- 1School of Physical Science & Engineering and Key Laboratory of Materials Physics of Ministry of Education, Zhengzhou University, Zhengzhou, China
- 2College of Science, Zhongyuan University of Technology, Zhengzhou, China
- 3Department of Physics and Electronic Engineering, Zhoukou Normal University, Zhoukou, China
The effects of Zn2+ incorporation on the phase formation, thermal expansion, phase transition, and vibrational properties of HfMg1−xZnxMo3O12 are investigated by XRD, dilatometry, and Raman spectroscopy. The results show that (i) single phase formation is only possible for x ≤ 0.5, otherwise, additional phases of HfMo2O8 and ZnMoO4 appear; (ii) The phase transition temperature from monoclinic to orthorhombic structure of the single phase HfMg1−xZnxMo3O12 can be well-tailored, which increases with the content of Zn2+; (iii) The incorporation of Zn2+ leads to an pronounced reduction in the positive expansion of the b-axis and an enhanced negative thermal expansion (NTE) in the c-axes, leading to a near-zero thermal expansion (ZTE) property with lower anisotropy over a wide temperature range; (iv) Replacement of Mg2+ by Zn2+ weakens the Mo–O bonds as revealed by obvious red shifts of all the Mo–O stretching modes with increasing the content of Zn2+ and improves the sintering performance of the samples which is observed by SEM. The mechanisms of the negative and near-ZTE are discussed.
Introduction
Large difference in coefficients of thermal expansion (CTE) of materials could lead to performance deterioration and even failure of devices due to thermal stress when temperature changes abruptly or frequently. Since most materials expand on heating and contract on cooling, materials with opposite property, namely negative thermal expansion (NTE), are particularly desired for tailoring CTEs. The rediscovery of NTE in ZrW2O8 in a wide temperature range (Evans et al., 1996, 1997a) triggered continuous efforts on understanding the NTE phenomenon and searching for more NTE materials (Yang et al., 2007; Chen et al., 2013, 2015; Tallentire et al., 2013; Lama et al., 2014; Liu et al., 2014; Peng et al., 2014; Xiao et al., 2014; Hu et al., 2015). To date, different families of NTE materials based on various mechanisms, such as the phonon effect (Pryde et al., 1996; Wang et al., 2011; Bridges et al., 2014; Cheng et al., 2016a; Ge et al., 2016a), magnetovolume effect (Takenaka and Takagi, 2005; Qu et al., 2012; Yan et al., 2014), spontaneous ferroelectric polarization (Chen et al., 2013; Peng et al., 2016), and charge transfer (Long et al., 2009; Azuma et al., 2011; Yamada et al., 2016) have been reported. Among the materials, the family of A2M3O12 (A = transition metal or a mixture of tetravalent and bivalent cations, M = W, Mo) have been particularly attractive, because whose NTEs go over a wide temperature range and can be tuned from low positive to large negative values due to chemical flexibility (Evans et al., 1997b; Suzuki and Omote, 2006; Wu et al., 2009, 2012, 2014; Li et al., 2011; Das et al., 2013; Miller et al., 2013; Song et al., 2014a; Liu et al., 2015; Chen et al., 2016; Cheng et al., 2016a).
In recent years, a number of novel NTE materials have been designed based on the basic structure of A2M3O12 family, including those with a general formula ABM3O12 where A is tetravalent Hf4+ or Zr4+ and B is bivalent cation Mg2+ or Mn2+, and M is W or Mo or a combination of them (Suzuki and Omote, 2004; Baiz et al., 2008; Gindhart et al., 2008; Marinkovic et al., 2008; Song et al., 2013; Li et al., 2014, 2016, 2017; Ge et al., 2016a; Liu et al., 2018) and those with a formula ABM2XO12 where A and M are the same as in ABM3O12, B is a trivalent cation and X is P5+ or V5+ (Chen et al., 2016; Cheng et al., 2016b, 2017; Ge et al., 2016b,c). The most distinct characteristics of the materials with formula ABM2XO12 are that they exhibit NTE over a wide temperature range and intense photoluminescence in the visible range. Nearly an order higher ionic conductivity was observed for HfMgW3O12 with respect to the family A2M3O12 (Omote et al., 2011). HfMgMo3O12 with a linear CTE of 1.02 × 10−6 K−1 from 298 to 1013 K was reported by Marinkovic et al. (2008). It crystallizes in orthorhombic symmetry with space group Pnma(62) or Pna21 (33) and transforms to monoclinic structure at 175 K (Miller et al., 2012).
In this paper, we investigate the effects of Zn2+ incorporation on the structure, phase transition, thermal expansion, and vibrational properties of HfMgMo3O12. It is shown that single phase solid solution of HfMg1−xZnxMo3O12 can be achieved only for the compositions of x ≤ 0.5, otherwise, additional phases of HfMo2O8 and ZnMoO4 appear. The monoclinic to orthorhombic phase transition temperature increases with the content of Zn2+ for x ≤ 0.5 so that HfMg0.5Zn0.5Mo3O12 crystallizes in monoclinic phase and all other samples (x ≤ 0.4) adopt orthorhombic structure at room temperature (RT). The incorporation of Zn2+ alters the axial CTE differently for each axis and finally results in near-zero thermal expansion (ZTE) property over wide temperature ranges with smaller thermal expansion anisotropy with respect to HfMgMo3O12. The mechanisms of Zn2+ incorporation on the phase transition, thermal expansion and vibrational properties are discussed.
Experimental
Analytic grade reagents of HfO2, MgO, ZnO, and MoO3 were mixed with stoichiometric ratios for HfMg1−xZnxMo3O12 with x = 0.0, 0.1, 0.2, 0.3, 0.4, 0.5, 0.6, 0.7, 0.8, and 1.0. The mixtures were ground in an agate mortar for 2 h, then, pressed under 325 MPa into cylinders with diameter of 10 mm and height of 6 mm using a uniaxial tablet machine. The cylinders were sintered at 1,073 K for 5 h in a muffle furnace in air and cooled down to 300 K naturally.
The as-prepared samples were analyzed by XRD with a PANalytical X'Pert PRO X-ray Diffractometer to identify the crystalline phase. Variable-temperature X-ray powder data were collected on a Rigaku (Japan, SmartLab 3KW) diffractometer with Cu Kα (λ = 0.15405 nm) radiation. Diffraction data were collected with a step size of 0.01° in the 2θ range of 10°–120°. The sample was heated at a rate of 10 K/min and remained at each measurement temperature for 5 min before measurement. Unit cell dimensions above the phase transition temperature were determined with software of PowderX. Variable-temperature/RT Raman spectra were recorded with A LabRAM HR Evolution Raman spectrometer (France HORIBA JobinYvon S.A.A.) equipped with a Linkam THMS600 Heating and Freezing Stage (Japan Hightech) (an accuracy of ±0.1 K). The excitation wavelength is 633 nm and low excitation laser power is necessary to avoid local heating by the laser. The microstructures and energy dispersive spectra of the samples were examined with a scanning electron microscope (SEM, Model Quanta 250). The relative length changes were measured with LINSEIS DIL L75 dilatometer at the heating and cooling rates of 5 K/min.
Results and Discussion
Figure 1A shows the XRD patterns of the solid solutions of HfMg1−xZnxMo3O12. When x = 0.0, the diffraction peaks are corresponding to HfMgMo3O12, which adopts an orthorhombic structure with space group Pnma or Pna21 (Marinkovic et al., 2008). No obvious changes in the XRD patterns could be observed with increasing the content of Zn2+ till x = 0.4. It is reasonable to conclude that HfMg1−xZnxMo3O12 for x ≤ 0.4 crystallized in an orthorhombic structure. Nevertheless, some subtle changes are observed for x = 0.5, such as the weak peak appearing at about 25.6° which is characteristic for a monoclinic structure (Song et al., 2014b; Ge et al., 2016a) of ABMo3O12. HfMg0.5Zn0.5Mo3O12 at RT is thus identified as a monoclinic structure. The XRD patterns change obviously with further increasing content of Zn2+. Detailed analyses show that the newly appeared peaks correspond well to HfMo2O8 and ZnMoO4 (Reichelt et al., 2000; Allen et al., 2004), respectively.
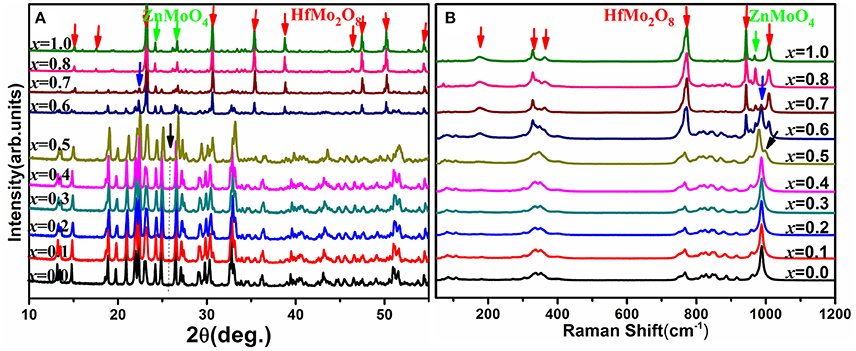
Figure 1. (A) X-ray diffraction patterns of the solid solutions of HfMg1−xZnxMo3O12; (B) Raman spectra of the solid solutions of HfMg1−xZnxMo3O12.
The above analysis is supported by Raman spectroscopic analysis (Figure 1B). The Raman spectra are consistent with each other for x ≤ 0.4 while the Raman band at 988 cm−1 splits into two bands at 980 and 998 cm−1 for x = 0.5 (as indicated by the black arrowhead), which is characteristic for a phase transition from higher orthorhombic symmetry to lower monoclinic symmetry (Li et al., 2011; Ge et al., 2016a) for the ABMo3O12 family. Distinct changes of the Raman spectra occur for higher content of Zn2+. The new Raman bands at about 175, 328, 362, 772, 943, and 1,008 cm−1 correspond well to HfMo2O8 (Liang et al., 2008b) and that around 968 cm−1 arises from ZnMoO4 (Ahsaine et al., 2016). Both XRD and Raman analyses demonstrate that a single phase solid solution of HfMg1−xZnxMo3O12 is only possible for x ≤ 0.5 and additional phases of HfMo2O8 and ZnMoO4 form for x ≥ 0.6. At RT, HfMg1−xZnxMo3O12 for x ≤ 0.4 adopt an orthorhombic structure while HfMg0.5Zn0.5Mo3O12 crystallizes in a monoclinic structure.
Raman spectroscopy is very sensitive to the monoclinic-to-orthorhombic phase transition (Li et al., 2011, 2016; Ge et al., 2016a). In order to get some insights into the influence of Zn2+ on the phase transition, we carried out temperature-dependent Raman spectral observation of HfMg1−xZnxMo3O12 (x ≤ 0.5) as shown in Figure 2. The XRD analyses suggest that HfMg1−xZnxMo3O12 (x ≤ 0.5) have similar open framework structure as HfMgMo3O12. In the orthorhombic phase, there are four molecular formulas in a unit cell, in which each MoO4 tetrahedron sharing its four vortexes with HfO6/MgO6 octahedra and each HfO6/MgO6 octahedron shares its corners with six MoO4 tetrahedra. Hf and Mg are alternatively aligned in the [010] direction forming a quasi-layered structure (Omote et al., 2011). The Raman modes from 1,050 to 900 cm−1, from 900 to 750 cm−1, from 400 to 320 cm−1, and from 320 to 280 cm−1 are identified as symmetric stretching (ν1), asymmetric stretching (ν3), asymmetric bending (ν4), and symmetric bending (ν2) modes in the MoO4 tetrahedra, respectively (Liang et al., 2008a; Li et al., 2011). Figure 2A shows the temperature dependent Raman spectra of HfMgMo3O12. The most distinctive change of the Raman spectra is the disappearance of the band at about 1,001 cm−1 with temperature increase from 168 to 178 K, which can be regarded as characteristic of the phase transition from low temperature monoclinic to high temperature orthorhombic structure (Li et al., 2011; Ge et al., 2016a). The phase transition temperature agrees well with the result derived from XRD analysis (Miller et al., 2012). In Figures 2B–F we present the temperature dependent Raman spectra for Zn2+-containing samples. It is shown that the vanishing of the characteristic Raman band for the monoclinic structure occurs in the ranges of 168–178, 203–213, 223–233, 258–268, 283–293, and 318–328 K for x = 0.0, 0.1, 0.2, 0.3, 0.4, and 0.5, respectively, demonstrating that the phase transition temperature increases with the content of Zn2+.
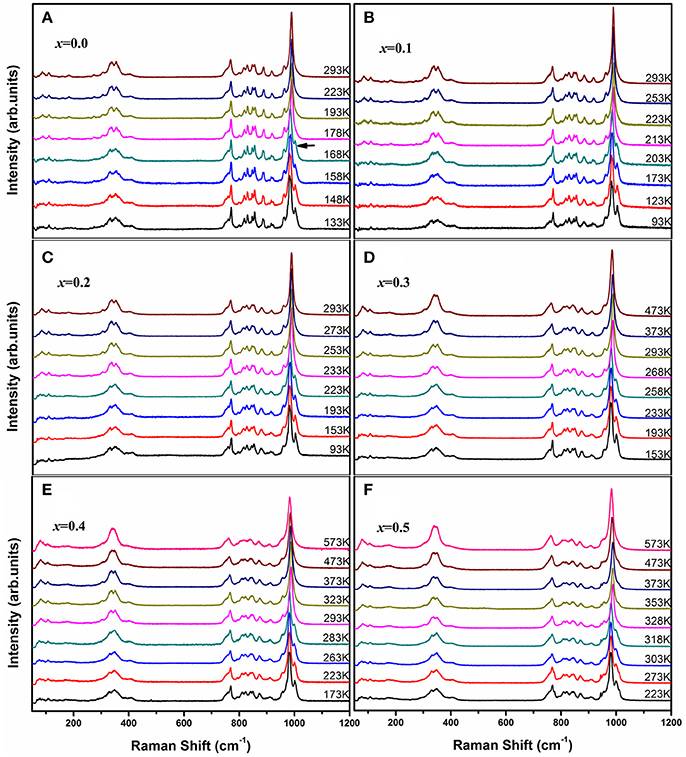
Figure 2. Temperature-dependent Raman spectra of HfMg1−xZnxMo3O12 with x = 0.0 (A), 0.1 (B), 0.2 (C), 0.3 (D), 0.4 (E), and 0.5 (F).
In the orthorhombic structure of HfMg1−xZnxMo3O12 (x ≤ 0.5), the four vortexes of each MoO4 tetrahedron are shared with two HfO6 and two MgO6/ZnO6 octahedra while each HfO6/MgO6/ZnO6 octahedron shares its corners with six MoO4 tetrahedra. Statistically, each MoO4 tetrahedron links to 0.0, 0.2, 0.4, 0.8, and 1.0 ZnO6 octahedron for x = 0.0, 0.1, 0.2, 0.3, 0.4, and 0.5. Since the ionic radius of Zn2+ is 74 pm which is slightly larger than that of Mg2+ (72 pm), large lattice distortion and increase in phase transition temperature is not expected if only the ionic radius is considered. The experimentally observed obvious increase in phase transition temperature is therefore attributed to the difference in electronegativity between Zn2+ (1.65 Pauling) and Mg2+ (1.31 Pauling). Replacement of Mg2+ by Zn2+ causes an increase in electronegativity at theZn2+-cation side and a decrease in the effective negative charge on oxygen, and hence a decrease in the oxygen-oxygen repulsion. With increasing the content of Zn2+, oxygen-oxygen attractive forces increase, causing the network collapse transition to occur at higher temperatures (Evans et al., 1997b).
Figure 3 shows the relative length changes of sintered cylinders with increasing temperature measured by dilatometry. All the samples for x ≤ 0.6 exhibit abrupt length increase around the temperature of monoclinic to orthorhombic phase transition. The phase transition temperature increases with increasing the content of Zn2+ except the one for x = 0.6 whose phase transition temperature is lower than that of x ≤ 0.5 due to the generation of HfMo2O8 and ZnMoO4. In this case, it can be deduced that the real content of Zn2+ in HfMg1−xZnxMo3O12 is lower than HfMg0.5Zn0.5Mo3O12. These results comply well with the above Raman spectroscopic analyses. The CTEs are calculated from the relative length change and shown in the Table 1. It indicates that all the single phase samples present excellent near-ZTE property above the phase transition temperature. It is interesting to notice that even for the multi-phase samples for x = 0.8 and 1.0, a near-ZTE property in a wide temperature range are realized. However, in this paper we focus on the effect of Zn2+ incorporation on the structure and properties of the single phase HfMg1−xZnxMo3O12.
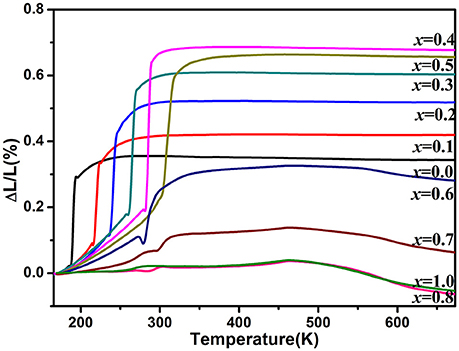
Figure 3. Variations of relative length of the solid solutions of HfMg1−xZnxMo3O12 with increasing temperature from 170 to 673 K.
In order to get insight into the axial thermal expansion property, we carried out temperature-dependent powder XRD measurements for the samples of x = 0.2 and 0.3. For comparison, variable-temperature XRD data of HfMgMo3O12 were also collected. Figure 4A shows the selected temperature-dependent XRD patterns for HfMg0.7Zn0.3Mo3O12 at different temperatures. It is obvious that its XRD pattern changes distinctively around 225 K, which is attributed to the phase transformation from lower temperature monoclinic to higher temperature orthorhombic structure. Lattice constants and cell volume at each temperature are calculated and given in Figure 4B. It is evident that the a- and c-axes contract while the b-axis expands with increasing temperature. The CTEs for the a-, b-, and c-axes and volume are calculated to be , , , , respectively. This gives rise to a linear CTE . Similar axial thermal expansion behaviors are obtained for HfMg0.8Zn0.2Mo3O12 from temperature dependent XRD measurements (not shown here). The changes of its lattice constants and volume with temperature are given in Figure 4C. The CTEs for the a-, b-, and c-axes and volume are calculated to be , , , , respectively, corresponding to a linear CTE . These results are consistent with the values measured by dilatometry, confirming HfMg0.7Zn0.3Mo3O12 and HfMg0.8Zn0.2Mo3O12 being intrinsically ZTE materials. Figure 4D shows the changes of lattice constants and volume of HfMgMo3O12 with temperature.
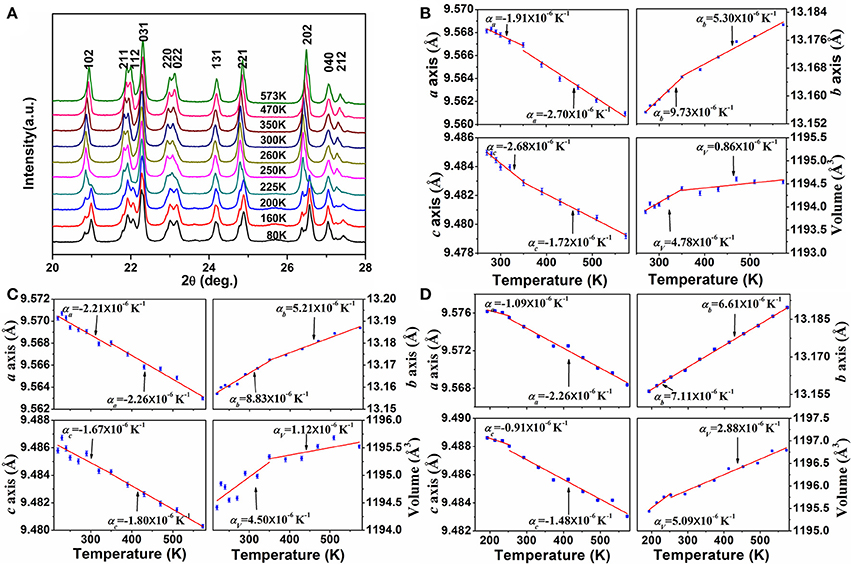
Figure 4. (A) Variable-temperature XRD patterns of HfMg0.7Zn0.3Mo3O12 (The XRD patterns were measured for 2θ = 10°-120°, here only a small range is shown for clarity); Changes of lattice constants and volume of HfMg1−xZnxMo3O12 with temperature: (B) for x = 0.3, (C) for x = 0.2, (D) for HfMgMo3O12.
Considering the fact that the intrinsic linear CTE of HfMgMo3O12 is 1.02 × 10−6 K−1 (Marinkovic et al., 2008), it is reasonable to conclude that the incorporation of Zn2+ reduces the linear CTE and results in near ZTE of HfMg1−xZnxMo3O12. A comparison of the axial CTEs for HfMgMo3O12, HfMg0.8Zn0.2Mo3O12, and HfMg0.7Zn0.3Mo3O12 are given in Table 2. It is found that partial substitution of Mg2+ by Zn2+ leads to a significant reduction of the CTE in the b-axis and an increase of the NTE in the c-axis, resulting in a near ZTE and lower anisotropy of thermal expansion in the Zn-containing compounds with respect to HfMgMo3O12 (see Table 2). The anisotropy of thermal expansion is defined as the maximum difference in the axial thermal expansion coefficients (Srikanth et al., 1992; Miller et al., 2013). The near zero linear thermal expansion and lower anisotropy property of the Zn-containing compounds suggest that they could withstand higher thermal shock resistance.
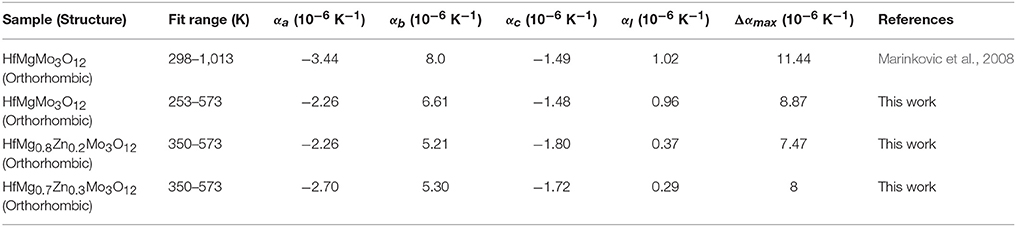
Table 2. Intrinsic thermal expansion coefficients (α) for HfMg0.7Zn0.3Mo3O12 and HfMg0.8Zn0.2Mo3O12 as obtained from variable-temperature XRD and literature and experimental values for HfMgMo3O12.
The difference in the linear CTEs measured by XRD and dilatometry could be understood by the microstructural effects. In contrast to XRD measurement which gives the thermal expansion property of cell lattice, dilatometry reveals the bulk thermal expansion property, including both intrinsic (thermal expansion of a material arising from the lattice dynamics) and extrinsic (thermal expansion related to microstructures such as texture, grain size, grain boundaries, poses, and microcracks) effects. The difference measured by the two methods reflects the extrinsic effect in the sintered bulk, which, on heating, can add a small negative component to the intrinsic linear expansion coefficients. Generally speaking, a smaller difference suggests a better sintered quality of the bulk material which is desired for most applications. The absolute differences for the Zn-containing compounds (Δ for x = 0.2 and Δ for x = 0.3) are obviously smaller than that for HfMgMo3O12 (Δ). It means that partial substitution of Mg2+by Zn2+ in HfMgMo3O12 could improve the sintering performance of the material and minimize the possible contributions of extrinsic effects. The analysis is supported by microstructural observation.
Figure 5 shows the SEM images of HfMg1−xZnxMo3O12 ceramics with x = 0.0 (Figure 5A), 0.1 (Figure 5B), 0.2 (Figure 5C), 0.3 (Figure 5D), 0.4 (Figure 5E), and 0.5 (Figure 5F). The micro morphology of the sample for x = 0.1 is dominated by well crystallized polyhdra or truncated polyhedra and the average particle size is obviously smaller than that of HfMgMo3O12. With increasing the content of Zn2+, the polyhedra become more rounded. Compared to HfMgMo3O12, the incorporation of Zn2+ seems to lead to less pores in the ceramic bodies and pores can hardly be found in the solid solutions for x = 0.1–0.3. It illustrates that proper amount incorporation of Zn2+ favors the formation of uniform distribution of particles and efficient reduction of porosity in the sintered body.
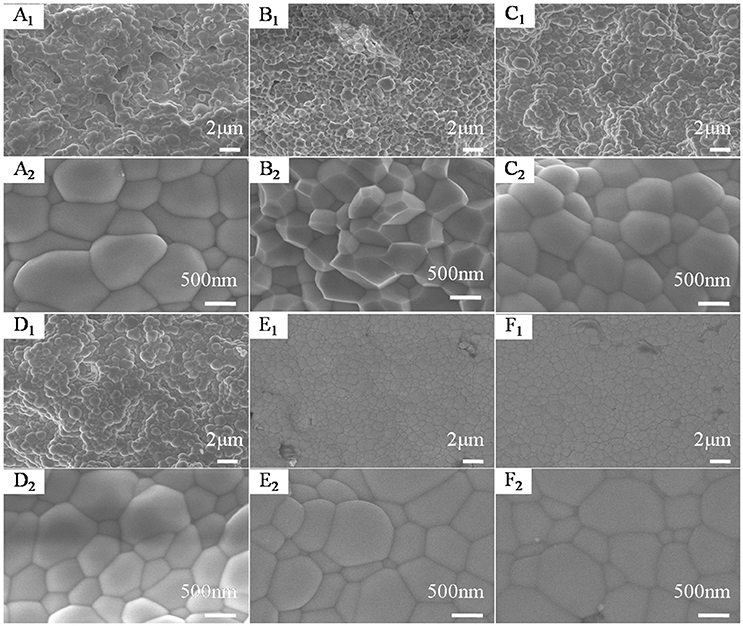
Figure 5. SEM images of HfMg1−xZnxMo3O12 with x = 0.0 (A), 0.1 (B), 0.2 (C), 0.3 (D), 0.4 (E), and 0.5 (F).
The schematic diagram of HfMgMo3O12 is given in Figure 6A to help us understand the mechanism of the phenomenons. In HfMg1−xZnxMo3O12 (x ≤ 0.5), Zn2+ is expected to substitute for Mg2+ due to the same valence and similar cation radius and each ZnO6 octahedron shares all its corners with six MoO4 tetrahedra. In order to see the bond strength changes induced by local electronic environment upon substitution of Zn2+for Mg2+, we show in Figure 6B the Raman spectra of the stretching region for x = 0.0, 0.1, 0.2, 0.3, 0.4, and 0.5. It is obvious that all the stretching modes shift successively to lower wavenumbers with increasing the content of Zn2+, indicating a softening of the Mo-O bonds upon incorporation of Zn2+. Once an Mg2+ is replaced by Zn2+, the local electronic equilibrium around the MoO4 tetrahedron is broken. Zn2+ has obviously a higher electronegativity and ability to drag electrons to the ZnO6 octahedron from its connected six MoO4 tetrahedra than Mg2+, resulting in the weakening of the Mo–O bonds. The differences in ionic radius and electronegativity could cause a slight rotation of the connected polyhedra and hence the M-O-M linkages. This is probably the reason that the positive expansion of the b-axis is pronouncedly reduced and the NTE in the c-axes become more negative, resulting hence in a lower anisotropy in thermal expansion and near-zero CTEs of the Zn-containing compounds. Due to the large difference in electronegativity between Zn2+ (1.65 Pauling) and Mg2+ (1.31 Pauling), the more of Zn2+ is incorporated, the more of the MoO4 tetrahedra get distorted as revealed by Raman spectroscopy, resulting in larger distortion and instability of the lattice. When the forming energy for the single phase exceeds that for the multi-phases, then the multi-phases form.
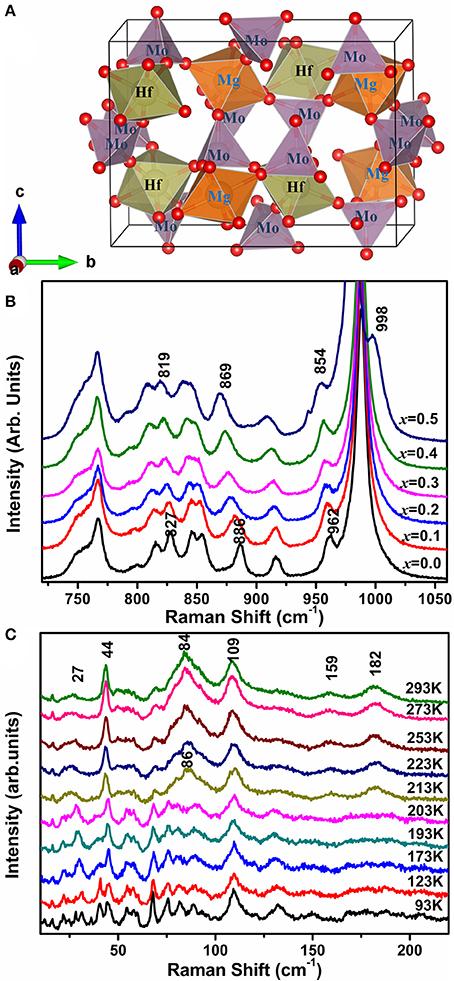
Figure 6. (A) Schematic diagram of HfMgMo3O12 building block (red sphere indicates oxygen atom); (B) Raman spectra of HfMg1−xZnxMo3O12 in the high wavenumber regions; (C) Temperature-dependent Raman spectra of HfMg0.9Zn0.1Mo3O12 in the low wavenumber region.
Figure 6C shows the temperature-dependent Raman spectra of HfMg0.9Zn0.1Mo3O12 in the low wavenumber region. Obvious change of the Raman spectra occur between 203 and 213 K, corresponding to the monoclinic to orthorhombic phase transition, such as the appearance of new Raman modes at about 27,44, 86, 159, and 182 cm–1. The modes at about 44 and 86 cm–1 are split into two or three modes in the low temperature phase and become degenerated in the high temperature phase. The low wavenumber modes arise from the external librational and translational vibrations of the connected octahedra–tetrahedra, or the librational and translational motions of metal ions in the Hf(Mg/Zn)–O–Mo linkages, which can also be regarded as the transverse vibrations of the bridging oxygen from the point of view of relative movement. Such an harmonic vibrations along with the distortion of the polyhedra are believed to be the origin of the NTE in the open frame work structure since they bring the two end atoms closer upon heating (Evans, 1999; Ding et al., 2008; Marinkovic et al., 2009; Wang et al., 2013).
Conclusion
Solid solutions of HfMg1−xZnxMo3O12 with near-ZTE are successfully synthesized by solid state reaction and the effects of Zn2+ incorporation on the phase formation, thermal expansion, phase transition, and vibrational properties and micro-morphologies are investigated by XRD, dilatometry, Raman spectroscopy, and SEM. It is shown that (i) single phase formation is only possible for x ≤ 0.5, otherwise, additional phases of HfMo2O8 and ZnMoO4 generate; (ii) HfMg1−xZnxMo3O12 crystallize in an orthorhombic structure for x ≤ 0.4 and in a monoclinic structure for x = 0.5 at RT; (iii) The phase transition temperature from monoclinic to orthorhombic structure increases with the content of Zn2+, which occurs within 168–178, 203–213, 223–233, 258–268, 283–293, and 318–328 K for x = 0.0, 0.1, 0.2, 0.3, 0.4, and 0.5, respectively; (iv) The incorporation of Zn2+ leads to an pronounced reduction in the positive expansion of the b-axis and an enhanced NTE in c-axes, making the Zn-containing materials exhibit near-ZTE over a wide temperature range and lower anisotropy in thermal expansion in the orthorhombic phase; (v) Replacement of Mg2+ by Zn2+ breaks the local electronic equilibrium around the MoO4 tetrahedron and weakens the Mo–O bonds, leading to obvious red shifts of all the Mo–O stretching modes with increasing the content of Zn2+ due to obviously higher electronegativity of Zn2+ than Mg2+; (vi) The incorporation of Zn2+ improves sintering property of samples, minimizing the possible contributions of extrinsic effects such as pores, which is preferred for most applications.
Author Contributions
EL conceived the idea and supervised the research. SL and RS are in charge of the synthesis and part measurements of the materials. XG, HY, and DC are in charge of the thermal expansion and Raman characterization. JG and MC are in charge of the XRD characterization and structural analyses. SL, XG, and EL are in charge of the manuscript preparation.
Conflict of Interest Statement
The authors declare that the research was conducted in the absence of any commercial or financial relationships that could be construed as a potential conflict of interest.
Acknowledgments
This work was supported by the National Natural Science Foundation of China (No. 11574276; No. 51302249).
References
Ahsaine, H. A., Zbair, M., Ezahri, M., Benlhachemi, A., Bakiz, B., Guinneton, F., et al. (2016). Structural and temperature-dependent vibrational analyses of the non-centrosymmetric ZnMoO4 molybdate. J. Mater. Environ. Sci. 7, 907–915.
Allen, S., Ward, R. J., Hampson, M. R., Gover, R. K., and Evans, J. S. (2004). Structures and phase transitions of trigonal ZrMo2O8 and HfMo2O8. Acta Crystallogr. B. 60, 32–40. doi: 10.1107/S0108768103025138
Azuma, M., Chen, W., Seki, H., Czapski, M., Olga, S., Oka, K., et al. (2011). Colossal negative thermal expansion in BiNiO3 induced by intermetallic charge transfer. Nat. Commun. 2:347. doi: 10.1038/ncomms1361
Baiz, T. I., Gindhart, A. M., Kraemer, S. K., and Lind, C. (2008). Synthesis of MgHf(WO4)3and MgZr(WO4)3 using a non-hydrolytic sol-gel method. J. Solgel Sci. Technol. 47, 128–130. doi: 10.1007/s10971-008-1765-5
Bridges, F., Keiber, T., Juhas, P., Billinge, S. J. L., Sutton, L., Wilde, J., et al. (2014). Local vibrations and negative thermal expansion in ZrW2O8. Phys. Rev. Lett. 112:045505. doi: 10.1103/PhysRevLett.112.045505
Chen, D. X., Yuan, B. H., Cheng, Y. G., Ge, X. H., Jia, Y., Liang, E. J., et al. (2016). Phase transition and near-zero thermal expansion in ZrFeMo2VO12. Phys. Lett. A 380, 4070–4074. doi: 10.1016/j.physleta.2016.10.009
Chen, J., Hu, L., Deng, J., and Xing, X. (2015). Negative thermal expansion in functional materials: controllable thermal expansion by chemical modifications. Chem. Soc. Rev. 44, 3522–3567. doi: 10.1039/C4CS00461B
Chen, J., Wang, F. F., Huang, Q. Z., Hu, L., Song, X. P., Deng, J. X., et al. (2013). Effectively control negative thermal expansion of single-phase ferroelectrics of PbTiO3-(Bi,La)FeO3 over a giant range. Sci. Rep. 3:2458. doi: 10.1038/srep02458
Cheng, Y. G., Liang, Y., Ge, X. H., Liu, X. S., Yuan, B. H., Guo, J., et al. (2016a). A novel material of HfScMo2VO12 with negative thermal expansion and intense white-light emission. RSC Adv. 6, 53657–53661. doi: 10.1039/C6RA09666B
Cheng, Y. G., Liang, Y., Mao, Y. C., Ge, X. H., Yuan, B. H., Guo, J., et al. (2017). A novel material of HfScW2PO12 with negative thermal expansion from 140 K to 1469 K and intense blue photoluminescence. Mater. Res. Bull. 85, 176–180. doi: 10.1016/j.materresbull.2016.09.008
Cheng, Y. G., Mao, Y. C., Liu, X. S., Yuan, B. H., Chao, M. J., and Liang, E. J. (2016b). Near-zero thermal expansion of In2(1−x)(HfMg)xMo3O12 with tailored phase transition. Chin. Phys. B 25:86501. doi: 10.1088/1674-1056/25/8/086501
Das, S., Das, S., and Das, K. (2013). Low temperature synthesis of negative thermal expansion Y2W3O12. J. Mater. Eng. Perf. 22, 3357–3363. doi: 10.1007/s11665-013-0652-6
Ding, P., Liang, E. J., Jia, Y., and Du, Z. Y. (2008). Electronic structure, bonding and phonon modes in the negative thermal expansion materials of Cd(CN)2 and Zn(CN)2. J. Phys. Condens. Matter 20:275224. doi: 10.1088/0953-8984/20/27/275224
Evans, J. S. O. (1999). Negative thermal expansion materials. J. Chem. Soc. Dalton Trans. 1999, 3317–3326. doi: 10.1039/a904297k
Evans, J. S. O., Hu, Z., Jorgensen, J. D., Argyriou, D. N., Short, S., and Sleight, A. W. (1997a). Compressibility, phase transitions, and oxygen migration in zirconium tungstate, ZrW2O8. Science 275, 61–65. doi: 10.1126/science.275.5296.61
Evans, J. S. O., Mary, T. A., and Sleight, A. W. (1997b). Negative thermal expansion in a large molybdate and tungstate family. J. Solid State Chem. 133, 580–583. doi: 10.1006/jssc.1997.7605
Evans, J. S. O., Mary, T. A., Vogt, T., Subramanian, M. A., and Sleight, A. W. (1996). Negative thermal expansion in ZrW2O8 and HfW2O8. Chem. Mater. 8, 2809–2823. doi: 10.1021/cm9602959
Ge, X., Liu, X., Cheng, Y., Yuan, B., Chen, D., Chao, M., et al. (2016c). Negative thermal expansion and photoluminescence properties in a novel material ZrScW2PO12. J. Appl. Phys. 120:205101. doi: 10.1063/1.4968546
Ge, X. H., Mao, Y. C., Li, L., Li, L. P., Yuan, N., Cheng, Y. G., et al. (2016a). Phase transition and negative thermal expansion property of ZrMnMo3O12. Chin. Phys. Lett. 33:046503. doi: 10.1088/0256-307X/33/4/046503
Ge, X. H., Mao, Y. C., Liu, X. S., Cheng, Y. G., Yuan, B. H., Chao, M. J., et al. (2016b). Negative thermal expansion and broad band photoluminescence in a novel material of ZrScMo2VO12. Sci. Rep. 6:24832. doi: 10.1038/srep24832
Gindhart, A. M., Lind, C., and Green, M. (2008). Polymorphism in the negative thermal expansion material magnesium hafnium tungstate. J. Mater. Res. 23, 210–213. doi: 10.1557/JMR.2008.0013
Hu, L., Chen, J., Fan, L. L., Ren, Y., Rong, Y. C., Pan, Z., et al. (2015). ChemInform abstract: zero thermal expansion and ferromagnetism in cubic Sc1−xMxF3 (M: Ga, Fe) over a wide temperature range. Cheminform 46:13566. doi: 10.1002/chin.201507003
Lama, P., Das, R. K., Smith, V. J., and Barbour, L. J. (2014). A combined stretching-tilting mechanism produces negative, zero and positive linear thermal expansion in a semi-flexible Cd(II)-MOF. Chem. Commun. 50, 6464–6467. doi: 10.1039/C4CC02634A
Li, F., Liu, X. S., Song, W. B., Yuan, B. H., Cheng, Y. G., Yuan, H. L., et al. (2014). Phase transition, crystal water and low thermal expansion behavior of Al2–2x(ZrMg)xW3O12· n(H2O). J. Solid State Chem. 218, 15–22. doi: 10.1016/j.jssc.2014.06.009
Li, T., Ge, X. H., Liu, X. S., Cheng, Y. G., Liu, Y. Y., Yuan, H. L., et al. (2016). Enhanced negative thermal expansion by solid solution of HfMgMo1.5W1.5O12. Mater. Expr. 6, 515–520. doi: 10.1166/mex.2016.1337
Li, T., Liu, X. S., Cheng, Y. G., Ge, X. H., Zhang, M. D., Lian, H., et al. (2017). Zero and controllable thermal expansion in HfMgMo3−xWxO12. Chin. Phys. B 26:348. doi: 10.1088/1674-1056/26/1/016501
Li, Z. Y., Song, W. B., and Liang, E. J. (2011). Structures, phase transition, and crystal water of Fe2−xYxMo3O12. J. Phys. Chem. C 115, 17806–17811. doi: 10.1021/jp201962b
Liang, E. J., Huo, H. L., Wang, J. P., and Chao, M. J. (2008a). Effect of water species on the phonon modes in orthorhombic Y2(MoO4)3 revealed by Raman spectroscopy. J. Phys. Chem. C 112, 6577–6581. doi: 10.1021/jp8013332
Liang, E. J., Liang, Y., Zhao, Y., Liu, J., and Jiang, Y. (2008b). Low-frequency phonon modes and negative thermal expansion in A(MO4)2 (A = Zr, Hf and M = W, Mo) by Raman and Terahertz time-domain spectroscopy. J. Phys. Chem. A 112, 12582–12587. doi: 10.1021/jp805256d
Liu, X. S., Cheng, Y. G., Liang, E. J., and Chao, M. J. (2014). Interaction of crystal water with the building block in Y2Mo3O12 and the effect of Ce3+ doping. Phys. Chem. Chem. Phys. 16, 12848–12857. doi: 10.1039/c4cp00144c
Liu, X. S., Wang, J. Q., Fan, C. Z., Shang, R., Cheng, F. X., Yuan, B. H., et al. (2015). Control of reaction pathways for rapid synthesis of negative thermal expansion ceramic Zr2P2WO12 with uniform microstructure. Int. J. Appl. Ceram. Technol. 12, E28–E33. doi: 10.1111/ijac.12201
Liu, Y. Y., Yuan, B. H., Cheng, Y. G., Liang, E. J., Ge, X. H., Yuan, H. L., et al. (2018). Phase transition and negative thermal expansion of HfMnMo3O12. Mater. Res. Bull. 99, 255–259. doi: 10.1016/j.materresbull.2017.11.009
Long, Y. W., Hayashi, N., Saito, T., Azuma, M., Muranaka, S., and Shimakawa, Y. (2009). Temperature-induced A-B intersite charge transfer in an A-site-ordered LaCu3Fe4O12 perovskite. Cheminform 40, 60–63. doi: 10.1002/chin.200947022
Marinkovic, B. A., Ari, M., Avillez, R. R. D., Rizzo, F., Ferreira, F. F., Miller, K. J., et al. (2009). Correlation between AO6 polyhedral distortion and negative thermal expansion in orthorhombic Y2Mo3O12 and related materials. Chem. Mater. 21, 2886–2894. doi: 10.1021/cm900650c
Marinkovic, B. A., Jardim, P. M., Ari, M., Avillez, R. R. D., Rizzo, F., and Ferreira, F. F. (2008). Low positive thermal expansion in HfMgMo3O12. Phys. Status Solidi 245, 2514–2519. doi: 10.1002/pssb.200880262
Miller, K. J., Johnson, M. B., White, M. A., and Marinkovic, B. A. (2012). Low-temperature investigations of the open-framework material HfMgMo3O12. Solid State Commun. 152, 1748–1752. doi: 10.1016/j.ssc.2012.06.022
Miller, K. J., Romao, C. P., Bieringer, M., Marinkovic, B. A., Prisco, L., and White, M. A. (2013). Near-zero thermal expansion in In(HfMg)0.5Mo3O12. J. Am. Ceram. Soc. 96, 561–566. doi: 10.1111/jace.12085
Omote, A., Yotsuhashi, S., Zenitani, Y., and Yamada, Y. (2011). High ion conductivity in MgHf(WO4)3 solids with ordered structure: 1-D alignments of Mg2+and Hf 4+ ions. J. Am. Ceram. Soc. 94, 2285–2288. doi: 10.1111/j.1551-2916.2011.04644.x
Peng, X., Chen, J., Lin, K., Fan, L. L., Rong, Y. C., Deng, J. X., et al. (2016). Structure and control of negative thermal expansion of Nd/Sm substituted 0.5PbTiO3-0.5BiFeO3 ferroelectrics. RSC Adv. 6, 32979–32982. doi: 10.1039/C6RA03774G
Peng, Z., Sun, Y. Z., and Peng, L. M. (2014). Hydrothermal synthesis of ZrW2O8 nanorods and its application in ZrW2O8/Cu composites with controllable thermal expansion coefficients. Mater. Des. 54, 989–994. doi: 10.1016/j.matdes.2013.09.012
Pryde, A. K. A., Hammonds, K. D., Dove, M. T., Heine, V., Gale, J. D., and Warren, M. C. (1996). Origin of the negative thermal expansion in ZrW2O8 and ZrV2O7. J. Phys. Condens. Matter 8:10973. doi: 10.1088/0953-8984/8/50/023
Qu, B. Y., He, H. Y., and Pan, B. C. (2012).Origin of the giant negative thermal expansion in Mn3(Cu0.5Ge0.5)N. Adv. Conden. Matter Phys. 2012, 275–281. doi: 10.1155/2012/913168
Reichelt, W., Weber, T., Söhnel, T., and Däbritz, S. (2000). Mischkristallbildung im system CuMoO4/ZnMoO4. Z. Anorgan. Allgem. Chem. 626, 2020–2027. doi: 10.1002/1521-3749(200009)626:9<2020::AID-ZAAC2020>3.0.CO;2-K
Song, W. B., Liang, E. J., Liu, X. S., Li, Z. Y., Yuan, B. H., and Wang, J. Q. (2013). A negative thermal expansion material of ZrMgMo3O12. Chin. Phys. Lett. 30:126502. doi: 10.1088/0256-307X/30/12/126502
Song, W. B., Wang, J. Q., Li, Z. Y., Liu, X. S., Yuan, B. H., and Liang, E. J. (2014a). Phase transition and thermal expansion property of Cr2−xZr0.5xMg0.5xMo3O12 solid solution. Chin. Phys. B 23:433. doi: 10.1088/1674-1056/23/6/066501
Song, W. B., Yuan, B. H., Liu, X. S., Li, Z. Y., Wang, J. Q., and Liang, E. J. (2014b). Tuning the monoclinic-to-orthorhombic phase transition temperature of Fe2Mo3O12 by substitutional co-incorporation of Zr4+ and Mg2+. J. Mater. Res. 29, 849–855. doi: 10.1557/jmr.2014.63
Srikanth, V., Subbarao, E. C., and Rao, G. V. (1992). Thermal expansion anisotropy, microcracking and acoustic emission of Nb2O5 ceramics. Ceram. Int. 18, 251–261. doi: 10.1016/0272-8842(92)90103-K
Suzuki, T., and Omote, A. (2004). Negative thermal expansion in (HfMg)(WO4)3. J. Am. Ceram. Soc. 87, 1365–1367. doi: 10.1111/j.1151-2916.2004.tb07737.x
Suzuki, T., and Omote, A. (2006). Zero thermal expansion in (Al2x(HfMg)1−x)(WO4)3. J. Am. Ceram. Soc. 89, 691–693. doi: 10.1111/j.1551-2916.2005.00729.x
Takenaka, K., and Takagi, H. (2005). Giant negative thermal expansion in Ge-doped anti-perovskite manganese nitrides. Appl. Phys. Lett. 87:261902. doi: 10.1063/1.2147726
Tallentire, S. E., Child, F., Fall, I., Vella-Zarb, L., Evans, I. R., Tucker, M. G., et al. (2013). Systematic and controllable negative, zero, and positive thermal expansion in cubic Zr1−xSnxMo2O8. J. Am. Chem. Soc. 135, 12849–12856. doi: 10.1021/ja4060564
Wang, L., Wang, F., Yuan, P. F., Sun, Q., Liang, E. J., Jia, Y., et al. (2013). Negative thermal expansion correlated with polyhedral movements and distortions in orthorhombic Y2Mo3O12. Mater. Res. Bull. 48, 2724–2729. doi: 10.1016/j.materresbull.2013.04.001
Wang, X., Huang, Q., Deng, J., Yu, R., Chen, J., and Xing, X. (2011). Phase transformation and negative thermal expansion in TaVO5. Inorg. Chem. 50, 2685–2690. doi: 10.1021/ic200003n
Wu, M., Liu, X., Chen, D., Huang, Q., Wu, H., and Liu, Y. (2014). Structure, phase transition, and controllable thermal expansion behaviors of Sc2−xFexMo3O12. Inorg. Chem. 53, 9206–9212. doi: 10.1021/ic501271t
Wu, M. M., Peng, J., Han, S. B., Hu, Z. B., Liu, Y. T., and Chen, D. F. (2012). Phase transition and negative thermal expansion properties of Sc2−xCrxMo3O12. Ceram. Int. 38, 6525–6529. doi: 10.1016/j.ceramint.2012.05.033
Wu, M. M., Xiao, X. L., Hu, Z. B., Liu, Y. T., and Chen, D. F. (2009). Controllable thermal expansion and phase transition in Yb2−xCrxMo3O12. Solid State Sci. 11, 325–329. doi: 10.1016/j.solidstatesciences.2008.08.002
Xiao, X., Zhou, W. J., Liu, X. S., Chao, M. J., Li, Y. C., Zhang, N., et al. (2014). Electrical properties of Al-ZrMgMo3O12 with controllable thermal expansion. Ceram. Int. 41, 2361–2366. doi: 10.1016/j.ceramint.2014.10.048
Yamada, I., Shiro, K., Etani, H., Marukawa, S., Hayashi, N., Mizumaki, M., et al. (2016). Valence transitions in negative thermal expansion material SrCu3Fe4O12. Inorg. Chem. 53, 10563–10569. doi: 10.1021/ic501665c
Yan, J., Sun, Y., Wang, C., Chu, L. H., Shi, Z. X., Deng, S. H., et al. (2014). Study of structure of Mn3Cu0.5Ge0.5N/Cu composite with nearly zero thermal expansion behavior around room temperature. Scr. Mater. 84–85, 19–22. doi: 10.1016/j.scriptamat.2014.04.010
Keywords: thermal expansion, near-zero thermal expansion, phase transition, X-ray diffraction (XRD), Raman spectrum
Citation: Li S, Ge X, Yuan H, Chen D, Guo J, Shen R, Chao M and Liang E (2018) Near-Zero Thermal Expansion and Phase Transitions in HfMg1−xZnxMo3O12. Front. Chem. 6:115. doi: 10.3389/fchem.2018.00115
Received: 15 January 2018; Accepted: 29 March 2018;
Published: 17 April 2018.
Edited by:
Jun Chen, University of Science and Technology Beijing, ChinaCopyright © 2018 Li, Ge, Yuan, Chen, Guo, Shen, Chao and Liang. This is an open-access article distributed under the terms of the Creative Commons Attribution License (CC BY). The use, distribution or reproduction in other forums is permitted, provided the original author(s) and the copyright owner are credited and that the original publication in this journal is cited, in accordance with accepted academic practice. No use, distribution or reproduction is permitted which does not comply with these terms.
*Correspondence: Erjun Liang, ZWpsaWFuZ0B6enUuZWR1LmNu