- Department of Materials Physics, School of Materials Science and Engineering, Central South University, Changsha, China
Single crystalline fork-like potassium vanadate (K2V8O21) has been successfully prepared by electrospinning method with a subsequent annealing process. The as-obtained K2V8O21 forks show a unique layer-by-layer stacked structure. When used as cathode materials for lithium-ion batteries, the as-prepared fork-like materials exhibit high specific discharge capacity and excellent cyclic stability. High specific discharge capacities of 200.2 and 131.5 mA h g−1 can be delivered at the current densities of 50 and 500 mA g−1, respectively. Furthermore, the K2V8O21 electrode exhibits excellent long-term cycling stability which maintains a capacity of 108.3 mA h g−1 after 300 cycles at 500 mA g−1 with a fading rate of only 0.043% per cycle. The results demonstrate their potential applications in next-generation high-performance lithium-ion batteries.
Introduction
Rechargeable lithium-ion batteries (LIBs) are one of the most important energy storage devices in microchips, cell phones, electric vehicles (EVs), and hybrid electric vehicles (HEVs) (Whittingham, 2004; Armand and Tarascon, 2008; Zheng et al., 2015; Ou et al., 2017; Pan et al., 2017a,b; Yang et al., 2017). Layer-structured LiCoO2 has been extensively studied and largely used as commercial cathode materials due to their high working potential, high energy density and good cycling performance. However, it delivers a relatively low capacity of ~130 m Ah g−1, which is only half of its theoretical capacity, thus restricting its further expansion in LIBs (Goodenough and Kim, 2010; Liang et al., 2014). In addition, the scarcity of Co resources has further pushed up the cost of LIBs manufacturing. Therefore, it would be interesting to find alternative electrode materials with lower cost, larger specific capacity and better safety.
As important cathode candidates in LIBs, vanadium oxides and vanadate have attracted extensive interests owing to their abundant reservation, high specific capacity, and high Li+ diffusion efficiency (Maingot et al., 1993; Torardi and Miao, 2002; Ng et al., 2007; Liu et al., 2009; Mai et al., 2010a,b; Wee et al., 2010; Liu and Yang, 2011; Pan et al., 2011a; Rui et al., 2011; Varadaraajan et al., 2011; Wang et al., 2011; Liang et al., 2013b; Jian et al., 2014; Zhou et al., 2014; Meng et al., 2016; An et al., 2017). For example, vanadium pentoxide (V2O5) has a high theoretical capacity of 442 mA h g−1, but its commercialization is still limited by poor cyclic stability (Owens et al., 1999; Cao et al., 2005; Wang et al., 2006). Many studies have demonstrated that the incorporation of metal cations (such as Li+, Na+, K+, Ag+, Zn2+, Cu2+, etc.) (Ma et al., 2008; Liu and Tang, 2009; Cheng and Chen, 2011; Liang et al., 2013a, 2014; Bach et al., 2014; Yang et al., 2016) into V2O5 interlayers is favorable to improve the electronic conductivity (Zhou et al., 2014), simultaneously creating more Li+ intercalation channels (Khoo et al., 2010) and improve the structural stability (Pan et al., 2011b). It was reported that introduction of K+ into vanadium oxygen layers is effective to deliver high specific capacity and excellent reversibility by the pillar effect and larger interlamellar space (Baddour-Hadjean et al., 2011, 2014; Xu et al., 2012, 2015; Fang et al., 2015, 2016; Meng et al., 2016). In addition, K2V8O21 is a more stable crystal phase compared to V2O5 or KVO3 and it has a higher theoretical capacity of 261 mA h g−1. Manev et al. reported the usage of K2V8O21 as a cathode material for the first time, which deliver an initial discharge capacity of about 190 mA h g−1, but with a poor capacity retention (Manev et al., 1993). The electrochemical performance of K2V8O21 can be improved by incorporation water into the layered structure by hydrothermal treatment (Aleksandrova et al., 2006, 2009). By doping inactive metal ions such as Nb5+ and Ti4+ into the crystal structure by mechanical ball milling, the capacity of K2V8O21 (Ni et al., 2015) can also be enhanced. However, the cycling performance of K2V8O21 is still unsatisfactory, in particular for the long-term cycling stability.
One-dimensional (1D) nanostructures such as nanowires (Mai et al., 2010b), nanorods (Gu et al., 2015), nanofibers (Wee et al., 2010) could offer shorter Li-ion diffusion pathways, higher specific surface area and faster electron transfer along longitudinal direction (Li et al., 2016). Besides, 1D nanostructures with layer-by-layer flakes have been proved favorable to increase the Li+ diffusion rate and enlarge the contact areas between electrode and electrolyte (Liang et al., 2013a). layer-by-layer structured K0.25V2O5 electrode exhibits a high initial discharge specific capacity of 256 mA h g−1 and superior long-term cycling stability (Fang et al., 2015).
In this work, we reported the synthesis of single crystal fork-like K2V8O21 (KVO) by a facile electrospinning method with a subsequent calcination process. Fork-like K2V8O21 material with layer-by-layer stacking structure has been fabricated and is used as a cathode material in LIBs. The K2V8O21 forks exhibit high specific capacity and superior long-term cycling stability.
Experimental
Materials and Synthesis
All reagents and solvents were of analytical grade and used as received without further purification. K2V8O21 was synthesized by single-nozzle electrospinning technique with subsequent annealing. Vanadium pentoxide (V2O5, ≥ 99.0 %), oxalic acid (H2C2O4·2H2O, ≥ 98.0 %), potassium nitrate (KNO3, ≥ 99.0 %) and polyvinylpyrrolidone (PVP, Mw ≈ 1, 300, 000) were used as starting materials. In a typical synthesis, 0.1 g of V2O5 and H2C2O4·2H2O in a molar ratio of 1:3 were dissolved in 5 mL of de-ionized water (DI) under vigorous stirring at 80°C for about 30 min to form a clear dark blue solution. Then, 0.0278 g of KNO3 was added into the above vanadium oxalate solution under magnetic stirring for 10 min before the addition of 5 mL of N,N-Dimethylformamide (DMF) and 1 g of PVP to form a homogeneous viscous dark blue precursor solution. Subsequently, the precursor solution was loaded into a 10 mL plastic syringe with a 21-gauge stainless steel nozzle. The solution was subjected to electrospinning at a DC voltage of 12 kV under a flow rate of 0.04 mm min−1. The electrospun nanofibers were collected on aluminum foil with a distance of 15 cm between the nozzle and aluminum collector. Finally, the obtained precursor nanofibers were annealed in a muffle furnace at 500°C in air for 2 h to yield the fork-like K2V8O21. The temperature ramping rate was set of 1°C min−1.
Material Characterization
The crystal phase of the as-prepared K2V8O21 were characterized by X-ray diffraction (XRD, Rigaku D/Max 2500) with non-monochromated Cu Kα radiation (λ = 1.54178 Å). The microscopic morphology of the products was investigated by scanning electron microscopy (SEM, FEI Nova Nano SEM 230) and transmission electron microscopy (TEM, JEOL JEM-2100 F). Thermogravimetric analysis (TGA) was carried out on a NETZSCH STA 449C analyzer in air from room temperature to 700°C with a heating rate of 10°C min−1.
Electrochemical Measurements
Electrochemical measurement was performed using standard CR2016 type coin cells. The fork-like K2V8O21, acetylene black and polyvinylidene fluoride (PVDF) binder in a weight ratio of 70:20:10 were added into N-methyl-2-pyrrolidone (NMP) solution to make the slurry, which was coated on alumina foil and dried at 100°C overnight under vacuum to obtain the electrodes. The mass loading of the K2V8O21 cathode material for coin cell testing was about 1 mg cm−2. All coin cells were assembled in a glove box (Mbraun, Germany) filled with ultra-high pure argon gas. Metallic lithium foils and polypropylene membrane were used as counter electrode and separator, respectively. And 1 M LiPF6 solution in ethylene carbonate/dimethyl carbonate (EC/DMC; 1:1, v/v) was used as the electrolyte. The cyclic voltammetry (CV) measurements of Li/K2V8O21 coin cell was conducted using an electrochemical workstation (CHI660C, China) at a scan rate of 0.1 mV s−1 in the voltage of 1.5–4 V. The galvanostatic charge/discharge (GCD) performances of the K2V8O21 electrodes were conducted at room temperature on a Land Battery Tester (Land CT2001A, China) in a voltage range of 1.5–4.0 V vs. Li/Li+.
Results and Discussion
Figure 1 shows the synthesis process of fork-like K2V8O21 through the single-nozzle electrospinning technique and a subsequent annealing. Firstly, KNO3, V2O5, H2C2O4·2H2O, PVP, DMF and DI were mixed to form a homogeneous viscous precursor, which was electrospun into nanofibers by electrospinning process. The followed annealing treatment can convert the precursor nanofibers to the fork-like crystalline K2V8O21 nanostructures in air at 500°C.
Figure 2 shows the structure and morphology of the electrospun precursor and its annealed products of fork-like K2V8O21. Figure 2a shows the SEM image of the precursor nanofibers. The K2V8O21 precursor nanofibers has an average diameter of 100 nm and a smooth and uniform surface. The formation of fiber-like structure can be attributed to the polymers serving as templates during the electrospinning process. Typically, the subsequent annealing could remove the backbones of polymers, and control the morphologies of final products such as nanofibers (Wee et al., 2010), nanowires (Mai et al., 2010b), and nanotubes (Zhao et al., 2015). 350°C was chosen for sintering the precursor nanofibers, and it can be seen from Figure 2b that the fibrous structure was not retained. At this temperature, PVP was decomposed and then converted to carbonaceous species (Liang et al., 2007; Teh et al., 2013). Meanwhile, the KVO nanoparticles that encapsulated in the nanofibers were gradually grown into flat flakes. Because of PVP enclosing the precursor with a fibrous structure and restricting the growth of KVO nanoparticles, KVO small flakes were formed into a hierarchical nanobelt structure. As shown in Figure 2c, the final fork-like K2V8O21 that combined nanorods and nanoflakes formed when the heating temperature was elevated to 500°C. During the annealing process, the transformation of KVO nanoflakes into KVO forks occurred, which was caused by the re-crystallization of K2V8O21. Large space can be clearly seen among the K2V8O21 forks, which may be favorable to the diffusion of lithium ion. Figure 2d shows a single K2V8O21 fork at high magnification, which reveals the layered structures of KVO forks, indicating a feature of layer-by-layer structure. K2V8O21 compound was also prepared by sol-gel route for comparison. The width of sol-gel K2V8O21 compound (SG-KVO) is larger than that of the electrospun fork-like K2V8O21 as shown in Figure S1 (Supplementary Material). Furthermore, the electrospun fork-like K2V8O21 (ES-KVO) show two rod-like tips which are different from the sol-gel K2V8O21, which could be induced by the combustion of PVP and re-crystallization of K2V8O21 clusters. Figure 2f shows the element mapping of fork-like K2V8O21 in Figure 2e, in which the red, blue, yellow and green spot represent the element of carbon, potassium, vanadium and oxygen elements, respectively. It can be seen that these elements are present in the sample and homogeneously dispersed on the fork-like nanostructures, further confirming that C element is well distributed in the fork-like K2V8O21.
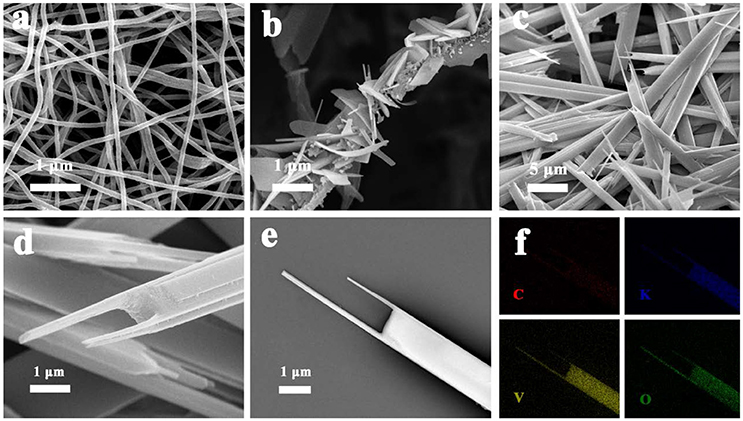
Figure 2. Different magnifications of SEM images of: (a) the precursor nanofibers and K2V8O21 nanostructures that were annealed at (b) 350°C, (c–e) 500°C; (f) Elemental mapping images of K2V8O21 compound with the element of C, K, V, O.
Thermogravimetric analysis (TGA) was performed from 25 to 700°C to investigate the thermal decomposition of KVO at a heating rate of 10°C min−1 (Figure 3). The as-obtained KVO nanofibers lost mass weight owing to the decomposition of metallic precursor and PVP during the annealing process, leading to the significant surface rupture and the formation of K2V8O21 simultaneously. The mass loss within the range of 25–200°C was attributed to the loss of volatile components, such as residual solvent (DMF, H2O) and adsorbed moisture (Ko et al., 2015). At 332.2°C, there was a prominent DSC exothermic peak with a dramatic mass-loss. This exothermic peak may be due to the decomposition of vanadium oxalate, potassium nitrate and the degradation of PVP, which has both intra—and intermolecular transfer reactions according to the degradation mechanisms (Azhari and Dish, 1998). The following exothermic peak, which was accompanied with a significant weight loss at 478.3°C was probably due to the formation of K2V8O21 compound and the oxidation of carbon and carbon monoxide that originated from PVP molecules (Wang et al., 2008). It can be concluded that at 500°C the chemical reaction was completed and the K2V8O21 was obtained. From 500 to 570°C, there was a mass loss about 4.33% and stabilized at about 15% at temperatures above 570°C, which implied that some C elements were remained when the fork-like KVO was obtained.
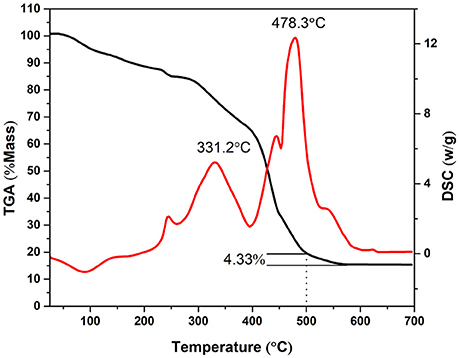
Figure 3. Thermogravimetric analysis results of the KVO precursor nanofibers in air from room temperature to 700°C. The temperature ramping rate was 10°C min−1.
The fork-like K2V8O21 prepared via electrospinning route was examined by X-ray diffraction and the result is showed in Figure 4a. The as-obtained product shows obvious XRD diffraction peaks, which illustrate the good crystallinity of the product. As for the XRD pattern, all the diffraction peaks show good agreement with K2V8O21 phase (JCPDS Card No. 24-0906). No obvious peaks from other phases can be found, demonstrating that the as-prepared K2V8O21 is of high purity. It is worth noting that the diffraction pattern of K2V8O21 has not been fully studied so far since the structure of this material was found.
The structure of the as-prepared fork-like K2V8O21 was further examined by transmission electron microscopy (TEM). The TEM image in Figure 4b shows the morphology of the endpoint of the obtained K2V8O21. It can be confirmed that the fork-like nanostructure is consistent with the SEM observation (Figure 2c). As is shown in Figure 4c, a detailed examination of a KVO folk reveals the broken tips of the K2V8O21 folk and the endpoint of K2V8O21 are transparent under the electron beam, implying an ultrathin property of the nanorods. Moreover, it is distinct that the nanorods exhibit layer-by-layer stacked structures, which is beneficial to the lithium intercalation and de-intercalation during the charge-discharge process (Pan et al., 2011b). The selected area electron diffraction (SAED) pattern is displayed in Figure 4d, which indicates that the as-prepared K2V8O21 nanorods are single-crystalline which is consistent with a previous report (An et al., 2010). It is generally accepted that single-crystalline nanostructured materials hold no significant grain boundaries with few defects, which facilitates the Li-ion diffusion during electrochemical reactions because Li ions need not to go across grain boundary and defects (Liang et al., 2007; Chen, 2013).
X-ray photoelectron spectroscopy (XPS) was further conducted to prove the chemical components and element valences of fork-like K2V8O21. As shown in Figure 5A, full survey spectrum reveals the existence of potassium, vanadium, oxygen and carbon elements in the as-prepared product. The peaks at 530.2, 517.2, 292.6, and 284.8 eV can be ascribed to O 1s, V 2p3/2, K 2p3/2, and C 1s, respectively. Correspondingly, the peaks at 292.6 and 295.4 eV which are 2.8 eV apart in the high-resolution spectrum of K 2p (Figure 5B) could be ascribed to K 2p3/2 and K 2p1/2 of K+ (Li et al., 2001). As shown in Figure 5C, the characteristic doublet of potassium vanadate V 2p is found at 517.6 and 524.7 eV, revealing that the fork-like K2V8O21 only contains V5+ valence state and no V4+ can be detected (Silversmit et al., 2004). In the broad spectrum (Figure 5A), some C element was observed and in the high-resolution spectrum of C 1s (Figure 5D), the peaks centered at 284.8, 286.4 and 288.5 eV were due to the C-C, C-O and C = O bonds, respectively. The existence of C element derived from PVP was partially retained after annealing.
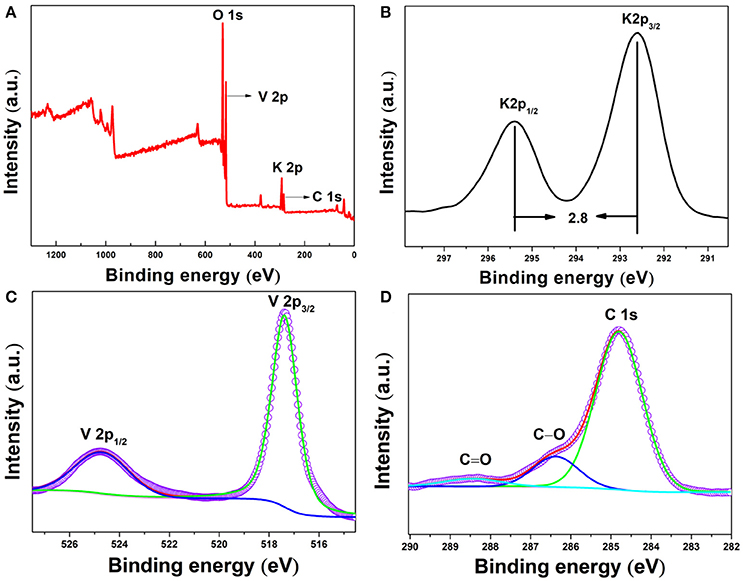
Figure 5. XPS spectra for K2V8O21 compound: (A) survey scan spectrum and high-resolution spectra of (B) K 2p, (C) V 2p and (D) C 1s.
The electrochemical properties of the electrospun fork-like K2V8O21 as cathode are evaluated by assembling CR2016 type coin half lithium-ion batteries. The cyclic voltammetry (CV) curves of K2V8O21 electrode is recorded in a voltage window of 1.5–4.0 V at a scan rate of 0.1 mV s−1. As shown in Figure 6A, two main reduction peaks located at 2.49 V and 2.82 V are ascribed to the intercalation of the lithium ions into K2V8O21 electrode in several steps. Moreover, a broad peak at about 1.75 V is also detected, which suggest further intercalation of lithium ions into the electrode material. During the anodic scan, two main oxidation peaks near 2.70 V and 3.05 V are well detected, which can be attributed to the de-intercalation of the lithium ions (Manev et al., 1993). According to the three consecutive cycles, the electrode materials has the structural reversibility due to the redox peak positions do not change much, although the peak current intensity decreases gradually. The intercalation/de-intercalation behavior of lithium in the cycling process of K2V8O21 electrode can be expressed as equation (1):
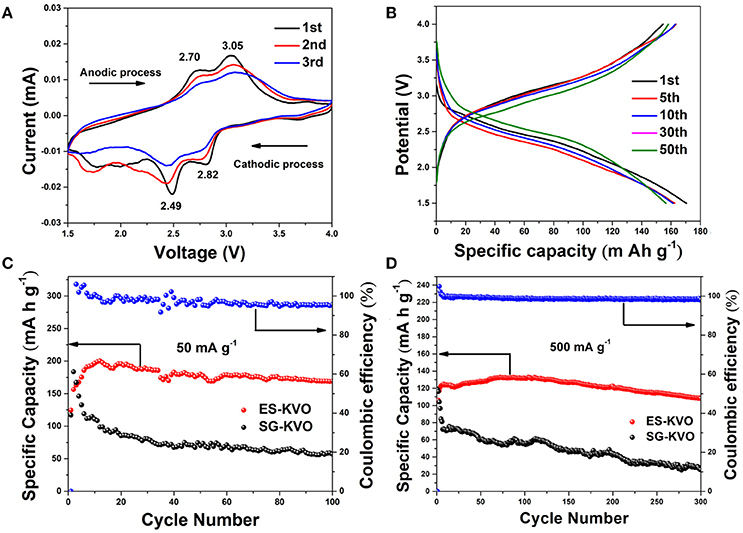
Figure 6. (A) The first three CV curves at a scan rate of 0.1 mV s−1 within a voltage range of 1.5–4.0 V and (B) GCD profiles of 1st, 5th, 10th, 30th, and 50th cycle at 100 mA g−1 of the K2V8O21 electrode; the cycling performances of ES-KVO and SG-KVO electrodes at the current density of (C) 50 mA g−1 and (D) 500 mA g−1.
Based on the previous reports on the voltage window and CV curves (Figure 6A), 1.5–4 V was chose as voltage window (Ni et al., 2015). Figure 6B shows the typical charge-discharge voltage profiles of K2V8O21 electrode and enumerates the 1st, 5th, 10th, 30th, and 50th cycles at a constant current density of 100 mA g−1. There are two voltage plateaus in both charge and discharge curves as shown in Figure 6B. In discharge process, there are two discharge plateaus at around 2.5 and 2.8 V, respectively, which are consistent with the previous CV curves (Figure 6A) that is due to the lithium-ion intercalation process. These charge/discharge profiles in different cycles have shown similar shapes, which demonstrate the good structural stability of this material. It is worth noting that, although the capacity fades upon cycling, the overpotentials on both charging and discharging are reduced, this can compensate the loss in the energy density upon cycling. So the energy efficiency of the 5th, 10th, 15th, 30th, and 50th cycles at a constant current density of 100 mA g−1 was calculated according to the following equation (2) (Eftekhari, 2017):
At various cycles of 5th, 10th, 15th, 30th, and 50th, the electrode exhibits energy efficiencies of 69.19, 71.15, 74.52, 77.55, and 78.02%, respectively. The improvement of Li+ diffusion may cause the energy efficiency increase during the cycling process, which is beneficial to use K2V8O21 as a cathode material. Figure 6C displays the cycling performance and coulombic efficiency of the electrospun K2V8O21 and sol-gel K2V8O21 at 50 mA g−1, respectively. An initial specific discharge capacity of 124.6 mA h g−1 is obtained from ES-KVO electrode and then the capacity increased gradually to reach a maximum value of 200.2 mA h g−1 at the 12th cycle. The activation process and the wetting of active electrode at the beginning may give rise to a capacity increase. After 100 cycles, the ES-KVO cathode still retains a discharge capacity of 169.2 mA h g−1 with an average capacity fading rate of 0.037% per cycle based on the discharge capacity of 5th cycle. Furthermore, the coulombic efficiency can be maintained above 95%. For comparison, the SG-KVO electrode can only deliver a maximum specific discharge capacity of 183.6 mA h g−1 and fade rapidly to <100 mA h g−1. It can be demonstrated from the above results that the as-obtained electrospun K2V8O21 electrode has shown a better cycling stability.
The long-term cycling performance of K2V8O21 electrode is also examined. The long-term cycling performance and coulombic efficiency of electrospun K2V8O21 and sol-gel K2V8O21 electrode at a same current density of 500 mA g−1 are shown in Figure 6D. Similar to the cycling performances performed at 50 mA g−1, the capacity of ES-KVO electrode increased in the initial several cycles at 500 mA g−1. This may be caused by the activation at the beginning and the improvement of lithium ion accessibility in the electrode materials during the cycling process. Although the initial discharge capacity of the cell is 120.8 mA h g−1, then the specific capacity increases slowly to reach a maximum value of 131.5 mA h g−1 at 72th cycle. After 300 cycles, a discharge capacity of 108.3 mA h g−1 still can be retained with a fading rate of only 0.043 % per cycle based on the capacity of 4th cycle. Besides, a high coulombic efficiency ~98% can be reached throughout the cycling, which shows a good reversibility of this K2V8O21 electrode at a high current rate. The initial coulombic efficiency of the K2V8O21 cathode was 114.07 %, which may be caused by the partial removal of K+ ions from the electrode materials during the charge process at initial cycle. An initial discharge capacity of SG-KVO electrode about 118.7 mA h g−1 is obtained but then dropping rapidly for subsequent cycles. After 300 cycles, the sol-gel electrode can only deliver a low discharge capacity of 25.2 mA h g−1, showing a poor long-term cycling stability. The fork-like K2V8O21 prepared by electrospinning has displayed superior specific capacity and long-term cycling stability compared to the SG-KVO, implying that it is a promising candidate for lithium-ion batteries as a cathode material. Moreover, Table S1 (Supplementary Material) summaries many reported electrochemical performance of metal vanadium oxides. Based on the comparison result, the reported fork-like K2V8O21 in this work shows excellent cycle performance when it was used as a cathode material for LIBs.
As discussed above, the electrospun fork-like K2V8O21 has demonstrated superior electrochemical performance, which may be attributed to the following reasons: (1) the morphology of layer-by-layer stacked fork-like nanostructure is propitious to lithium-ion diffusion and has enlarged the contact area between electrode and electrolyte; (2) the large space between the fork-like K2V8O21 could promote the diffusion of lithium-ion and buffer the volume change during the electrochemical reactions; (3) the single crystalline property of K2V8O21 can offer convenient pathways for lithium ion diffusion to increase the efficiency of lithiation and delithiation.
Conclusions
In summary, we have successfully prepared a single crystalline fork-like K2V8O21 via facile electrospinning method followed by an annealing process in air at 500°C for 2 h. The single crystalline fork-like K2V8O21 has shown layer-by-layer stacked nanostructure with large space and conductive carbon through heat treatment of KVO precursor. Due to this advantageous feature, the fork-like K2V8O21 demonstrates excellent electrochemical performances including high specific discharge capacity of 200.2 mA h g−1 at a current density of 50 mA g−1 with a good capacity retention (96.52%) after 100 cycles. Moreover, the electrodes demonstrate superior long-term cycling stability up to 300 cycles at a current density of 500 mA g−1. The results from our work have demonstrated that the as-electrospun K2V8O21 is a promising cathode candidate for next-generation high-performance LIBs.
Author Contributions
PH did the main experiment and write the manuscript. TZ involved in the discussion of the experiment and revised the manuscript. QS and JL did the SEM experiment. RC assisted the material synthesis. XC, YW, and AP made the research plan. AP also provided the financial support.
Conflict of Interest Statement
The authors declare that the research was conducted in the absence of any commercial or financial relationships that could be construed as a potential conflict of interest.
Acknowledgments
This work was supported by Natural Science Foundation of Hunan Province (2018JJ1036), China, and Innovation-driven Program of Central South University (2017CX001).
Supplementary Material
The Supplementary Material for this article can be found online at: https://www.frontiersin.org/articles/10.3389/fchem.2018.00195/full#supplementary-material
References
Aleksandrova, A., Uzunova, S., Stankulov, T., and Momchilov, A (2006). Nano-sized vanadium bronzes as cathode material for rechargeable Li batteries. Funct. Propert. Nanostruct. Mater. 223, 479–484. doi: 10.1007/1-4020-4594-8_47
Aleksandrova, A., Uzunov, I., Banov, B., and Momchilov, A. (2009). Potassium bronzes as active material for Li-ion batteries. Compt. Rend. Acad. Bulg. 62, 453–460.
An, J. N., Xu, C. Y., Zhen, L., and Huang, Y. D. (2010). Surfactant-free hydrothermal synthesis and characterization of single-crystal K2V8O21 nanobelts. Ceram. Int. 36, 1825–1829. doi: 10.1016/j.ceramint.2010.03.031
An, X., Yang, H., Wang, Y., Tang, Y., Liang, S., Pan, A., et al. (2017). Hydrothermal synthesis of coherent porous V2O3/carbon nanocomposites for high-performance lithium- and sodium-ion batteries. Sci. China Mater. 60, 717–727. doi: 10.1007/s40843-017-9054-0
Armand, M., and Tarascon, J.-M. (2008). Building better batteries. Nature 451, 652–657. doi: 10.1038/451652a
Azhari, S. J., and Dish, M. A. (1998). Thermal degradation and stability of poly(4-vinylpyridine) homopolymer and copolymers of 4-vinylpyridine with methyl acrylate. Polym. Degrad. Stabil. 69, 253–256. doi: 10.1016/S0141-3910(97)00073-6
Bach, S., Boudaoud, A., Emery, N., Baddour-Hadjean, R., and Pereira-Ramos, J. P. (2014). K0.5V2O5: a novel Li intercalation compound as positive electrode material for rechargeable lithium batteries. Electrochim. Acta 119, 38–42. doi: 10.1016/j.electacta.2013.12.039
Baddour-Hadjean, R., Bach, S., Emery, N., and Pereira-Ramos, J. P. (2011). The peculiar structural behaviour of β-Na0.33V2O5 upon electrochemical lithium insertion. J. Mater. Chem. 21, 11296–11305. doi: 10.1039/C1JM11393C
Baddour-Hadjean, R., Boudaoud, A., Bach, S., Emery, N., and Pereira-Ramos, J. P. (2014). A comparative insight of potassium vanadates as positive electrode materials for Li batteries: influence of the long-range and local structure. Inorg. Chem. 53, 1764–1772. doi: 10.1021/ic402897d
Cao, A. M., Hu, J. S., Liang, H. P., and Wan, L. J. (2005). Self-assembled vanadium pentoxide (V2O5) hollow microspheres from nanorods and their application in lithium-ion batteries. Angew. Chem. Int. Ed. 44, 4391–4395. doi: 10.1002/anie.200500946
Chen, J. (2013). Recent progress in advanced materials for lithium ion batteries. Materials 6, 156–183. doi: 10.3390/ma6010156
Cheng, F., and Chen, J. (2011). Transition metal vanadium oxides and vanadate materials for lithium batteries. J. Mater. Chem. 21, 9841–9848. doi: 10.1039/c0jm04239k
Eftekhari, A. (2017). Energy efficiency: a critically important but neglected factor in battery research. Sustain. Energy Fuels 1, 2053–2060. doi: 10.1039/C7SE00350A
Fang, G., Zhou, J., Hu, Y., Cao, X., Tang, Y., and Liang, S. (2015). Facile synthesis of potassium vanadate cathode material with superior cycling stability for lithium ion batteries. J. Power Sources 275, 694–701. doi: 10.1016/j.jpowsour.2014.11.052
Fang, G., Zhou, J., Liang, C., Cai, Y., Pan, A., Tan, X., et al. (2016). General synthesis of three-dimensional alkali metal vanadate aerogels with superior lithium storage properties. J. Mater. Chem. A 4, 14408–14415. doi: 10.1039/C6TA05568K
Goodenough, J. B., and Kim, Y. (2010). Challenges for rechargeable li batteries. Chem. Mater. 22, 587–603. doi: 10.1021/cm901452z
Gu, X., Yue, J., Chen, L., Liu, S., Xu, H., Yang, J., et al. (2015). Coaxial MnO/N-doped carbon nanorods for advanced lithium-ion battery anodes. J. Mat. Chem. A 3, 1037–1041. doi: 10.1039/C4TA05622A
Jian, X. M., Wenren, H. Q., Huang, S., Shi, S. J., Wang, X. L., Gu, C. D., et al. (2014). Oxalic acid-assisted combustion synthesized LiVO3 cathode material for lithium ion batteries. J. Power Sources 246, 417–422. doi: 10.1016/j.jpowsour.2013.07.110
Khoo, E., Wang, J., Ma, J., and Lee, P. S. (2010). Electrochemical energy storage in a β-Na0.33V2O5 nanobelt network and its application for supercapacitors. J. Mat. Chem. 20, 8368–8374. doi: 10.1039/C0JM00652A
Ko, Y. W., Teh, P. F., Pramana, S. S., Wong, C. L., Su, T., Li, L., et al. (2015). Electrospun single-phase Na1.2V3O8 materials with tunable morphologies as cathodes for rechargeable Lithium-Ion batteries. ChemElectroChem 2, 837–846. doi: 10.1002/celc.201500023
Li, S., Kang, E. T., Neoh, K. G., Ma, Z. H., Tan, K. L., and Huang, W. (2001). In situ XPS studies of thermally deposited potassium on poly(p-phenylene vinylene) and its ring-substituted derivatives. Appl. Surf. Sci. 181, 201–210. doi: 10.1016/S0169-4332(01)00397-X
Li, W., Zeng, L., Wu, Y., and Yu, Y. (2016). Nanostructured electrode materials for lithium-ion and sodium-ion batteries via electrospinning. Sci. China Mater. 59, 287–321. doi: 10.1007/s40843-016-5039-6
Liang, S., Zhou, J., Fang, G., Liu, J., Tang, Y., Li, X., et al. (2013a). Ultrathin Na1.1V3O7.9 nanobelts with superior performance as cathode materials for lithium-ion batteries. ACS Appl. Mater. Interfaces 5, 8704–8709. doi: 10.1021/am402352q
Liang, S., Zhou, J., Fang, G., Zhang, C., Wu, J., Tang, Y., et al. (2014). Synthesis of mesoporous β-Na0.33V2O5 with enhanced electrochemical performance for lithium ion batteries. Electrochim. Acta 130, 119–126. doi: 10.1016/j.electacta.2014.02.131
Liang, S., Zhou, J., Pan, A., Zhang, X., Tang, Y., Tan, X., et al. (2013b). Facile synthesis of Ag/AgVO3 hybrid nanorods with enhanced electrochemical performance as cathode material for lithium batteries. J. Power Sources 228, 178–184. doi: 10.1016/j.jpowsour.2012.11.104
Liang, Y., Fan, J., Xia, X., and Jia, Z. (2007). Synthesis and characterisation of SnO2 nano-single crystals as anode materials for lithium-ion batteries. Mater. Lett. 61, 4370–4373. doi: 10.1016/j.matlet.2007.02.008
Liu, H., and Tang, D. (2009). Synthesis of ZnV2O6 powder and its cathodic performance for lithium secondary battery. Mater. Chem. Phys. 114, 656–659. doi: 10.1016/j.matchemphys.2008.10.055
Liu, H., and Yang, W. (2011). Ultralong single crystalline V2O5 nanowire/graphene composite fabricated by a facile green approach and its lithium storage behavior. Energy Environ. Sci. 4, 4000–4008. doi: 10.1039/c1ee01353j
Liu, L., Jiao, L., Sun, J., Zhang, Y., Zhao, M., Yuan, H., et al. (2009). Electrochemical properties of submicron-sized LiV3O8 synthesized by a low-temperature reaction route. J. Alloy. Compd. 471, 352–356. doi: 10.1016/j.jallcom.2008.03.096
Ma, H., Zhang, S., Ji, W., Tao, Z., and Chen, J. (2008). r-CuV2O6 Nanowires: hydrothermal synthesis and primary lithium battery application. J. Am. Chem. Soc. 13, 5361–5367. doi: 10.1021/ja800109u
Mai, L., Xu, L., Han, C., Xu, X., Luo, Y., Zhao, S., et al. (2010a). Cucumber-Like V2O5/poly(3,4-ethylenedioxythiophene)&MnO2 Nanowires with Enhanced Electrochemical Cyclability. Nano Lett. 10, 4750–4755. doi: 10.1021/nl304434v
Mai, L., Xu, L., Han, C., Xu, X., Luo, Y., Zhao, S., et al. (2010b). Electrospun ultralong hierarchical vanadium oxide nanowires with high performance for lithium ion batteries. Nano Lett. 10, 4750–4755. doi: 10.1021/nl103343w
Manev, V., Momchilov, A., Nassalevska, A., Pistoia, G., and Pasquali, M. (1993). Potassium vanadates–promising materials for secondary lithium batttries. J. Power Sources 44, 561–568. doi: 10.1016/0378-7753(93)80203-2
Maingot, S., Baddour, R., Pereira-Ramos, J. P., Baffier, N., and Willmann, R. (1993). A new Iron V2O5 bronze as electrode material for rechargeable lithium batteries. J. Electrochem. Soc. 140, L158–L160.
Meng, J., Liu, Z., Niu, C., Xu, X., Liu, X., Zhang, G., et al. (2016). A synergistic effect between layer surface configurations and K ions of potassium vanadate nanowires for enhanced energy storage performance. J. Mater. Chem. A 4, 4893–4899. doi: 10.1039/C6TA00556J
Ng, S. H., Chew, S. Y., Wang, J., Wexler, D., Tournayre, Y., Konstantinov, K., et al. (2007). Synthesis and electrochemical properties of V2O5 nanostructures prepared via a precipitation process for lithium-ion battery cathodes. J. Power Sources 174, 1032–1035. doi: 10.1016/j.jpowsour.2007.06.166
Ni, E. F., Goto, S., Quan, Z., and Sonoyama, N. (2015). Electrochemical property for the metal-doped vanadium Bronze K2V8O21 as a cathode material of lithium battery. Electrochemistry 83, 902–908. doi: 10.5796/electrochemistry.83.902
Ou, X., Liang, X., Zheng, F., Pan, Q., Zhou, J., Xiong, X., et al. (2017). Exfoliated V5S8/graphite nanosheet with excellent electrochemical performance for enhanced lithium storage. Chem. Eng. J. 320, 485–493. doi: 10.1016/j.cej.2017.03.069
Owens, B. B., Passerini, S., and Smyrl, W. H. (1999). Lithium ion insertion in porous metal oxides. Electrochim. Acta. 45, 215–224. doi: 10.1016/S0013-4686(99)00205-4
Pan, A., Liu, J., Zhang, J.-G., Cao, G., Xu, W., Nie, Z., et al. (2011a). Template free synthesis of LiV3O8 nanorods as a cathode material for high-rate secondary lithium batteries. J. Mater. Chem. 21, 1153–1161. doi: 10.1039/C0JM02810J
Pan, A., Zhang, J. G., Cao, G., Liang, S., Wang, C., Nie, Z., et al. (2011b). Nanosheet-structured LiV3O8 with high capacity and excellent stability for high energy lithium batteries. J. Mater. Chem. 21, 10077–10084. doi: 10.1039/C1JM10976F
Pan, Q., Zheng, F., Ou, X., Yang, C., Xiong, X., and Liu, M. (2017a). MoS2 encapsulated SnO2-SnS/C nanosheets as a high performance anode material for lithium ion batteries. Chem. Eng. J. 316, 393–400. doi: 10.1039/C5TA05011A
Pan, Q., Zheng, F., Ou, X., Yang, C., Xiong, X., Tang, Z., et al. (2017b). MoS2 decorated Fe3O4/Fe1–xS@C nanosheets as high-performance anode materials for lithium Ion and sodium ion batteries. ACS Sustain. Chem. Eng. 5, 4739–4745. doi: 10.1021/acssuschemeng.7b00119
Rui, X., Zhu, J., Sim, D., Xu, C., Zeng, Y., Hng, H. H., et al. (2011). Reduced graphene oxide supported highly porous V2O5 spheres as a high-power cathode material for lithium ion batteries. Nanoscale 3, 4752–4758. doi: 10.1039/c1nr10879d
Silversmit, G., Depla, D., Poelman, H., Marin, G. B., and De Gryse, R. (2004). Determination of the V2p XPS binding energies for different vanadium oxidation states (V5+ to V0+). J. Electron Spectrosc. Relat. 135, 167–175. doi: 10.1016/j.elspec.2004.03.004
Teh, P. F., Sharma, Y., Ko, Y. W., Pramana, S. S., and Srinivasan, M. (2013). Tuning the morphology of ZnMn2O4 lithium ion battery anodes by electrospinning and its effect on electrochemical performance. RSC Adv. 3, 2812–2821. doi: 10.1039/c2ra22943a
Torardi, C. C., and Miao, C. R. (2002). New battery cathode materials: synthesis, characterization, and electrochemical performance of M1-xV3O8−yFz,nH2O (M = NH4, K). Chem. Mater. 14, 4430–4433. doi: 10.1021/cm020620u
Varadaraajan, V., Satishkumar, B. C., Nanda, J., and Mohanty, P. (2011). Direct synthesis of nanostructured V2O5 films using solution plasma spray approach for lithium battery applications. J. Power Sources 196, 10704–10711. doi: 10.1016/j.jpowsour.2011.09.016
Wang, H., Huang, K., Huang, C., Liu, S., Ren, Y., and Huang, X. (2011). (NH4)0.5V2O5 nanobelt with good cycling stability as cathode material for Li-ion battery. J. Power Sources 196, 5645–5650. doi: 10.1016/j.jpowsour.2011.02.046
Wang, Y., Takahashi, K., Lee, K. H., and Cao, G. Z. (2006). Nanostructured vanadium oxide electrodes for enhanced Lithium-Ion intercalation. Adv. Funct. Mater. 16, 1133–1144. doi: 10.1002/adfm.200500662
Wang, Z., Liu, X., Lv, M., Chai, P., Liu, Y., and Meng, J. (2008). Preparation of ferrite MFe2O4 (M = Co, Ni) ribbons with nanoporous structure and their magnetic properties. J. Phys. Chem. 112, 11292–11297. doi: 10.1021/jp804178w
Wee, G., Soh, H. Z., Cheah, Y. L., Mhaisalkar, S. G., and Srinivasan, M. (2010). Synthesis and electrochemical properties of electrospun V2O5 nanofibers as supercapacitor electrodes. J. Mat. Chem. 20, 6720–6725. doi: 10.1039/c0jm00059k
Whittingham, M. S. (2004). Lithium batteries and cathode materials. Chem. Rev. 104, 4271–4301. doi: 10.1021/cr020731c
Xu, K., Hu, S., Wu, C., Lin, C., Lu, X., Peng, L., et al. (2012). Highly entangled K0.5V2O5 superlong nanobelt membranes for flexible nonvolatile memory devices. J. Mat. Chem. 22, 18214–18220. doi: 10.1039/c2jm34031c
Xu, M., Han, J., Li, G., Niu, Y., Liu, S., Hou, J., et al. (2015). Synthesis of novel book-like K0.23V2O5 crystals and their electrochemical behavior in lithium batteries. Chem. Commun. 51, 15290–15293. doi: 10.1039/C5CC05425G
Yang, C., Ou, X., Xiong, X., Zheng, F., Hu, R., Chen, Y., et al. (2017). V5S8-graphite hybrid nanosheets as a high rate-capacity and stable anode material for sodium-ion batteries. Energy Environ. Sci. 10, 107–113. doi: 10.1039/C6EE03173K
Yang, K., Fang, G., Zhou, J., Qin, M., Tang, Y., Pan, A., et al. (2016). Hydrothermal synthesis of sodium vanadate nanobelts as high-performance cathode materials for lithium batteries. J. Power Sources 325, 383–390. doi: 10.1016/j.jpowsour.2016.06.023
Zhao, J., Liu, B., Xu, S., Yang, J., and Lu, Y. (2015). Fabrication and electrochemical properties of porous VN hollow nanofibers. J. Alloy. Compd. 651, 785–792. doi: 10.1016/j.jallcom.2015.06.111
Zheng, F., Yang, C., Xiong, X., Xiong, J., Hu, R., Chen, Y., et al. (2015). Nanoscale surface modification of lithium-Rich layered-oxide composite cathodes for suppressing voltage fade. Angew. Chem. Int. Ed Engl. 54, 13058–13062. doi: 10.1002/anie.201506408
Keywords: potassium vanadate, electrospinning, fork-like nanostructure, cathode materials, lithium-ion batteries
Citation: Hao P, Zhu T, Su Q, Lin J, Cui R, Cao X, Wang Y and Pan A (2018) Electrospun Single Crystalline Fork-Like K2V8O21 as High-Performance Cathode Materials for Lithium-Ion Batteries. Front. Chem. 6:195. doi: 10.3389/fchem.2018.00195
Received: 06 April 2018; Accepted: 14 May 2018;
Published: 01 June 2018.
Edited by:
Kaili Zhang, City University of Hong Kong, Hong KongReviewed by:
Chaopeng Fu, Shanghai Jiao Tong University, ChinaLing Wu, Soochow University, China
Chenghao Yang, South China University of Technology, China
Ali Eftekhari, Queen's University Belfast, United Kingdom
Copyright © 2018 Hao, Zhu, Su, Lin, Cui, Cao, Wang and Pan. This is an open-access article distributed under the terms of the Creative Commons Attribution License (CC BY). The use, distribution or reproduction in other forums is permitted, provided the original author(s) and the copyright owner are credited and that the original publication in this journal is cited, in accordance with accepted academic practice. No use, distribution or reproduction is permitted which does not comply with these terms.
*Correspondence: Anqiang Pan, cGFuYW5xaWFuZ0Bjc3UuZWR1LmNu