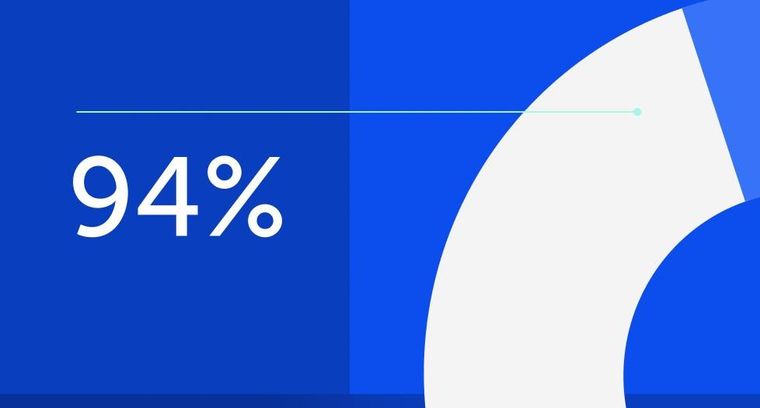
94% of researchers rate our articles as excellent or good
Learn more about the work of our research integrity team to safeguard the quality of each article we publish.
Find out more
ORIGINAL RESEARCH article
Front. Chem. Eng., 11 April 2025
Sec. Environmental Chemical Engineering
Volume 7 - 2025 | https://doi.org/10.3389/fceng.2025.1515470
This article is part of the Research TopicHydrothermal Liquefaction: Aqueous Phase Treatment, Product Recovery, and Downstream ImplicationsView all 3 articles
Hydrothermal liquefaction (HTL) has the potential to improve resource recovery at water resource recovery facilities (WRRF), but the production of a high-strength aqueous by-product (HTL-aq) is hampering HTL implementation. The formation of biofilms in anaerobic digestion have been shown to be useful when degrading recalcitrant compounds present in HTL-aq due to the promotion of direct interspecies electron transfer (DIET) and increase in the microbial activity of syntrophic and methanogenic populations. The Recirculating Anaerobic Dynamic Membrane Bioreactor (RAnDMBr) was able to degrade 65% of the chemical oxygen demand (COD) at 1.5 ± 0.2 g COD LR−1 day−1 and 5.6 ± 2.3 days producing 0.19 ± 0.02 LCH4 gCODfed−1. However, adding a solution rich in nutrients on a daily basis was necessary. The system presented microbial populations able to degrade aromatic compounds (i.e., Anaerolinaceae) to perform DIET and syntrophy (i.e., Syntrophus) and methanogens (i.e., Methanobacterium and Methanosarcina) with the biofilm having a higher relative abundance of methanogens than the suspended biomass. Increasing the organic loading rate to 2 g COD LR−1 day−1 caused inhibition in the system by accumulation of volatile fatty acids, probably due to an increase in phenol, N-heterocyclic and aromatic compounds. Overall, this research shows that the RAnDMBr can be used to treat HTL-aq in WRRF without inhibition at OLRs of 1.5 ± 0.2 g COD LR−1 day−1 or lower, making HTL-aq treatment more feasible. Future research should focus co-digestion of HTL-aq with a co-substrate rich in nutrients and on fouling mitigation strategies that will allow to increase the recirculation ratio to promote advective substrate transport through the biofilm.
While the energy, nutrients and metals contained in wastewater are valued in $600 USD/Mgal, the current treatment approach in water resource recovery facilities (WRRF) is inefficient in terms of energy and materials recovery (Rittmann, 2011; Mulchandani and Westerhoff, 2016). Sludge management can be seen as an opportunity to recover the chemical energy from wastewater. Of all the sludge produced in the US, 22% is being landfilled, 16% is being incinerated and 52% is being land applied (with 80% of the sludge being digested before land application) (Urgun-Demirtas et al., 2022). Anaerobic digestion (AD) followed by land application can recover electricity and heat from biogas and introduce nutrients in agriculture. However, only 5%–7% of the US WRRF have digesters and use the biogas (Shen et al., 2015). Besides, 50% of the sludge volume still remains after digestion and needs to be processed through land application, landfilling or incineration. This results in 6.5 Mt/y of treated biosolids, which are land applied, incinerated or sent to a landfill costing $330-$880 USD/t (Seiple et al., 2020). Moreover, new regulations and concerns about emerging contaminants such as Per- and polyfluoroalkyl substances in wastewater solids are creating challenges and new costs for land application that almost guarantee that solids management will continue to increase in cost (Winchell et al., 2024). Thus, there is a critical need to implement new processes to maximize resource recovery and remove emerging contaminants without increasing overall costs.
Hydrothermal liquefaction (HTL) uses moderately hot water (at 280°C–370°C) and high pressure (1400–3600 PSI) conditions to convert wet-wastes sewage sludge to biocrude in 30 min or less. HTL is a promising technology for WRRFs because it can reduce sludge volume by 90%, recover P in the form of a solid fertilizer and convert 70% of the energy content in the sludge into biocrude, a precursor for aviation fuel (Basar et al., 2021; Usman et al., 2023). The results of a U.S. based analysis suggest an estimated minimum fuel selling price for biocrude from $2.28/gasoline gallon equivalent (GGE) to $3.45/GGE, and an estimated supply chain greenhouse gas emissions reduction from 73.4% to 81.8% relative to petroleum-derived diesel (Jiang et al., 2023). For WRRFs, where solids handling costs are rapidly increasing due to various factors (fuel cost for incineration, restrictions on land and landfill disposal), the integration of HTL could decrease disposal costs by 43% (Seiple et al., 2020). An HTL-based system could also offer a value proposition by destroying emerging contaminants (Hao et al., 2021). Nonetheless, HTL has not yet been implemented at the full scale. One of the reasons HTL has not been implemented is because it generates an aqueous by-product (HTL-aq) with a high concentration of chemical oxygen demand (COD) (50–70 g/L) and NH4+ (5–8 g N/L) with phenol, N-heterocyclic and aromatic compounds that makes HTL-aq unsuitable for direct recirculation to the WRRF headworks (Basar et al., 2023; Zhu et al., 2023). While multiple options have been proposed to treat HTl-aq (e.g., microbial fuel cell, microbial electrolysis, membrane distillation, super critical water oxidation, catalytic hydrothermal gasification), the processes are not ready to be implemented in WRRF at the full scale (Watson et al., 2020). The use of AD to treat HTL-aq can be practical as it presents low operating costs compared to other technologies, it recovers resources like biogas, and its operation is well-known. As the AD technology is already treating 40% of the sludge produced in the U.S. (Shen et al., 2015), the anaerobic digesters can be utilized for HTL-aq treatment if HTL is implemented at WRRFs. However, the treatment of HTL-aq through conventional AD can be challenging due to the presence of phenol, N-heterocyclic and aromatic compounds in HTL-aq, that are hard to biodegrade under anaerobic conditions (Padoley et al., 2008; Poirier et al., 2016; Kong et al., 2019; Azarmina and Eskicioglu, 2025). For example, Azarmina and Eskicioglu, (2025) used a conventional CSTR to treat HTL-aq but the system could only degrade HTL-aq without inhibition if HTL-aq was diluted 25 times or higher. Therefore other AD configurations new approaches are necessary to ensure proper treatment. Batch treatment is the most studied AD configuration for HTL-aq treatment (Wang et al., 2021; Adedeji et al., 2023; Zhu et al., 2023; Liu et al., 2024). However, batch configurations are not a good representation of the reality because most AD plants are continuous. Studies using continuous AD systems to treat HTL-aq are still scarce but overall, configurations decoupling the SRT from HRT have been used (Chen et al., 2016; Si et al., 2018; Usman et al., 2019; Hao et al., 2020; Yang et al., 2020; Bueno et al., 2021). For example, Bueno et al. (2021) used a Horizontal-Flow Anaerobic Immobilized Biomass reactor and started to experience inhibition when the OLR increased from 1.6 gCOD LR−1 day-1 to 3.2 gCOD LR−1 day−1. Si et al. (2018) used two different configurations, a UASB and a PBR to treat HTL-aq at an OLR of eight gCOD LR−1 day−1, but the test only lasted 8 days. Overall, the formation of biofilms in AD have been shown to be useful when degrading recalcitrant compounds present in HTL-aq due to the promotion of direct interspecies electron transfer (DIET) and increase in the microbial activity of syntrophic and methanogenic populations (Usman et al., 2019; Zhu et al., 2023). Furthermore, biofilms can provide protection to microorganisms again toxic compounds (Mah and O’toole, 2001; Cohen, 2011). Anaerobic membrane bioreactors are an AD configuration that has never been tested for HTL-aq treatment. Specially, using of the recent developed Recirculating Anaerobic Dynamic Membrane Bioreactor (RAnDMBr) to treat HTL-aq could be very relevant (Fairley-wax et al., 2022). The RAnDMBR operation enhances biofilm formation, something was shown to be critical to protect the microorganisms from the HTL-aq toxic compounds.
Here, a WRRF in the US [Great Lakes Water Authority (GLWA)] and a national lab [Pacific Northwest National Lab (PNNL)] created a multidisciplinary partnership to use a new AD configuration, the RAnDMBR, to treat HTL-aq (Fairley-wax et al., 2022). The RAnDMBR was used to promote the formation of an enhanced biofilm, enhance syntrophy between microbial populations and increase the microbial activity. The goal of this study is to reduce the COD load of HTL-aq without applying an excessive dilution factor and keeping a hydraulic retention time between 5-10 days.
The RAnDMBr was inoculated with the effluent coming from a pilot scale digester treating sewage sludge and food waste at GLWA WRRF (Detroit, MI, United States). After collecting the inoculum, it was stored a 4°C for 17 days before its use. A mixture of primary and secondary sludge (7:1; wet weight) with a 75% moisture content was collected at GLWA WRRF and transported with a refrigerated truck to PNNL. At PNNL the sludge was processed through an engineering-scale HTL system, referred to as MHTLS, at 12 L/h, 350°C, 2900 psig and at a residence time of 15 min in the plug flow tubular reactor (Marrone et al., 2018; Snowden-Swan et al., 2021; Cronin et al., 2022). Just downstream of the reactor, the product slurry passes into a vessel that captures solids (mostly ash) though settling and filtration. The solids are intermittently removed from the process with a blowdown system. With the fine non-volatile solid removed, pressure letdown is simplified and the aqueous and biocrude streams are gravity segregated in a conventional oil/water separator (Supplementary Figures S1A, B). About 200 L of the separated HTL-aq was analyzed at PNNL through High-performance liquid chromatography and Ion chromatography and sent back to GLWA to perform the testing with the RAnDMBR. During the testing, the HTL-aq was stored in the fridge at 4°C. A characterization of the inoculum, the HTL-aq used in this study can be seen in Supplementary Tables S1, S2. HTL performance and detailed characterization data on the feed, products and byproduct streams from the HTL test, (Test MHTLS-15), other product and byproduct of the HTL test are also included in the study performed by Snowden-Swan et al. (2022).
A 5.0 L (working volume including membrane) semi-continuous RAnDMBR similar the one developed by Fairley-wax et al. (2022) was used in this study for the treatment of HTL-aq. A schematic of the RAnDMBR can be seen in Supplementary Figure S1C. The RAnDMBR temperature was controlled at 37°C through the use of a recirculating water bath (VEVOR, United States) and mixing was provided by a magnetic stirrer (Corning, United States). Hamilton probes EasyFerm Plus PHI Arc 325 and EasyFerm Plus oxidation-reduction potential Arc 325 were used to continuously monitor temperature, pH and oxidation-reduction potential. The RAnDMBR was set up with two peristaltic pumps (Masterflex 07551-20), a three-way valve (REDHAT 120V AC, Grainger, United States) and a pressure transducer (OMEGA, United States) to measure transmembrane pressure (TMP). The design of the RAnDMBr involves a tree-like structure submerged in the bioreactor which branches were surrounded by a stainless-steel mesh of 25 μm pore size (Supplementary Figure S1C). The goal of the tree-like structure is to build a high surface area biofilm to enhance the degradation of HTL-aq. The branches in the tree-like structure provided a very high surface area (19.79 m2 mreactor−3) in the system where the biofilm (dynamic membrane) was developed. Contrary to common dynamic membrane bioreactors, the reactor bulk liquid was not permeated continuously through the dynamic membrane. In order to generate a biofilm on the branches of the tree-like structure and promote advective substrate transport, the bulk liquid was recirculated through the meshes. Therefore, the RAnDMBR would work under cycles of recirculation and permeation (Supplementary Figure S1D). For a period of time of tR, the system would recirculate the bulk liquid through the stainless steel meshes and for a period of time tP the system would perform permeation. Permeation would take place by activating a three-way valve that would switch the direction of the fluid from recirculation to permeation (Supplementary Figure S1D). Because the dynamic membrane permeability would not be constant, the time of recirculation and permeation varied during the study to keep the reactor at the desired hydraulic retention time (HRT) (5 or 10 days depending on the experiment stage, see Table 1). To determine how often the bulk liquid was recirculated through the dynamic membrane, the recirculation ratio was calculated by dividing the recirculation flow rate by the effluent flow rate. The RAnDMBR operated under different organic loading rates by diluting the HTL-aq with DI water. The operating conditions of this study can be seen in Table 1. The changes in OLR did not exceed 0.5 g COD LR−1 day−1 to avoid inhibition of the microbial community by a very high OLR increase (Zhen et al., 2022). While replicates have not been tested during this experiment, previous studies have demonstrated that long-term time-series experiments can provide results similar to those obtained with anaerobic digesters operated in replicate (Vanwonterghem et al., 2014; Werner et al., 2014).
The inoculum was characterized at the time of inoculation (day 0) and the feedstock samples were analyzed once a week. Suspended biomass (bulk liquid) and permeate samples were collected three times a week. Total and volatile suspended solids (TSS and VSS) were analyzed following standard methods (APHA et al., 2012). Total, partial, and intermediate alkalinity were determined using an automatic titrator with hydrochloric acid (Metler Toledo, United States) (Ripley et al., 1986). Concentrations of volatile fatty acids (VFAs) from C2 to C7, including iso-forms of C4 and C5, were determined using an Agilent Technologies 6890N GC-MS equipped with a Restek stabilwax-DA Column (Restek 11,023) (Supplementary Figure S2). The oven temperature was held at 80°C for 4 min, then increased to 250°C at 15°C min−1 and held at 250°C for 2 min. The injector temperature was set to 200°C and helium was used as the carrier gas with a flow of 1.5 mL/min. Before performing VFA analysis, all samples were centrifuged at 10,000 g for 10 min, and the supernatant was filtered through 0.2 μm nylon membrane filters (TISCH Scientific, North Bend, OH, United States). 500 μL of the filtrate was mixed with 490 μL of a 0.2% formic acid solution (Prepared by dilution of 50 μL of Formic acid into 25 mL of Acetonitrile) and 10 uL of internal standard. The internal standard was prepared by weighing 10 mg of d7-isobutyric acid (95.15 g/mol) into a 2 mL vial and diluted with 990 μL of LCMS grade water. 100 μL of this stock solution was diluted into 900 μL of LCMS grade water as the final standard solution at 1,000 μg/mL which is roughly 10 mM. Total and soluble COD (tCOD and sCOD) analyses were performed using Hach medium-range (0–1500 mg L−1) COD digestion vials. Before performing sCOD analyses, all samples were centrifuged at 10,000 × g for 10 min and the supernatant was filtered through 0.45-μm nylon membrane filters (TISCH Scientific, North Bend, OH, United States). Total phosphorus and total nitrogen were determined using Hach vials TNT 844 and TNT828, respectively, and following manufacturer instructions. Total ammonia nitrogen was measured by using an IntelliCAL ISENH3181 Ammonia Ion Selective Electrode from Hach and following manufacturer instructions, however, to avoid interferences with solids, bulk liquid samples were centrifuged at 10,000 × g for 10 min and only the supernatant was used for measurement. Biogas was collected in a Tedlar bag and was measured daily by using a 100 mL gas-tight glass syringe. The gas composition (CH4 and CO2) was measured three times a week using a biogas analyser (SWR 100, MRU). HPLC and Ion chromatography were performed by PNNL on the HTL-aq generated. HPLC analyses of samples were carried out using HPLC equipped with a Waters 2414 refractive index detector. A Bio-Rad Aminex HPX-87H ion exclusion column (300 mm × 7.8 mm) was used for analyte separation. Sulfuric acid (0.005 M) was used as eluent at a flow rate of 0.55 mL/min. Ion chromatography for anions (fluoride, bromide, nitrate, chloride, sulfate, and phosphate) was performed with a DIONEX ICS-3000 equipped with an AS11HC (4.0 × 250 mm) column and a conductivity detector. A gradient from 5.0 to 60 mM hydroxide at 1 mL/min is used to separate organic and inorganic anions. The column is held at 30°C. HTL aqueous product samples were filtered (as needed) and run neat or at 10X, or 100X, depending on analyte concentration. Detection limits range from 1.0 to 100 ppm. For each analytical batch, the system is calibrated with standard analyte mix and calibration is verified with an independent QC check.
The microbial community in the inoculum, suspended biomass (bulk liquid) and biofilm was determined though metagenomics. When collecting biofilm samples, the reactor was opened and the tree structure was removed to collect a biofilm sample from the meshes. All the biomass samples were pelletized by centrifugation at 10,000 × g for 10 min at 4°C immediately after collection. After centrifugation, the supernatant was decanted, and 1 mL of DNA shield was added before storing the samples at −20°C. The samples were shipped with dry ice to Zymo for DNA extraction and sequencing. The ZymoBIOMICS® -96 MagBead DNA Kit (Zymo Research, Irvine, CA) was used to extract DNA using an automated platform. Genomic DNA samples were profiled with shotgun metagenomic sequencing. Sequencing libraries were prepared with the KAPA™ HyperPlus Library Preparation Kit (Kapa Biosystems, Wilmington, MA) with up to 100 ng DNA input following the manufacturer’s protocol using internal single-index 8 bp barcodes with TruSeq® adapters (Illumina, San Diego, CA). All libraries were quantified with TapeStation® (Agilent Technologies, Santa Clara, CA) and then pooled in equal abundance. The final pool was quantified using qPCR. The final library was sequenced on the Illumina NovaSeq®. Raw sequence reads were trimmed to remove low quality fractions and adapters with Trimmomatic-0.33 (Bolger et al., 2014): quality trimming by sliding window with 6 bp window size and a quality cutoff of 20, and reads with size lower than 70 bp were removed. Microbial composition was profiled using sourmash (Titus Brown and Irber, 2016). The full GTDB database (R07-RS207) was used for bacterial and archaea identification. The resulting taxonomy and abundance information were further analyzed: (1) to perform alpha- and beta-diversity analyses; (2) to create microbial composition barplots with QIIME (Caporaso et al., 2010); and (3) to create taxa abundance heatmaps with hierarchical clustering (based on Bray-Curtis dissimilarity).
Information about the overall performance of RAnDMBr during the treatment of HTL-aq can be found in Figure 1; Supplementary Figure S3.
Figure 1. Chemical oxygen demand (COD) removal and methane yield (A), hydraulic retention time (HRT) and organic loading rate (OLR) (B), and pH and volatile fatty acids (VFA) accumulation (C). The vertical line indicates changes made to the OLR. The blue vertical line indicates when the nutrient solution started to be added to the feedstock.
The system had a period of acclimatation of about 37 days before the COD removal (69.0% ± 6.9% from day 30–115) and methane yield (0.22 ± 0.03 LCH4 gCODfed−1 from day 30–115) were stable (Figure 1A). Such start-up period can happen when the source of inoculum used is not coming from a reactor treating the same type of substrate. Due to the lack of AD plants treating HTL-aq, an inoculum from a nearby AD bioreactor was used instead. This and the storage at 4°C for 17 days could be the reasons for the 37 days acclimation period. During the first 25 days, the pH decreased continuously from 8.1 to 6.9. To avoid inhibition by a low pH, the organic loading rate (OLR) decreased from 0.5 to 0.2 g COD LR−1 day−1 by increasing the HRT from 5 to 10 days, making the pH increase until reaching a stable value of 7.5 on day 85 (Figures 1B, C). The decrease in pH was not due to an imbalance of the microbial community because the VFA levels in the reactor were low (<0.09 g VFA as COD L−1), it was due to a dilution of the NH3 levels in the RAnDMBr caused by the continuous addition of the feedstock (Figure 1C, Supplementary Figures S3A, C). The inoculum used in this study came from a digester with high levels of total ammonia nitrogen (1101.1 ± 12.6 mg NH3-N L−1) while the feedstock used during the first 25 days was diluted by a factor of 30 and had a total ammonia concentration of 138 ± 38.3 mg NH3-N L−1 (Supplementary Table S1).
From day 75, the OLR was increased slowly by decreasing the dilution of HTL-aq in the feedstock and the process presented an imbalance when the OLR reached a value of 1.0 g COD LR−1 day−1 on day 116 (Figure 1). In 40 days (116–166) the COD removal decreased from 83.2% to 5% and methane production stopped. Increasing the concentration of HTL-aq in the feedstock generated an imbalance of the system that reduced the COD removal and increased the VFA levels from 0.1 g VFA as COD L−1–1.8 g VFA as COD L−1 in 40 days (Figure 1C; Supplementary Figure S3A). The increase in VFA levels was mostly due to the inhibition of methanogenic archaea since acetate represented 65%–68% of the total VFA. In order to recover the system, the OLR was reduced to 0.5 g COD LR−1 day−1 by decreasing the concentration of HTL-aq in the feedstock (see Table 1). This allowed the system to recover despite another imbalance due to an unexpected accident on day 205 where the reactor remained half empty for 5 days. Going back to an OLR of 0.5 g COD LR−1 day−1 allowed the VFA levels to decrease to 0.02 g VFA as COD L−1 and the COD removal and methane yield to increase to 62.6% ± 2.4% and 0.11 ± 0.06 LCH4 gCODfed−1, respectively (averages calculated from days 260–310) (Figure 1; Supplementary Figure S3). Inhibition due to the accumulation of VFAs when treating high concentrations of HTL-aq through AD has been reported earlier (Fernandez et al., 2018; Si et al., 2018; Watson et al., 2020; Li et al., 2022; Macêdo et al., 2024). However, other AD studies using biofilms in AD achieved a COD removal of 60%–80% with OLRs higher than 1.0 g COD LR−1 day−1 (close to 2 g COD LR−1 day−1) (Usman et al., 2019; Chen et al., 2020; Hao et al., 2020; Yang et al., 2020). One of the reasons the RAnDMBr could not work at an OLR higher than 0.5 g COD LR−1 day−1 could be the low nutrients concentration in the substrate (Supplementary Table S1). As seen in Supplementary Figure S4 the COD:P of the substrate and the digester content was not in the ideal range for anaerobic digestion (400-17) (Thaveesri et al., 1995; Janke et al., 2017). In this study, the HTL-aq was provided by PNNL and their HTL system has a settling separation vessel to enhance the separation of inorganics from the biocrude/HTL-aq mixture. The settling vessel promotes the separation of phosphates from the HTL-aq, producing a hydrochar rich in P that can use as fertilizer and an HTL-aq with very low concentration of P for bioprocesses (Elliott et al., 2016). The low levels of nutrients in HTL-aq made PNNL researchers to add trace metals when growing Scenedesmus obliquus DOE 0152.Z and Chlorella sorokiniana with HTL-aq from PNNL HTL system (Edmundson et al., 2017). To avoid inhibition in the system due to the lack of P and trace metals, on day 332 we started to mix the feedstock with a solution rich in nutrients (details about the nutrient stock solution can be found in Supplementary Table S4). On days 359 and 473 the OLR could increase to 1.0 g COD LR−1 day−1 and 1.5 g COD LR−1 day−1, respectively, without experiencing inhibition or a change in pH, VFA levels, COD removal or methane yield (Figure 1; Supplementary Figure S3). The need to add nutrients suggests that co-digestion with a substrate like sewage sludge, sludge centrate or HTL solids would be necessary to overcome the deficiency of P and other nutrients in HTL-aq (Edmundson et al., 2017).
The system was stable until day 521, when the OLR increased to 2.0 g COD LR−1 day−1 and the COD removal and methane yield decreased to 20.0% and 0.05 LCH4 gCODfed-1, respectively. During the last 79 days, the free ammonia levels were higher than ever (53.4–63.5 mg NH3-N L−1) as well as the VFA (maximum of 4.3 g of VFA as COD L−1). Acetate, propionate, butyrate, iso-butyrate, iso-valerate and caproate contributed to the VFA levels during this period. Increasing the concentration of HTL-aq in the feedstock could have led to inhibition due to an increase in concentration of free NH3 and phenolics, and nitrogen heterocyclic compounds (Si et al., 2018). Similarly, Li et al. (2022) experienced inhibition of the reactor when the proportion of HTL-aq in the feedstock increased. The increase in HTL-aq proportion in the feedstock led to an increase in the concentration of phenolics, and nitrogen heterocyclic compounds which inhibited the activity methanogenic and syntrophic populations (Si et al., 2018; Watson et al., 2020). While free ammonia is also associated to inhibition in AD, the values of free ammonia reported here (53.4–63.5 mg NH3-N L−1) were below the limit of what is considered inhibitory for AD (Chen et al., 2008). In another study, the use of HTL-aq in AD after ammonia stripping did not improve the stability of the digester under mesophilic conditions, suggesting that ammonia inhibition is not a problem (Cox and Eskicioglu, 2024). However, the conditions in Cox and Eskicioglu (2024) (HRT of 20 days and dilution factor of 9.0) were less aggressive than here (HRT of 6 days and dilution factor of 5.6).
Overall, the RAnDMBr could operate at and OLR of 1.5 ± 0.2 g COD LR−1 day−1 and HRT of 6.5 ± 0.6 days without inhibition and removing 65.0% ± 2.5% of the COD. As mentioned earlier, there have been reactor configurations that could work without inhibition at OLR>1.5 g COD LR−1 day−1 and still remove 60%–70% of the COD (Usman et al., 2019; Yang et al., 2020). These studies were using granulated activated carbon to grow biofilms. Granulated activated carbon have shown the advantage of promoting DIET and also absorbing recalcitrant compounds (Zhu et al., 2023). While these two factors were not tested in this study, it is possible that the RAnDMBr does not absorb recalcitrant compounds, making the system more susceptible to inhibition when increasing the OLR.
One of the challenges in the use of membrane bioreactors is membrane fouling, which increases cost of maintenance and operation through increased membrane cleaning, replacement costs and power requirement (Nabi et al., 2023). Membranes can get clogged by the accumulation and deposition of microbes, cell debris, collides and solute on the surface and in the pores of membranes, increasing TMP or decreasing flux and making it hard to achieve low HRTs (0–1 day). The dynamic membrane bioreactor used in this study is different from other systems because flux from the inside to the outside of the bioreactor was not continuous. Here, the reactor bulk liquid would be recirculating through the membrane back into the reactor for a period of time tR. For a period of time tP the bulk liquid would cross the membrane and be discharged as permeate (tp + tR = 24 h, see Supplementary Figure S1D). Values on the recirculation ratio and the reactor flux and TMP are presented in Figure 2.
Figure 2. Transmembrane pressure (TMP), flux and recirculation ration. The vertical line indicates changes made to the OLR. The flux and recirculation ratio are reported since day 0 but TMP was only measured from day 92–600.
For the first 15 days the flux increased gradually from 7.0 to 19.0 L m−2 h−1 (LMH) by increasing the speed of the peristaltic pump in order to decrease the HRT to 5 days (Figure 2). When the HRT was increased back to 10 days the flux was reduced from 19.0 to 7.5 LMH by decreasing the speed of the peristaltic pump. For the first 38 days the recirculation ratio was constant around 20.0 L LR−1 but the membrane started to show symptoms of fouling on day 38, reducing the flux to 3.1 LMH and increasing the HRT to 17–20 days. To bring the HRT back to 10 days, the flux decreased to 1.4 LMH by increasing the permeation time (tP). Increasing tP allowed us to discharge again 0.5 L of permeate per day to keep the HRT at 10 days, but tR needs to decrease when increasing tP, making the recirculation ratio to decrease from 20.0 L LR−1–7.0 L LR−1.
When the COD removal started to decrease on day 116 it was decided to increase the recirculation to 5.5 on day 155 in order to give more opportunity to the microbial community in the biofilm to degrade COD further (Figure 2). This strategy was not successful because, as mentioned in the previous section, nutrients needed to be added to the feedstock. From days 155–359 the reactor operated at a recirculation ratio of 5.5, a TMP of 69.6 ± 5.27 kPa and a flux of 1.1 ± 0.3 LMH (Figure 2). On day 359 it was decided to decrease the HRT to 5 days by increasing tP and decreasing tR, which made the flux, the recirculation ratio and the TMP to decrease (averages from days 359–472, see Figure 2). Increasing the OLR made the TMP to increase to 73.4 ± 2.8 kPa keeping the flux (0.6 ± 0.1 LMH) and recirculation ratio (1.5 L LR−1) to similar values (average from days 473–600).
For most of the days, the RAnDMBR operated at fluxes of 0.5–1.5 LMH, which would be considered very low for typical dynamic membrane bioreactors that usually operate at HRTs lower than 1 day (Fairley-wax et al., 2022; Nabi et al., 2023; Samaei et al., 2023). In this study the RAnDMBR could work at an HRT of 5.6 ± 2.3 days without the need of fouling mitigation strategies. However, having such a low flux made the RAnDMBR to operate at a low recirculation ratio too (1.5 L LR−1). The purpose for using the RAnDMBR is to promote biofilm growth and extent the contact between substrate and microorganisms by providing advective substrate transport. Increasing the recirculation ratio would promote advective transport and biofilm growth. Therefore, it could be possible to increase the OLR and operate the RAnDMBr without inhibition by applying recirculation ratios higher than 1.5 L LR−1. However, this would require the implementation of a fouling mitigation strategy. There are different fouling mitigation strategies that have been tested in anaerobic membrane bioreactors. Some of them are physical methods (e.g., gas sparging and vibration), chemical methods (e.g., cleaning the membrane with hypochlorite, ozonation or UV), biological methods (e.g., quorum quenching and bacteriophages) or electrochemical methods (e.g., electro-coagulation or reactive electrochemical membranes). Some reviews have been published having more information about the different methods (Tomczak et al., 2023; Min et al., 2024). Future research should focus on comparing fouling mitigation strategies that are not cost-intensive (like relaxation) to increase the RAnDMBR recirculation ratio and prove this hypothesis (Samaei et al., 2023).
Figure 3; Supplementary Figure S5 show the microbial community composition in the biofilm and suspended biomass (bulk liquid) samples collected from the reactor. We were able to get resolution of our microbial community up to the genus level. While this is not enough to have a very thorough detail of the mechanisms taking place in the reactor it gives information about some of the microbial reactions that were taking place during our experiment. Overall, we see populations that are crucial for the degradation of HTL-aq such as genera able to degrade aromatic compounds (Pseudomonas_C, Anaerolinaceae_T78, Acinetobacter and Syntrophorhabdus) able to perform DIET and syntrophy (Syntrophosphaera, Cloacimonas, Syntrophus) and methanogens (Methanothrix, Methanobacterium, Methanosarcina and Methanospirillum). Such populations have also been identified in other studies where HTL-aq was degraded through AD (Chen et al., 2017; Si et al., 2018; Usman et al., 2019; Yang et al., 2020; Mao et al., 2021; Wang et al., 2022). Figure 4 shows a Principal Coordinate Analysis (PCOA) of the suspended biomass and biofilm samples.
Figure 3. Relative abundance of dominant groups in the inoculum and biofilm samples collected from the recirculating anaerobic dynamic membrane bioreactor over time. Only genera present at relative activities greater than 1% in at least 6 samples or present at a relative abundance of 5% at least once are shown.
Figure 4. Principal coordinate analysis (PCOA) plot based on Bray-Curtis distance of microbial community in inoculum, biofilm and suspended biomass samples over time. The numbers represent the days of sampling.
Changes in the OLR/HRT (days 25, 75, 115, 166, 359, 473, 521, and 600), the inhibition episodes (days 162 and 212) and the addition nutrients (day 332) could be a cause for the microbial community to change. However, only inhibition had the most drastic effect on the microbial community structure. The increase in VFA during the inhibition of the reactor caused changes in the methanogenic populations. For example, methanogens that are more resistant to high VFA levels (Methanobacterium) increased while Methanothrix decrease. After the reactor recovered on day 239 the microbial community structure did not go back its previous composition (Figure 4). In the suspended biomass and biofilm samples there was an increase in relative abundance of Bacteroidales_TTA-H9, Brachymonas, and Methanosarcina and a decrease in Methanothrix (Figure 3; Supplementary Figure S5). Brachymonas has been associated with the degradation of alicyclic hydrocarbons like phenol and production of EPS under anoxic conditions (Zheng et al., 2020). However, since the reactor operated under anaerobic conditions, it is unclear if this was its role. This is not the first time that Brachymonas is present at high relative abundance in AD and its function is still unresolved (Pervin et al., 2013). The increase in VFA levels (specially acetate) changed the methanogenic pathway from hydrogenotrophic to acetoclastic in the suspended biomass and biofilm samples between days 253–331. Before the inhibition event took place, the reactor probably produced methane by combining Syntrophosphaera, a syntrophic propionate oxidizer, with hydrogenotrophic methanogens (Methanothrix, Methanobacterium and Methanospirillum) (Figure 3; Supplementary Figure S5). These two types of population were already present in the inoculum which presented high levels of ammonia (125.1 mg NH3-N L−1), a condition that would favor acetate and propionate syntrophic oxidation and hydrogenotrophic methanogenesis. The increase in acetate during the inhibition period increased the relative abundance of Methanosarcina to 4.4%–17.6% in the suspended biomass and in the biofilm. After day 331 the relative abundance of Methanosarcina decrease to 1.7% in the suspended biomass and biofilm samples, suggesting that the hydrogenotrophic pathway was again the dominant for methane production.
The addition of nutrients in the system from day 331 did not present a significant change in the microbial community. After that, the OLR increased to 1.1 g COD LR−1 day−1, 1.5 g COD LR−1 day−1 and 2.0 g COD LR−1 day−1. The increase in OLR caused changes in the microbial community, especially when the OLR increased to 2.0 g COD LR−1 day−1, although not as drastic as when the system was previously inhibited (Figure 4). The increase in VFA when the OLR increased to 2.0 g COD LR−1 day−1 increased the relative abundance of Methanosarcina to 5.5%. However, the relative abundance of methanogens in the biofilm and in the suspended biomass decreased, which could explain the decrease in COD removal (Supplementary Figures S6, S7).
The PCOA plot shows that the microbial community structure in the reactor changed with time and that the microbial community in the biofilm and suspended biomass samples collected on the same day were different (Figure 4). In comparison to the biofilm, the bulk liquid presented a higher relative abundance of genera able to degrade aromatic compounds (Supplementary Figure S7), a similar relative abundance of genera able to perform syntrophy or DIET (Supplementary Figure S8) and a lower relative abundance of genera able to perform methanogenesis (Supplementary Figure S6). It is common to see in anaerobic membrane bioreactors biofilms with a higher relative abundance and activity of methanogens (Smith et al., 2015). In fact, the high activity and presence of microbial populations in biofilms makes membrane bioreactors a very suitable technology to perform resource recovery at low HRTs (Smith et al., 2015; Fairley-wax et al., 2022; Shrestha et al., 2022). The fact that the RAnDMBr had a higher relative abundance of methanogens in the biofilm could be the reason that 65% of the HTL-aq COD was degraded at a low HRT (5 days). However, RNA-based analyses would be necessary to further confirm that the biofilm had a higher methanogenic activity as well. Moreover, determining if DIET took place in the biofilm would require more analysis than studying relative abundance only.
The results from this study provide information for process development and future applications. Future work should focus on the use of a co-substrate to supply the lack of P in the HTL-aq, and on the possibility to increase the OLR through the increase of the recirculation ration. Moreover, the RAnDMBR could be implemented in AD facilities by implementing an external membrane similar to the one used in this study.
The RAnDMBr was able to treat diluted HTL-aq (dilution factor of 7.5) and degrade 65% of the COD at 1.5 ± 0.2 g COD LR−1 day−1 and 5.6 ± 2.3 days producing 0.19 ± 0.02 LCH4 gCODfed−1. The system presented severe inhibition after the OLR increased to 2.0 g COD LR−1 day−1. During this study it was necessary to add a solution with nutrients in our feedstock to overcome P deficiency in HTL-aq. Future research should focus on the addition of a co-substrate to provide P and study the impact of the co-substrate addition on the reactor performance and on the microbial community structure and activity. Future research should also focus on increasing the recirculation ratio in the system by implementing fouling mitigation strategies. This could enhance advective substrate transport and enhance the degradation of recalcitrant compounds, allowing the system to operate at higher OLRs.
The original contributions presented in the study are included in the article/Supplementary Material, further inquiries can be directed to the corresponding author.
XF: Conceptualization, Data curation, Investigation, Methodology, Supervision, Writing–original draft. MB: Investigation, Writing–review and editing. AP: Investigation, Writing–review and editing. MT: Conceptualization, Writing–review and editing. AS: Conceptualization, Writing–review and editing. JN: Conceptualization, Funding acquisition, Supervision, Writing–review and editing.
The author(s) declare that financial support was received for the research and/or publication of this article. This work was supported by the Great Lakes Water Authority.
The authors would like to thank Tim Fairley-Wax for helpful discussions, and the GLWA team for their assistance with the bioreactor system and monitoring. This work was supported by the Great Lakes Water Authority.
The authors declare that the research was conducted in the absence of any commercial or financial relationships that could be construed as a potential conflict of interest.
The author(s) declare that no Generative AI was used in the creation of this manuscript.
All claims expressed in this article are solely those of the authors and do not necessarily represent those of their affiliated organizations, or those of the publisher, the editors and the reviewers. Any product that may be evaluated in this article, or claim that may be made by its manufacturer, is not guaranteed or endorsed by the publisher.
The Supplementary Material for this article can be found online at: https://www.frontiersin.org/articles/10.3389/fceng.2025.1515470/full#supplementary-material
Adedeji, O. M., Bauer, S. K., and Jahan, K. (2023). Anaerobic digestion of aqueous product of co-hydrothermal liquefaction of beverage waste and sewage sludge: reduction of toxicity and energy assessment. Energy Convers. Manag. 290, 117228. doi:10.1016/j.enconman.2023.117228
APHAAWWAWEF (2012). Standard methods for the examination of water and wastewater. 22nd Edn. Washington DC.
Azarmina, N., and Eskicioglu, C. (2025). Anaerobic co-digestion of hydrothermal liquefaction of aqueous phase with municipal sludge and dewatering centrate. J. Environ. Manage 375, 124254. doi:10.1016/j.jenvman.2025.124254
Basar, I. A., Liu, H., Carrere, H., Trably, E., and Eskicioglu, C. (2021). A review on key design and operational parameters to optimize and develop hydrothermal liquefaction of biomass for biorefinery applications. Green Chem. 23, 1404–1446. doi:10.1039/d0gc04092d
Basar, I. A., Liu, H., and Eskicioglu, C. (2023). Incorporating hydrothermal liquefaction into wastewater treatment – Part III: aqueous phase characterization and evaluation of on-site treatment. Chem. Eng. J. 467, 143422. doi:10.1016/j.cej.2023.143422
Bolger, A. M., Lohse, M., and Usadel, B. (2014). Trimmomatic: a flexible trimmer for Illumina sequence data. Bioinformatics 30, 2114–2120. doi:10.1093/bioinformatics/btu170
Bueno, B. E., Quispe-arpasi, D., Soares, L. A., Kimiko, I., Bernadete, M., Varesche, A., et al. (2021). Continuous anaerobic treatment of the aqueous phase of hydrothermal liquefaction from spirulina using a horizontal-flow anaerobic immobilized biomass (HAIB) reactor. Water Air Soil Pollut. 232, 97. doi:10.1007/s11270-021-05025-2
Caporaso, J. G., Kuczynski, J., Stombaugh, J., Bittinger, K., Bushman, F. D., Costello, E. K., et al. (2010). QIIME allows analysis of high-throughput community sequencing data. Nat. Methods 7, 335–336. doi:10.1038/nmeth.f.303
Chen, H., Hao, S., Chen, Z., O-Thong, S., Fan, J., Clark, J., et al. (2020). Mesophilic and thermophilic anaerobic digestion of aqueous phase generated from hydrothermal liquefaction of cornstalk: molecular and metabolic insights. Water Res. 168, 115199. doi:10.1016/j.watres.2019.115199
Chen, H., Wan, J., Chen, K., Luo, G., Fan, J., Clark, J., et al. (2016). Biogas production from hydrothermal liquefaction wastewater (HTLWW): focusing on the microbial communities as revealed by high-throughput sequencing of full-length 16S rRNA genes. Water Res. 106, 98–107. doi:10.1016/j.watres.2016.09.052
Chen, H., Zhang, C., Rao, Y., Jing, Y., Luo, G., and Zhang, S. (2017). Methane potentials of wastewater generated from hydrothermal liquefaction of rice straw: focusing on the wastewater characteristics and microbial community compositions. Biotechnol. Biofuels 10, 1–16. doi:10.1186/s13068-017-0830-0
Chen, Y., Cheng, J. J., and Creamer, K. S. (2008). Inhibition of anaerobic digestion process: a review. Bioresour. Technol. 99, 4044–4064. doi:10.1016/j.biortech.2007.01.057
Cohen, Y. (2011). Biofiltration - the treatment ofluids by microorganisms immobilized into the filter bedding material: a review. Bioresour. Technol. 77, 257274. doi:10.1016/s0960-8524(00)00074-2
Cox, A. E., and Eskicioglu, C. (2024). Ammonia recovery via stripping from hydrothermal liquefaction aqueous from sludge for anaerobic co-digestion pretreatment. Chem. Eng. J. 496, 153715. doi:10.1016/j.cej.2024.153715
Cronin, D., Schmidt, A. J., Billing, J., Hart, T. R., Fox, S. P., Fonoll, X., et al. (2022). Comparative study on the continuous flow hydrothermal liquefaction of various wet-waste feedstock types. ACS Sustain Chem. Eng. 10, 1256–1266. doi:10.1021/acssuschemeng.1c07214
Edmundson, S., Huesemann, M., Kruk, R., Lemmon, T., Billing, J., Schmidt, A., et al. (2017). Phosphorus and nitrogen recycle following algal bio-crude production via continuous hydrothermal liquefaction. Algal Res. 26, 415–421. doi:10.1016/j.algal.2017.07.016
Elliott, D. C., Hart, T. R., Neuenschwander, G. G., Oyler, J. R., Rotness, J. L. J., Schmidt, A. J., et al. (2016). System and process for efficient separation of biocrudes and water in a hydrothermal liquefaction system.
Fairley-wax, T., Raskin, L., and Skerlos, S. J. (2022). Recirculating anaerobic dynamic membrane bioreactor treatment of municipal wastewater. ES&T Eng. 2, 842–852. doi:10.1021/acsestengg.1c00394
Fernandez, S., Srinivas, K., Schmidt, A. J., Swita, M. S., and Ahring, B. K. (2018). Anaerobic digestion of organic fraction from hydrothermal liquefied algae wastewater byproduct. Bioresour. Technol. 247, 250–258. doi:10.1016/j.biortech.2017.09.030
Hao, S., Choi, Y. J., Wu, B., Higgins, C. P., Deeb, R., and Strathmann, T. J. (2021). Hydrothermal alkaline treatment for destruction of per-and polyfluoroalkyl substances in aqueous film-forming foam. Environ. Sci. Technol. 55, 3283–3295. doi:10.1021/acs.est.0c06906
Hao, S., Ren, S., Zhou, N., Chen, H., Usman, M., He, C., et al. (2020). Molecular composition of hydrothermal liquefaction wastewater from sewage sludge and its transformation during anaerobic digestion. J. Hazard Mater 383, 121163. doi:10.1016/j.jhazmat.2019.121163
Janke, L., Weinrich, S., Leite, A. F., Schüch, A., Nikolausz, M., Nelles, M., et al. (2017). Optimization of semi-continuous anaerobic digestion of sugarcane straw co-digested with filter cake: effects of macronutrients supplementation on conversion kinetics. Bioresour. Technol. 245, 35–43. doi:10.1016/j.biortech.2017.08.084
Jiang, Y., Mevawala, C., Li, S., Schmidt, A., Billing, J., Thorson, M., et al. (2023). Uncertainty analysis for techno-economic and life-cycle assessment of wet waste hydrothermal liquefaction with centralized upgrading to produce fuel blendstocks. J. Environ. Chem. Eng. 11, 109706. doi:10.1016/j.jece.2023.109706
Kong, Z., Li, L., Xue, Y., Yang, M., and Li, Y. Y. (2019). Challenges and prospects for the anaerobic treatment of chemical-industrial organic wastewater: a review. J. Clean. Prod. 231, 913–927. doi:10.1016/j.jclepro.2019.05.233
Li, R., Liu, D., Zhang, Y., Tommaso, G., Si, B., Liu, Z., et al. (2022). Enhanced anaerobic digestion of post-hydrothermal liquefaction wastewater: bio-methane production, carbon distribution and microbial metabolism. Sci. Total Environ. 837, 155659. doi:10.1016/j.scitotenv.2022.155659
Liu, C., Yue, Z., Ma, D., Li, K., Zhao, P., Yang, M., et al. (2024). Integration of hydrothermal liquefied sludge as wastewater for anaerobic treatment and energy recovery: aqueous phase characterization, anaerobic digestion performance and energy balance analysis. J. Water Process Eng. 60, 105096. doi:10.1016/j.jwpe.2024.105096
Macêdo, W. V., Harpøth, R. D., Poulsen, J. S., de Jonge, N., Fischer, C. H., Agneessens, L. M., et al. (2024). Anaerobic digestion of wastewater from hydrothermal liquefaction of sewage sludge and combined wheat straw-manure. Bioresour. Technol. 399, 130559. doi:10.1016/j.biortech.2024.130559
Mah, T.-F. C., and O’toole, G. A. (2001). Mechanisms of biofilm resistance to antimicrobial agents. Trends Microbiol. 9, 34–39. doi:10.1016/s0966-842x(00)01913-2
Mao, L., Tsui, T., Zhang, J., Dai, Y., and Wah, Y. (2021). System integration of hydrothermal liquefaction and anaerobic digestion for wet biomass valorization: biodegradability and microbial syntrophy. J. Environ. Manage 293, 112981. doi:10.1016/j.jenvman.2021.112981
Marrone, P. A., Elliott, D. C., Billing, J. M., Hallen, R. T., Hart, T. R., Kadota, P., et al. (2018). Bench-scale evaluation of hydrothermal processing technology for conversion of wastewater solids to fuels. Water Environ. Res. 90, 329–342. doi:10.2175/106143017x15131012152861
Min, S., Lee, H., Deng, L., Guo, W., Xu, B., Yong Ng, H., et al. (2024). Advanced strategies for mitigation of membrane fouling in anaerobic membrane bioreactors for sustainable wastewater treatment. Chem. Eng. J. 485, 149996. doi:10.1016/j.cej.2024.149996
Mulchandani, A., and Westerhoff, P. (2016). Recovery opportunities for metals and energy from sewage sludges. Bioresour. Technol. 215, 215–226. doi:10.1016/j.biortech.2016.03.075
Nabi, M., Liang, H., Zhou, Q., Cao, J., and Gao, D. (2023). In-situ membrane fouling control and performance improvement by adding materials in anaerobic membrane bioreactor: a review. Sci. Total Environ. 865, 161262. doi:10.1016/j.scitotenv.2022.161262
Padoley, K. V., Mudliar, S. N., and Pandey, R. A. (2008). Heterocyclic nitrogenous pollutants in the environment and their treatment options - an overview. Bioresour. Technol. 99, 4029–4043. doi:10.1016/j.biortech.2007.01.047
Pervin, H. M., Batstone, D. J., and Bond, P. L. (2013). Previously unclassified bacteria dominate during thermophilic and mesophilic anaerobic pre-treatment of primary sludge. Syst. Appl. Microbiol. 36, 281–290. doi:10.1016/j.syapm.2013.03.003
Poirier, S., Bize, A., Bureau, C., Bouchez, T., and Chapleur, O. (2016). Community shifts within anaerobic digestion microbiota facing phenol inhibition: towards early warning microbial indicators? Water Res. 100, 296–305. doi:10.1016/j.watres.2016.05.041
Ripley, L. E., Boyle, W. C., and Converse, J. C. (1986). Improved alkalimetric monitoring for anaerobic digestion of high-strength wastes. J. Water Pollut. Control Fed. 58, 406–411.
Rittmann, B. E., Mayer, B., Westerhoff, P., and Edwards, M. (2011). Capturing the lost phosphorus. Chemosphere 84, 846–853. doi:10.1016/j.chemosphere.2011.02.001
Samaei, S. H. A., Chen, J., Li, G., Whitman, A., and Xue, J. (2023). Anaerobic dynamic membrane bioreactors (AnDMBRs): a promising technology for high-strength wastewater treatment. J. Environ. Chem. Eng. 11, 111139. doi:10.1016/j.jece.2023.111139
Seiple, T. E., Skaggs, R. L., Fillmore, L., and Coleman, A. M. (2020). Municipal wastewater sludge as a renewable, cost-effective feedstock for transportation biofuels using hydrothermal liquefaction. J. Environ. Manage 270, 110852. doi:10.1016/j.jenvman.2020.110852
Shen, Y., Linville, J., Urgun-Demirtas, M., Mintz, M., and Snyder, S. (2015). An overview of biogas production and utilization at full-scale wastewater treatment plants (WWTPs) in the United States: challenges and opportunities towards energy-neutral WWTPs. Renew. Sustain. Energy Rev. 50, 346–362. doi:10.1016/j.rser.2015.04.129
Shrestha, S., Xue, S., Kitt, D., Song, H., Truyers, C., Muermans, M., et al. (2022). Anaerobic dynamic membrane bioreactor development to facilitate organic waste conversion to medium-chain carboxylic acids and their downstream recovery. ACS ES&T Eng. 2, 169–180. doi:10.1021/acsestengg.1c00273
Si, B., Li, J., Zhu, Z., Shen, M., Lu, J., Duan, N., et al. (2018). Inhibitors degradation and microbial response during continuous anaerobic conversion of hydrothermal liquefaction wastewater. Sci. Total Environ. 630, 1124–1132. doi:10.1016/j.scitotenv.2018.02.310
Smith, A. L., Skerlos, S. J., and Raskin, L. (2015). Membrane biofilm development improves COD removal in anaerobic membrane bioreactor wastewater treatment. Microb. Biotechnol. 8, 883–894. doi:10.1111/1751-7915.12311
Snowden-Swan, L., Billing, J., Thorson, M., Schmidt, A., Jiang, Y., Santosa, M., et al. (2021). Wet waste hydrothermal liquefaction and biocrude upgrading to hydrocarbon fuels: 2020 state of technology. Available online at: https://www.ntis.gov/about.
Snowden-Swan, L., Billing, J., Thorson, M., Schmidt, A., Santosa, M., Jones, S., et al. (2022). Wet waste hydrothermal liquefaction and biocrude upgrading to hydrocarbon fuels: 2021 state of technology. Available online at: https://www.ntis.gov/about%0Ahttps://www.osti.gov/biblio/1617028%0Ahttps://www.osti.gov/servlets/purl/1617028.
Thaveesri, J., Daffonchio, D., Liessens, B., Vandermeren, P., and Verstraete, W. (1995). Granulation and sludge bed stability in upflow anaerobic sludge bed reactors in relation to surface thermodynamics. Appl. Environ. Microbiol. 61, 3681–3686. doi:10.1128/aem.61.10.3681-3686.1995
Titus Brown, C., and Irber, L. (2016). sourmash: a library for MinHash sketching of DNA. J. Open Source Softw. 1, 27. doi:10.21105/joss.00027
Tomczak, W., Gryta, M., Grubecki, I., and Miłek, J. (2023). Biogas production in AnMBRs via treatment of municipal and domestic wastewater: opportunities and fouling mitigation strategies. Appl. Sci. Switz. 13, 6466. doi:10.3390/app13116466
Urgun-Demirtas, M., Dalke, R., and Pagilla, K. R. (2022). in Sludge management and utilization for decarbonization. Editors Z. Jason Ren, and K. Pagilla (London, United Kingdom: IWA Publishing). doi:10.2166/9781789061796
Usman, M., Cheng, S., Boonyubol, S., and Cross, J. S. (2023). The future of aviation soars with HTL-based SAFs: exploring potential and overcoming challenges using organic wet feedstocks. Sustain Energy Fuels 7, 4066–4087. doi:10.1039/d3se00427a
Usman, M., Hao, S., Chen, H., Ren, S., Tsang, D. C. W., O-Thong, S., et al. (2019). Molecular and microbial insights towards understanding the anaerobic digestion of the wastewater from hydrothermal liquefaction of sewage sludge facilitated by granular activated carbon (GAC). Environ. Int. 133, 105257. doi:10.1016/j.envint.2019.105257
Vanwonterghem, I., Jensen, P. D., Dennis, P. G., Hugenholtz, P., Rabaey, K., and Tyson, G. W. (2014). Deterministic processes guide long-term synchronised population dynamics in replicate anaerobic digesters. ISME J. 8, 2015–2028. doi:10.1038/ismej.2014.50
Wang, P., Sakhno, Y., Adhikari, S., Peng, H., Jaisi, D., Soneye, T., et al. (2021). Effect of ammonia removal and biochar detoxification on anaerobic digestion of aqueous phase from municipal sludge hydrothermal liquefaction. Bioresour. Technol. 326, 124730. doi:10.1016/j.biortech.2021.124730
Wang, Z., Zhang, C., Watson, J., Sharma, B. K., Si, B., and Zhang, Y. (2022). Adsorption or direct interspecies electron transfer ? A comprehensive investigation of the role of biochar in anaerobic digestion of hydrothermal liquefaction aqueous phase. Chem. Eng. J. 435, 135078. doi:10.1016/j.cej.2022.135078
Watson, J., Wang, T., Si, B., Chen, W. T., Aierzhati, A., and Zhang, Y. (2020). Valorization of hydrothermal liquefaction aqueous phase: pathways towards commercial viability. Prog. Energy Combust. Sci. 77, 100819. doi:10.1016/j.pecs.2019.100819
Werner, J. J., Garcia, M. L., Perkins, S. D., Yarasheski, K. E., Smith, S. R., Muegge, B. D., et al. (2014). Microbial community dynamics and stability during an ammonia-induced shift to syntrophic acetate oxidation. Appl. Environ. Microbiol. 80, 3375–3383. doi:10.1128/AEM.00166-14
Winchell, L. J., Wells, M. J. M., Ross, J. J., Kakar, F., Teymouri, A., Gonzalez, D. J., et al. (2024). Fate of perfluoroalkyl and polyfluoroalkyl substances (PFAS) through two full-scale wastewater sludge incinerators. Water Environ. Res. 96, e11009. doi:10.1002/wer.11009
Yang, L., Si, B., Zhang, Y., Watson, J., Stablein, M., Chen, J., et al. (2020). Continuous treatment of hydrothermal liquefaction wastewater in an anaerobic bio fi lm reactor: potential role of granular activated carbon. J. Clean. Prod. 276, 122836. doi:10.1016/j.jclepro.2020.122836
Zhen, X., Luo, M., Dong, H., Fang, L., Wang, W., Feng, L., et al. (2022). Effect of organic load regulation on anaerobic digestion performance and microbial community of solar-assisted system of food waste. Water Reuse 12, 260–273. doi:10.2166/wrd.2022.107
Zheng, M., Shi, J., Xu, C., Ma, W., Zhang, Z., Zhu, H., et al. (2020). Ecological and functional research into microbiomes for targeted phenolic removal in anoxic carbon-based fluidized bed reactor (CBFBR) treating coal pyrolysis wastewater (CPW). Bioresour. Technol. 308, 123308. doi:10.1016/j.biortech.2020.123308
Zhu, D., Wang, Z., Liu, K., Si, B., Yang, G., Tian, C., et al. (2023). Multi-cycle anaerobic digestion of hydrothermal liquefaction aqueous phase: role of carbon and iron based conductive materials in inhibitory compounds degradation, microbial structure shaping, and interspecies electron transfer regulation. Chem. Eng. J. 454, 140019. doi:10.1016/j.cej.2022.140019
Keywords: hydrothermal liquefaction, anaerobic dynamic membrane bioreactor, biofilm, biogas, aqueous by-product
Citation: Fonoll Almansa X, Baydoun M, Patel A, Thorson MR, Schmidt A and Norton JW Jr. (2025) Using a recirculating anaerobic dynamic membrane bioreactor to treat hydrothermal liquefaction aqueous by-product. Front. Chem. Eng. 7:1515470. doi: 10.3389/fceng.2025.1515470
Received: 22 October 2024; Accepted: 13 March 2025;
Published: 11 April 2025.
Edited by:
Angel Fernández Mohedano, Autonomous University of Madrid, SpainReviewed by:
M. Angeles De La Rubia, Autonomous University of Madrid, SpainCopyright © 2025 Fonoll Almansa, Baydoun, Patel, Thorson, Schmidt and Norton. This is an open-access article distributed under the terms of the Creative Commons Attribution License (CC BY). The use, distribution or reproduction in other forums is permitted, provided the original author(s) and the copyright owner(s) are credited and that the original publication in this journal is cited, in accordance with accepted academic practice. No use, distribution or reproduction is permitted which does not comply with these terms.
*Correspondence: Xavier Fonoll Almansa, eGF2aWVyLmZvbm9sbEB1dGV4YXMuZWR1
Disclaimer: All claims expressed in this article are solely those of the authors and do not necessarily represent those of their affiliated organizations, or those of the publisher, the editors and the reviewers. Any product that may be evaluated in this article or claim that may be made by its manufacturer is not guaranteed or endorsed by the publisher.
Research integrity at Frontiers
Learn more about the work of our research integrity team to safeguard the quality of each article we publish.