Int. 44, 4392–4399. doi: 10.1016/j.ceramint.2017.12.038
Zhou, Q., Hong, C. X., Yao, Y., Ibrahim, A. M., Xu, L. N., Kumar, R., et al. (2017). Fabrication and characterization of highly sensitive acetone chemical sensor based on ZnO nanoballs. Materials 10:E799. doi: 10.3390/ma10070799
Zhou, Q., Lu, Z., Wei, Z., Xu, L., Gui, Y., and Chen, W. (2018b). Hydrothermal synthesis of hierarchical ultrathin NiO nanoflakes for high-performance CH4 sensing. Front. Chem. 6:194. doi: 10.3389/fchem.2018.00194
Zhou, Q., Umar, A., Sodki, E. M., Amine, A., Xu, L., Gui, Y., et al. (2018c). Fabrication and characterization of highly sensitive and selective sensors based on porous NiO nanodisks. Sens. Actuat. B 259, 604–615. doi: 10.1016/j.snb.2017.12.050
Zhou, Q., Xu, L. N., Umar, A., Chen, W. G., and Kumar, R. (2018d). Pt nanoparticles decorated SnO2 nanoneedles for efficient CO gas sensing applications. Sens. Actuat. B 256, 656–664. doi: 10.1016/j.snb.2017.09.206
Zhu, L., Li, Y., and Zeng, W. (2017). Enhanced ethanol sensing and mechanism of Cr-doped ZnO nanorods: experimental and computational study. Ceram. Int. 43, 14873–14879. doi: 10.1016/j.ceramint.2017.08.003
Zhu, L., and Zeng, W. (2017a). A novel coral rock-like ZnO and its gas sensing. Mater. Lett. 209, 244–246. doi: 10.1016/j.matlet.2017.08.020
Keywords: tin oxide, gas sensors, fault characteristic gases, power transformer oil, sensing properties, sensing mechanism
Citation: Zhang Q, Zhou Q, Lu Z, Wei Z, Xu L and Gui Y (2018) Recent Advances of SnO2-Based Sensors for Detecting Fault Characteristic Gases Extracted From Power Transformer Oil. Front. Chem. 6:364. doi: 10.3389/fchem.2018.00364
Received: 22 June 2018; Accepted: 30 July 2018;
Published: 29 August 2018.
Edited by:
Zhongchang Wang, Laboratório Ibérico Internacional de Nanotecnologia (INL), PortugalCopyright © 2018 Zhang, Zhou, Lu, Wei, Xu and Gui. This is an open-access article distributed under the terms of the Creative Commons Attribution License (CC BY). The use, distribution or reproduction in other forums is permitted, provided the original author(s) and the copyright owner(s) are credited and that the original publication in this journal is cited, in accordance with accepted academic practice. No use, distribution or reproduction is permitted which does not comply with these terms.
*Correspondence: Qu Zhou, emhvdXF1QHN3dS5lZHUuY24=
Disclaimer: All claims expressed in this article are solely those of the authors and do not necessarily represent those of their affiliated organizations, or those of the publisher, the editors and the reviewers. Any product that may be evaluated in this article or claim that may be made by its manufacturer is not guaranteed or endorsed by the publisher.
Research integrity at Frontiers
Learn more about the work of our research integrity team to safeguard the quality of each article we publish.