- 1College of Materials Science and Engineering, Hunan University, Changsha, China
- 2Hunan Province Key Laboratory for Advanced Carbon Materials and Application Technology, Hunan University, Changsha, China
Previous studies have shown that the impurity Li3V2(PO4)3 in LiVPO4F will adversely affect its electrochemical performance. In this work, we show that the crystalline composition of LiVPO4F/C is mainly influenced by the environmental temperature. The content of Li3V2(PO4)3 formed in LiVPO4F/C is 0, 11.84 and 18.75% at environmental temperatures of 10, 20, and 30°C, respectively. For the sample LVPF-30C, the SEM pattern shows a kind of alveolate microstructure and the result of selected area electron diffraction shows two sets of patterns. The LiVPO4F/C cathode without impurity phase Li3V2(PO4)3 was prepared at 10°C. The selected area electron diffraction result proves that the lattice pattern of LiVPO4F is a regular parallelogram. Electrochemical tests show that only one flat plateau around 4.2 V appears in the charge/discharge curve, and the reversible capacity is 140.4 mAh·g−1 at 0.1 C, and 116.3 mAh·g−1 at 5 C. From these analyses, it is reasonable to speculate that synthesizing LiVPO4F/C at a low environmental temperature is a practical strategy to obtain pure crystalline phase and good electrochemical performance.
Introduction
The rechargeable lithium-ion battery has been widely studied because of its applications in electric vehicles, mobile phones, and energy storage devices (Huang et al., 2009; Konarov et al., 2017). LiFePO4 delivers superior thermal stability and excellent cyclic performance, but a low working potential decreases its energy density (Yamada et al., 2003; Kim et al., 2015; Eftekhari, 2017; Wu et al., 2017).
A novel cathode lithium vanadium fluorophosphate (LiVPO4F) material has been reported (Gover et al., 2006). The working potential (4.2 V) of LiVPO4F is much higher than that of LiFePO4 and LiCoO2 (Ma et al., 2013a; Hu et al., 2014; Wu et al., 2016). Moreover, the thermal stability of LiVPO4F is better than that of LiFePO4 and LiCoO2 (Wang et al., 2014; Xu et al., 2015). If the shortcoming of electronic conductivity is solved, LiVPO4F will be an outstanding cathode material (Reddy et al., 2010; Ma et al., 2013b; Satish et al., 2016). Some improvements have been adjusted to LiVPO4F cathode, such as cation doped, carbon coated and various synthesized routes (Wang et al., 2013a; Liu et al., 2016; Wu et al., 2018). Recently, adopting facile and controllable methods to prepare LiVPO4F is the key areas of research. LiVPO4F was reported by two-step carbothermal reduction in some references. However, this method suffers from high energy consumption and a large content of carbon, because the intermediate VPO4 is prepared separately at 700–800°C (Ma et al., 2014; Liu et al., 2015; Wang et al., 2016).
Thus, a novel one-step method in which the synthesis of VPO4 is omitted and carbon content is restricted to a very low level is of great research interest. Although the electrochemical performance of LiVPO4F prepared is improved, the plateaus belonging to impurity Li3V2(PO4)3 come into being (Liu et al., 2012; Wang et al., 2013b; Xiao et al., 2013). Therefore, the formation of Li3V2(PO4)3 is observed even though we use a synthesis method that employs a novel chemical reduction route. The content of Li3V2(PO4)3 should be carefully controlled because it may adversely affect the performance of the LiVPO4F cathode.
In this work, we discovered that the formation of impurity Li3V2(PO4)3 is directly related to the environmental temperature. The formation mechanism was investigated through further analysis of the structure and synthesis procedures.
Experimental
Materials Synthesis
LiVPO4F/C was synthesized by using a novel chemical reduction method. The chemical reagent used was of analytical reagent grade. 0.03 mol H2C2O4 dissolved in deionized water was used as a chelating agent and reducing agent. 0.01 mol V2O5 was added slowly under vigorous magnetic stirring at 60°C. LiF and NH4H2PO4 at the molar ratio of 1:1 to vanadium were introduced in after 10 min. A PVDF carbon source of 1.4943 g was dispersed in 30 ml water in a solution of hexadecyl trimethyl ammonium bromide under ultrasonic assistance at 50°C. Subsequently, the PVDF suspension was added to the reaction system. Finally, the suspension was dried overnight in vacuum at 85°C. The precursor was presintered at 400°C for 5 h and sintered at 800°C for 4 h in a tubular furnace with flowing high-purity argon.
Characterization
The crystal structure of the material was examined by X-ray diffraction (XRD, Rigaku D/MAX 2500). The morphology and elemental content were investigated with scanning electron microscopy (SEM, Navo NanoSEM230) and energy disperse spectroscopy (EDS). Nanoscale morphology and selected area electron diffraction (SAED) were performed by using high-resolution transmission electron microscopy (HRTEM, JEOL-3010).
Electrochemical Test
The electrochemical performance of LiVPO4F/C electrodes was evaluated using an Arbin BT2000 battery test system. The cathode film was fabricated by mixing LiVPO4F/C (80 wt.%), acetylene black (15 wt.%), and PVDF (5 wt.%) in the solvent N-methyl pyrrolidone, and the slurry was coated on an aluminum collector. The electrodes were dried in a vacuum oven at 120°C for 12 h and 2016 coin-type cells were assembled in a glove box (S1220/750). The electrolyte was 1.3 mol·L−1 LiPF6 in a mixing solvent of ethylene carbonate, dimethyl carbonate, and ethyl methyl carbonate (1:1:1). A lithium foil and a polypropylene separator (Celgard 2400) were used as counter electrode and separator, respectively.
Results and Discussion
The electrochemical performance of triclinic LiVPO4F/C is partially determined by the content of impurity Li3V2(PO4)3/C in it. Our study revealed that LiVPO4F prepared at a high environmental temperature delivers poor performance. To investigate the reason for this, we synthesized LiVPO4F/C at different environmental temperatures (30, 20, and 10°C), and named the respective samples as LVPF-30C, LVPF-20C, and LVPF-10C.
The XRD patterns of the samples are shown in Figure 1A. The main diffraction peaks correspond to a triclinic system with the space group of P-1, and can be indexed as the standard pattern of LiVPO4F (Barker et al., 2003; Huang et al., 2009). The absence of peaks corresponding to crystalline carbon proves that carbon is amorphous. No impurity peaks in LVPF-10C, which delivers the strongest peaks among the samples, was found. The refined cell parameters a, b, and c were 5.174, 5.308, and 7.509 Å, and the cell volume was 174.18 Å3. These results compare well with the classic results reported by Barker (Barker et al., 2005). However, the peaks at 20.69°, 23.53°, and 24.48° belonging to the impurity Li3V2(PO4)3 (symbol # in Figure 1A) occur in the curves of LVPF-30C and LVPF-20C (Zhu et al., 2008). The percentages of Li3V2(PO4)3 in LiVPO4F were estimated by refining the XRD patterns in Figure 1B. The content of Li3V2(PO4)3 increased gradually from 0% (10°C) to 11.84% (20°C) and 18.75% (30°C). Hence, our preliminary presumption is that low environmental temperature plays an important role in the preparation of pure LiVPO4F.
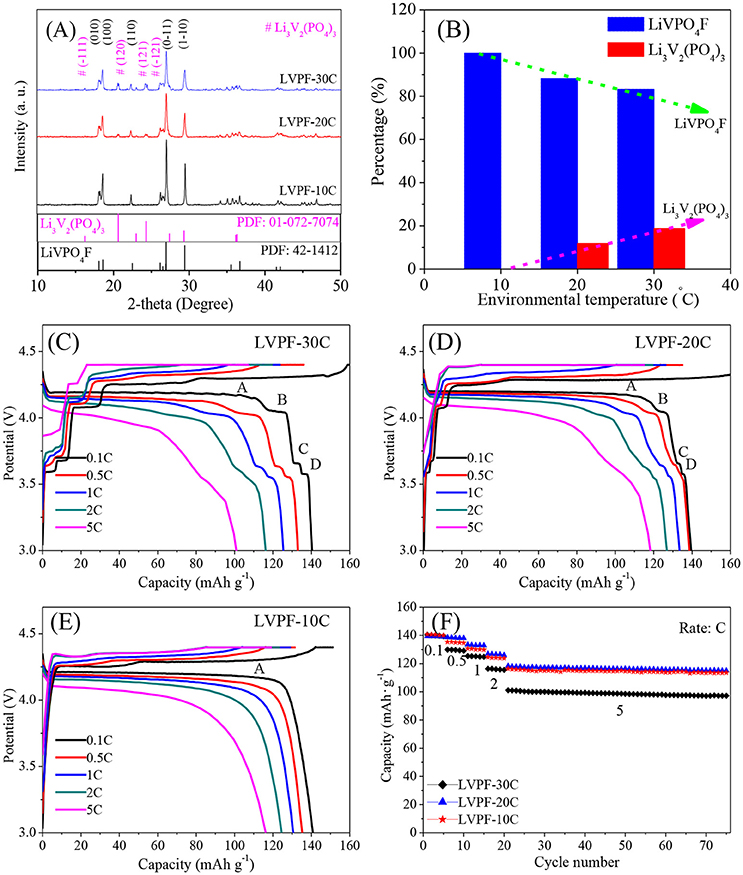
Figure 1. XRD patterns of LiVPO4F/C (A), percentage of Li3V2(PO4)3 in LiVPO4F/C (B), and charge/discharge curves of LiVPO4F/C (C–F).
In Figure 1C, four flat plateaus (A, B, C, and D) appear in the discharge curves of LVPF-30C. The predominant plateau A around 4.2 V is attributed to LiVPO4F/C, and is in accordance with Barker's work (Barker et al., 2003), and B, C, and D are assigned to Li3V2(PO4)3/C. The specific capacities at 0.1 C and 5 C are 138.6 and 101.1 mAh·g−1. However, when temperature drops to 20°C (corresponding to LVPF-20C, Figure 1D), the plateaus of Li3V2(PO4)3/C are shorter than before, establishing the decreasing content of impurity. The specific capacity increases obviously, especially at 5 C (118.3 mAh·g−1). Further, only a plateau A at 4.2 V without other plateaus of impurity Li3V2(PO4)3/C is observed in LVPF-10C (Figure 1E). It is important to note that Li3V2(PO4)3/C disappear entirely. The specific capacities at 0.1 C 1 C and 5 C are 140.4 mAh·g−1, 130.6 mAh·g−1 and 116.3 mAh·g−1, which are very close to that of LVPF-20C in Figure 1F. LVPF-30C delivers the worst performance at a high current density. LVPF-20C with 11.84% impurity Li3V2(PO4)3/C possesses the optimum capacity at a high current density. The reason is that Li3V2(PO4)3/C is a fast ion conductor and allows a fast transfer of lithium ions in the cathode. Nevertheless, an excess of the impurity Li3V2(PO4)3/C in LiVPO4F/C adversely affects the rate and the cycling capability.
In Figure 2A, the alveolate structure can be easily observed in LVPF-30C. The surface of most particles is broken. This structure is observed in the HRTEM image. The SAED pattern is made up of two sets of lattices with different characteristics (inset of Figure 2B). These parallelogram lattices are attributed to triclinic LiVPO4F (bottom) and monoclinic Li3V2(PO4)3 (top). In Figure 2C, the EDS image in the alveolate field proves the existence of Li3V2(PO4)3 distinctly because the content of fluorine is much lower than that of vanadium. Figures 2D,E show that vanadium is uniformly distributed on the surface of particles and a small quantity of fluorine is detected. This proves that impurity Li3V2(PO4)3 without fluorine is formed in the alveolate zone.
There is no alveolate structure on the flawless surface of LVPF-10C (Figure 2F) and the lattice fringes can be clearly observed (Figure 2G). The pattern of SAED in the square frame is a typical parallelogram, and is similar to the bottom lattice in Figure 2B. This pattern is attributed to the typical crystalline form of LiVPO4F with the triclinic system. Thus, LVPF-10C possesses a good crystalline morphology with a thin layer covering on the surface of the crystalline LiVPO4F. Its lattice pattern is a series of concentric circles, which is the characteristic of amorphous carbon (Song et al., 2008). The atomic contents of vanadium and fluorine are 8.61 and 8.30%, respectively, and match well with the atomic ratio of LiVPO4F in Figure 2H. Thus, we conclude that low temperature (10°C) helps to prepare pure phase LiVPO4F.
The formation mechanism of the alveolate structure is investigated in Figure 3. On one hand, the excessive oxalic acid hydrolyzes in deionized water and produces hydrogen ions in aqueous solution. Ammonium dihydrogen phosphate generates ammonium ions in the hydrolysis reaction. A fluoride compound is formed when a hydrogen ion and an ammonium ion are combined with a fluoride ion released by LiF. Therefore, HF and NH4F are formed in the reaction (Zhou et al., 2009). It is well known that fluoride compounds are unstable and easily evaporate. From the viewpoint of reaction kinetics, the volatilization rate of fluoride will increase at least 6 to 8 times at 30°C compared to 10°C in reaction and drying. Therefore, the content of fluorine in the precursor at 30°C is evidently lower than that at 10°C. It can be inferred that the impurity Li3V2(PO4)3 is formed in this condition. On the other hand, the temperature of the tubular furnace drops slowly at 30°C. The cooling rate of LVPF-30C is lower than that of LVPF-10C. The longer cooling time of LVPF-30C accelerates the evaporation of fluoride, especially at 600–800°C. Therefore, this ensures that the fluorine content in LVPF-30C is much less than the value determined. The impurity Li3V2(PO4)3 is formed, which is in accordance with the above analysis of its structure and morphology.
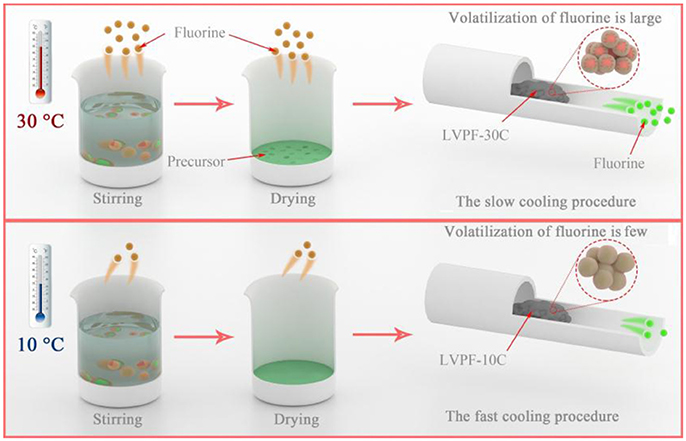
Figure 3. Schematic of the volatilization of fluorine at environmental temperatures of 30°C and 10°C.
Hence, the volatilization of fluoride should be inhibited in the preparation processes of LiVPO4F. Based on all of the evidence we have presented above, we legitimately conclude that a lower environmental temperature is more helpful to synthesize a LiVPO4F/C cathode with a low content of impurity and excellent electrochemical performance.
Conclusions
A sample of LVPF-10C, which was prepared at an environmental temperature of 10°C, exhibited a regular parallelogram space pattern that is attributed to the pure triclinic form of LiVPO4F. High environmental temperature accelerates the volatilization of fluoride in the drying and sintering process and decreases the fluorine content. Then, a large quantity of Li3V2(PO4)3 reduces the plateaus in the discharge curves and deteriorates the rate of performance in LVPF-30C. Therefore, our work is devoted to give a direction to improve the synthetic process and advise what we need to do in the future.
Author Contributions
TZ wrote the paper and designed the main part of the experiment. CF was the main advisor. ZW and QL carried out material preparation and the electrochemical test. ZZ discussed and refined the paper. TZ, CF, ZW, QL, and ZZ proposed the research. CF, SH, and JL obtained the main financial support for the research and supervised all the experiments.
Conflict of Interest Statement
The authors declare that the research was conducted in the absence of any commercial or financial relationships that could be construed as a potential conflict of interest.
Acknowledgments
This work was supported by the National Natural Science Foundation of China [51472082, 51672079, 51372079] and science and technology project of Changsha City [k1508010–11].
References
Barker, J., Gover, R. K. B., Burns, P., Bryan, A., Saidi, M. Y., and Swoyer, J. L. (2005). Structural and electrochemical properties of lithium vanadium fluorophosphate, LiVPO4F. J. Power Sources 146, 516–520. doi: 10.1016/j.jpowsour.2005.03.126
Barker, J., Saidi, M. Y., and Swoyer, J. L. (2003). Electrochemical insertion properties of the novel lithium vanadium fluorophosphate, LiVPO4F. J. Electrochem. Soc. 150, A1394–A1398. doi: 10.1016/j.ssi.2003.09.009
Eftekhari, A. (2017). LiFePO4/C nanocomposites for lithium-ion batteries. J. Power Sources 343, 395–411. doi: 10.1016/j.jpowsour.2017.01.080
Gover, R. K. B., Burns, P., Bryan, A., Saidi, M. Y., Swoyer, J. L., and Barker, J. (2006). LiVPO4F: a new active material for safe lithium-ion batteries. Solid State Ionics 177, 2635–2638. doi: 10.1016/j.ssi.2006.04.049
Hu, G. R., Cao, J. C., Peng, Z. D., Cao, Y. B., and Du, K. (2014). Enhanced high-voltage properties of LiCoO2 coated with Li[Li0.2Mn0.6Ni0.2]O2. Electrochim. Acta 149, 49–55. doi: 10.1016/j.electacta.2014.10.072
Huang, H., Faulkner, T., Barker, J., and Saidi, M. Y. (2009). Lithium metal phosphates, power and automotive applications. J. Power Sources 189, 748–751. doi: 10.1016/j.jpowsour.2008.08.024
Kim, M., Lee, S., and Kang, B. (2015). Fast-rate capable electrode material with higher energy density than LiFePO4:4.2V LiVPO4F synthesized by scalable single-Step solid-state reaction. Adv. Sci. (Weinh) 3:1500366. doi: 10.1002/advs.201500366
Konarov, A., Myung, S. T., and Sun, Y. K. (2017). Cathode materials for future electric vehicles and energy storage systems. Acs Energy Lett. 2, 703–708. doi: 10.1021/acsenergylett.7b00130
Liu, J. Q., Zhong, S. K., Ling, W. U., Kang, W., and Fan, L. Ü. (2012). Electrochemical performance of LiVPO4F/C synthesized by different methods. Chin. J. Power Sources 22, s157–s161. doi: 10.1016/S1003-6326(12)61702-6
Liu, Z., Peng, W., Fan, Y., Li, X., Wang, Z., Guo, H., et al. (2015). One-step facile synthesis of graphene-decorated LiVPO4F/C nanocomposite as cathode for high-performance lithium ion battery. Ceram. Int. 41, 9188–9192. doi: 10.1016/j.ceramint.2015.03.090
Liu, Z., Peng, W., Shih, K., Wang, J., Wang, Z., Guo, H., et al. (2016). A MoS2 coating strategy to improve the comprehensive electrochemical performance of LiVPO4F. J. Power Sources 315, 294–301. doi: 10.1016/j.jpowsour.2016.02.083
Ma, R., Shao, L., Wu, K., Shui, M., Ren, Y., and Shu, J. (2013a). Comparison of LiVPO4F to Li4Ti5O12 as anode materials for lithium-ion batteries. ACS Appl. Mater. Inter. 5, 8615–8627. doi: 10.1021/am402132u
Ma, R., Shao, L., Wu, K., Shui, M., Wang, D., and Shu, J. (2014). Effects of oxidation on structure and performance of LiVPO4F as cathode material for lithium-ion batteries. J. Power Sources 248, 874–885. doi: 10.1016/j.jpowsour.2013.10.029
Ma, R., Shu, J., Lu, H., Miao, S., Shao, L., Wang, D., et al. (2013b). Ex situ FTIR spectroscopy study of LiVPO4F as cathode material for lithium-ion batteries. Ionics (Kiel). 19, 725–730. doi: 10.1007/s11581-012-0807-8
Reddy, M. V., Rao, G. V. S., and Chowdari, B. V. R. (2010). Long-term cycling studies on 4 V-cathode, lithium vanadium fluorophosphate. J. Power Sources 195, 5768–5774. doi: 10.1016/j.jpowsour.2010.03.032
Satish, R., Aravindan, V., Ling, W. C., and Madhavi, S. (2016). LiVPO4F: a new cathode for high-energy lithium ion capacitors. Chemistryselect 1, 3316–3322. doi: 10.1002/slct.201600912
Song, M. S., Kim, D. Y., Kang, Y. M., Kim, Y. I., Lee, J. Y., and Kwon, H. S. (2008). Amphoteric effects of Fe2P on electrochemical performance of lithium iron phosphate-carbon composite synthesized by ball-milling and microwave heating. J. Power Sources 180, 546–552. doi: 10.1016/j.jpowsour.2008.01.079
Wang, J., Liu, Z., Yan, G., Li, H., Peng, W., Li, X., et al. (2016). Improving the electrochemical performance of lithium vanadium fluorophosphate cathode material: focus on interfacial stability. J. Power Sources 329, 553–557. doi: 10.1016/j.jpowsour.2016.08.131
Wang, J., Li, X., Wang, Z., Guo, H., Li, Y., He, Z., et al. (2013a). Enhancement of electrochemical performance of Al-doped LiVPO4F using AlF3 as aluminum source. J. Alloys Comp. 581, 836–842. doi: 10.1016/j.jallcom.2013.07.147
Wang, J., Li, X., Wang, Z., Huang, B., Wang, Z., and Guo, H. (2014). Nanosized LiVPO4F/graphene composite: a promising anode material for lithium ion batteries. J. Power Sources 251, 325–330. doi: 10.1016/j.jpowsour.2013.11.095
Wang, J., Wang, Z., Shen, L., Li, X., Guo, H., Tang, W., et al. (2013b). Synthesis and performance of LiVPO4F/C-based cathode material for lithium ion battery. T. Nonferr. Metal. Soc. 23, 1718–1722. doi: 10.1016/S1003-6326(13)62653-9
Wu, L., Shi, S., Zhang, X., Yang, Y., Liu, J., Tang, S., et al. (2018). Room-temperature pre-reduction of spinning solution for the synthesis of Na3V2(PO4)3/C nanofibers as high-performance cathode materials for Na-ion batteries. Electrochim. Acta 274, 233–241. doi: 10.1016/j.electacta.2018.04.122
Wu, X., Li, Y., Liu, Z., Wu, X., Xiong, L., and Chen, J. (2016). The electrochemical performance of aqueous rechargeable battery of Zn/Na0.44MnO2 based on hybrid electrolyte. J. Power Sources 336, 35–39. doi: 10.1016/j.jpowsour.2016.10.053
Wu, X., Xiang, Y., Peng, Q., Wu, X., Li, Y., and Wu, X. M. (2017). A green-low-cost rechargeable aqueous zinc-ion battery using hollow porous spinel ZnMn2O4 as the cathode material. J. Mater. Chem. A 5, 17990–17997. doi: 10.1039/c7ta00100b
Xiao, P., Lai, M., and Lu, L. (2013). Transport and electrochemical properties of high potential tavorite LiVPO4F. Solid State Ionics 242, 10–19. doi: 10.1016/j.ssi.2013.04.002
Xu, G., Liu, Z., Zhang, C., Cui, G., and Chen, L. (2015). Strategies for improving the cyclability and thermo-stability of LiMn2O4-based batteries at elevated temperatures. J. Mater. Chem. A 3, 4092–4123. doi: 10.1039/c4ta06264g
Yamada, A., Hosoya, M., Chung, S., Kudo, Y., Hinokuma, K., Liu, K., et al. (2003). Olivine-type cathodes. J. Power Sources 119–121, 232–238. doi: 10.1016/S0378-7753(03)00239-8
Zhou, F., Zhao, X., and Dahn, J. R. (2009). Reactivity of charged LiVPO4F with 1M LiPF6 EC: DEC electrolyte at high temperature as studied by accelerating rate calorimetry. Electrochem. Commun. 11, 589–591. doi: 10.1016/j.elecom.2008.12.052
Keywords: lithium-ion batteries, lithium vanadium fluorophosphates, environmental temperature, alveolate structure, electrochemical performance
Citation: Zeng T, Fan C, Wen Z, Li Q, Zhou Z, Han S and Liu J (2018) Effect of Environmental Temperature on the Content of Impurity Li3V2(PO4)3/C in LiVPO4F/C Cathode for Lithium-ion Batteries. Front. Chem. 6:283. doi: 10.3389/fchem.2018.00283
Received: 26 April 2018; Accepted: 22 June 2018;
Published: 24 July 2018.
Edited by:
Jiexi Wang, Central South University, ChinaReviewed by:
Xianwen Wu, Jishou University, ChinaJie Shu, Ningbo University, China
Guochun Yan, Collège de France, France
Haoran Jiang, Hong Kong University of Science and Technology, Hong Kong
Copyright © 2018 Zeng, Fan, Wen, Li, Zhou, Han and Liu. This is an open-access article distributed under the terms of the Creative Commons Attribution License (CC BY). The use, distribution or reproduction in other forums is permitted, provided the original author(s) and the copyright owner(s) are credited and that the original publication in this journal is cited, in accordance with accepted academic practice. No use, distribution or reproduction is permitted which does not comply with these terms.
*Correspondence: Changling Fan, ZmFuY2xAaG51LmVkdS5jbg==
Jinshui Liu, anNsaXVAaG51LmVkdS5jbg==