- Life Quality Engineering Interest Group, School of Chemical and Environmental Engineering, College of Chemistry, Chemical Engineering and Material Science, Soochow University, Suzhou, China
Unlike many accomplished fields, human nutrition lacks the support of engineering science. Here, the aspects of food processing in the gastro-intestinal (GI) tract are reviewed, highlighting the experimental chemical reaction engineering (CRE) approach to digestion studies in particular. As a first look at human nutrition in terms of conservation laws, the differential forms of mass and energy balances are presented, emphasizing the chemical and biochemical reaction rates of generation and consumption and the heats associated with these reactions, respectively. These rates and the heats should be very meaningful for understanding the dynamics of nutrition within the body, though they remain unknown. Without solving the differential equations, global integrations of the mass balances within each organ, up to the organ boundary, can create control volumes for gaining new insights, such as the transient multicomponent nature of the stomach “reactor” emptying. Global integration within the human body to the boundaries of the entire GI tract, from the mouth to anus, finds the GI tract to be a “pipe outside the body.” This has revealed interesting aspects, highlighting the human body as a “molecular machine.” It is envisaged that the terms outlined here ought to be established in the future to improve human nutrition.
Background–nutritional phenomena have strong links to chemical reaction engineering
Nutrition of the human body is supported normally by digesting foods and absorbing nutrients (Parker, 2001; Lanham-New et al., 2019; Aguilera, 2018; Snoeck and Van Rompaey, 2021; Halton and Hu, 2004; Westerterp-Plantenga, 2008). These are biochemical reactions coupled with passive and active separations in the “reactors” in the human gastro-intestinal tract. This is realized through eating, digestion, absorption, and fermentation in the gastro-intestinal (GI) tract (Aguilera, 2018; Yoo and Chen, 2006). They are multicomponent and often multiphasic (Bornhorst et al., 2016). Connecting the GI tract activities with what is going on inside the body fluids is expected to be a powerful means to understand human nutrition (Nadia et al., 2021a; Qin et al., 2020; Qin et al., 2022).
Scientific food design today starts with quantitative information on the molecular composition of the raw materials. Some materials are identified for their anticipated roles in supporting human health. Foods are processed ex vivo to increase shelf-life and to produce better consumer acceptance. Many processes, including cooking, create interesting microstructures. The functions of these microstructures must be expressed through in vivo processing in the human body. The oral processing in the “oral reactor” generates much of the pleasure. The perceptive fulfillment, including fullness, is reflected throughout the GI tract. Upon absorption into the body fluids, the molecules from digestion are expressed and used to support human life. This may be called a molecule-to-molecule approach.
There is, however, a gap in recognizing the usefulness of engineering in nutrition. This was mainly because engineering education typically lacks a curriculum that involves health sciences. Engineers, if interested in nutrition and health, would have to find a way to bridge the gap through self-learning, for instance. The author postulated that creating a near-real bionic in vitro digestion tract, a human model in particular, would create a path into the field of nutrition. Learning while building one, the learning becomes real. An in vitro system can be assessed wherever and whenever, facilitating sampling during digestion.
Over the past 2 decades, assembling artificial organs (the reactors) for near-real simulations has provided insightful observations into anatomy and physiology. One understands quantitatively the contribution from each small “bit” of an anatomical structure by comparing the overall performances when the bit is there or not there. One can choose to have that bit or not in the in vitro device and then measure the digestion rate, for instance. One example is the definitive effect of internal wrinkles on the digestion rate in an in vitro rat stomach system (Chen et al., 2013; Chen et al., 2016).
The author conceptualized the idea of “engineering of physiological phenomena” in the early 2000s. Figure 1 shows the details of the author’s lectures over the past 15 years. The organs that make up the GI tract are amazing soft-elastic chemical reactors.
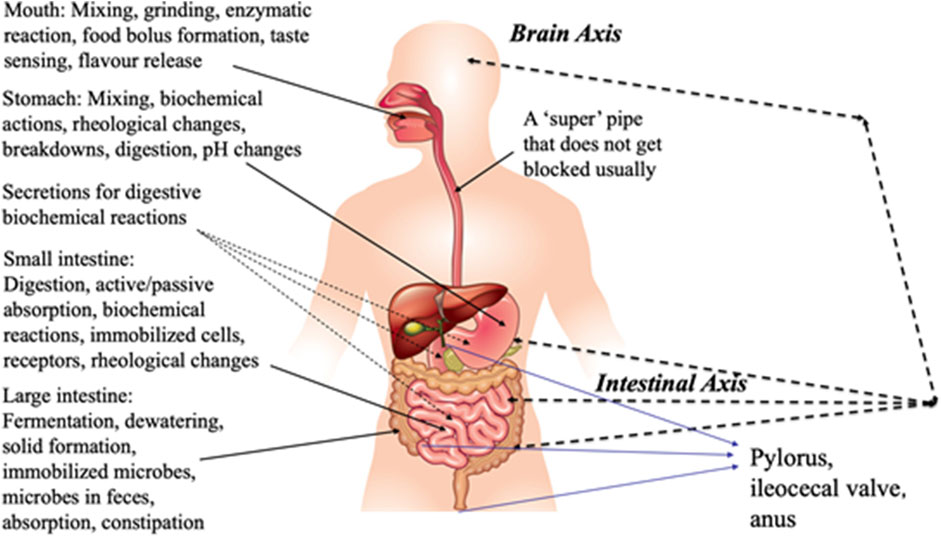
Figure 1. Schematic diagram showing the engineering aspects of the digestive processes (conceptualized by the author).
Important anatomical details cannot be ignored, such as the pylorus and the “valve” where the ileum joints into the cecum, that is, the ileocecal sphincter. The cecum, in chemical engineering, would be a “buffer tank.” This connects the upper stream operation in the ileum and the downstream operation in the ascending colon. Distinguished professor Jose Aguilera has drawn up unit operations known to chemical engineers to interpret the GI tract (Aguilera, 2018).
For industrial training purposes, the author has intuitively divided food processing into above-the-neck and below-the-neck processes to emphasize the nature of the activities that target different objectives. Production-oriented companies tend to work more toward satisfying the above-the-neck demands, such as appearance, taste, and flavor. In recent times, there has been a significant surge in consumer concern regarding below-the-neck issues relating to nutrition and health. Considering both above- and below-the-neck aspects makes a better food business today.
Correspondingly, in vitro (reactor) devices simulating digestion, absorption, and fermentation have been developed. The bionic aspects of in vitro devices have been enhanced considerably in more recent works (Chen et al., 2016; Bellmann et al., 2016; Wright et al., 2016; Barros et al., 2016; Wang et al., 2019; Peng et al., 2021; Wang et al., 2022; Shang et al., 2022; Ye et al., 2022).
Fermentation processes in the human colon reactor have been studied less due to the difficulty in setting up a representative culture (plus scientific feces) that can lead to a meaningful interpretation. A bionic in vitro colon-like reactor has only become available very recently (Hernalsteens et al., 2021).
Magnetic resonance imaging (MRI) has been used to observe quantitatively the behavior of an in vitro gastric simulator (to check the validity) as well as in vivo human stomach (protein digestion, protein gel digestion, emptying, and pH changes) (Deng et al., 2022; Deng et al., 2023; Deng et al., 2020). Measurements in vivo may give firsthand information about the real organ of concern and are likely to assist in the manufacture of more realistic in vitro systems.
Fluid mechanics inside the human stomach have been simulated using computational fluid dynamics (CFD) to reveal the nature of the mixing inside this unconventional engineering device (Ferrua and Singh, 2010; Xue et al., 2012; Alokaily et al., 2019; Li and Jin, 2020; Li et al., 2021). Simulations on pure fluid flow inside the small intestine have been carried out with increased vigor, including studies on the coupled mechanics involving intestinal walls (Qin et al., 2020; Buist et al., 2004; Lin et al., 2006; Zhang et al., 2022; Zha et al., 2021). Antral pulsation is of great importance in digestion; however, the biochemical reactions in the stomach that weaken the strength of the solids must also be very important and have not been incorporated.
Nutrition is no doubt most influential over our health. However, it is rare to discuss nutrition matters as transient multicomponent and multiphasic phenomena, especially from an engineering science viewpoint. Here, the principles of chemical reaction engineering manifested through the conservation of mass, energy, and momentum, coupled with reaction kinetics, are described to begin to look at human nutrition. In addition, without going into complex numerical schemes for solving the equations, some interesting observations can be made. The control engineering aspects are also described briefly to highlight what ought to be looked at in the near future.
Chemical reaction engineering of nutritional phenomena–—what we do not know yet
Figure 2A illustrates a fundamental chemical engineering system for the human body, where a network of circulations exists with various organ boundaries. In the structure of the system, an elemental control volume can be invoked, representing any point within the body (within any organ). The basic Newtonian approach is expected to write down mass, energy, and momentum balances differentially in Cartesian coordinates,
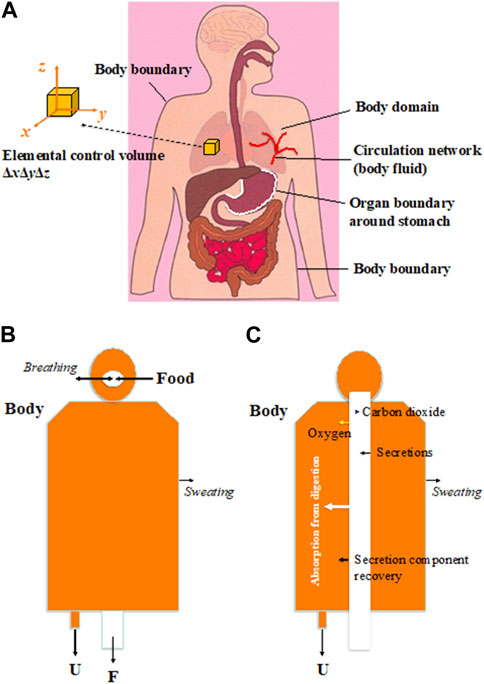
Figure 2. (A) Schematic diagram showing the control volumes in relation to human body; (B) Schematic diagram showing the global integration result of the mass balance over the entire human body (U is urine and F is fasces); (C) Schematic diagram showing the global integration result of the mass balance over the human body but up to the boundary of the GI tract. Oxygen and Carbon Dioxide are marked here as they are the most important; the idea expressed here does not exclude other gases that come in and out of the body.
The mass conservation equations for the molecular species describe their generation and consumption in the control volume and their transfer into and out of it through diffusion and convection. In terms of energy balance, it accounts for the heat associated with these reactions, as well as energy transport within and out of the system. Chemical energies and the works done are mentioned in nutrition texts, but these are typically not expressed in reaction kinetics; that is, they do not have a unit that contains time. These energies may be broadly “encapsulated” within the generation and consumption reactions mentioned here. Work done to support movements in the body, like momentarily expanding or shrinking, pulsating, and cognitive processes like thinking, all consume certain species and are associated with the changes in the species, as well as the gains or losses due to transport processes for a location. These activities may be reflected in thermal energy conservation.
The elemental control volume in Figure 2A can be placed in any organ or between organs where a set of reactions may happen.
The momentum conservation within the human body may cover actions of body fluid flows, organ contraction, and expansion. The changes in species type and concentration, which affect the physical fluid properties like viscosity, in particular, are important matters to consider.
The conservation equations can be written in differential forms aiming to evaluate the local rate of change, that is, the concentration of species (kg.m−3), thermal energy (J.m−3), and velocities (m.s−1), respectively. It is called the Eulerian approach. When following a species, or a mixture of species such as food, into the digestion tract and following what happens along the tract, the formulation can be made into a Lagrange expression. Both the Eulerian and Lagrange approaches can be employed to solve the same problem.
The molecular species and thermal energy balances may be written as follows:
In view of nutrition, the above two equations are the most important. The momentum equation for fluids is essentially the well-known Navier–Stokes equation, which can be found in basic fluid mechanics texts.
Equation 1 governs the mass conservation for species i, where ci is the concentration of species i (kg.m−3). Equation 2 represents energy conservation with contributions from the heat associated with the changes in the species in addition to the transport. The energy equation shows that the local temperature inside the human body is not necessarily constant. For simplicity, the incompressible fluid assumption is taken, so the pressure effect on thermal energy balance is neglected. k is the local heat conductivity (W.m−1.K−1) of the matrix of the body domain, which is a function of composition and temperature.
It is interesting to note that the approach described above yields equations like those commonly found in combustion science, where multispecies and multiphasic transportation coupled with chemical reactions are prevalent (Glassman, 1977; Williams, 1985).
The metabolism reactions are represented by
Di is the diffusion coefficient of species i in the body matrix (m.s−2) (Cussler, 1984), while h represents the heat of reaction per unit mass (J.kg−1). Additionally,
The velocity must be resolved with the momentum balance. A porous media approach may be considered when considering an organ. This approach necessitates a distinct treatment of the pressure effect and the media permeability effect on fluid flow compared to the conventional Navier–Stokes equation (Nield and Bejan, 1992).
Physiologically, work can occur throughout the body. This is accomplished by consuming the species of concern, ending with certain thermal effects (some may be too small to be noticed). The literature has extensively discussed the energy values of the nutritional components, such as proteins, fats, and carbohydrates (Lanham-New et al., 2019). These values, however, have not touched upon the rate phenomena, nor have they covered the issues of the spatial distribution in the body. Equations must account for the fact that most people have three meals a day at three (normally predictable) times, and some people additionally have coffee, tea, or other beverages that may have calories. These temporal behaviors cannot be overlooked.
Numerous ways to resolve partial differential equations of a similar nature have been achieved over many decades (Finlayson, 2012; Chapra and Canale, 2015). However, the emphasis here is not on solving them.
Integration of the conservation equations of mass and energy—what one may see
One can qualitatively and symbolically integrate the differential balances up to each organ boundary or the body boundary to gain some new insights into human nutrition.
Example 1: Stomach
Integration of mass conservation to the boundary of a stomach can be carried out as an example. The domain under investigation is the entire inner stomach. One observes the rates of mass flowing into the stomach, including foods, drinks, physiological secretions such as acid and enzymatic solutions responding to the intakes (considering the control engineering aspect), and the mass exiting from the pylorus. The mixing inside the stomach creates fascinating details illustrated numerically. However, previous studies generally do not account for realistic time-dependent influxes and outfluxes of a multicomponent nature.
In experimental studies, a food sample is fed into the stomach in one go (or over perhaps a fraction of time compared to the whole gastric emptying). The emptying process, starting from stomach retention being 100%, is described using a model like the following (Peng et al., 2021; Nadia et al., 2021b):
where y(t) is the gastric retention ratio at time t (min), and t1/2 (min) is the time required when half of the food sample is emptied. β is a constant that reflects the shape of the emptying curve. This is insufficient to describe the species-specific behavior, which is likely intermittent, that is, dependent upon food intake and the reactions occurring in the stomach. To provide a more suitable description, one needs to consider the flow of each species, including the reaction products.
The muscular mechanics of the intestinal tract have been preliminarily investigated with bioelectricity recording and mathematical modeling at the University of Auckland. The spatially distributed mechanical driving forces generated in the stomach motivate what is going out by creating a pressure head that overcomes the resistance of the pylorus (with a small opening of approximately 1–2 mm usually). This soft-elastic reactor behavior is fundamentally different from those in the rigid stirred tank reactors. A general principle of emptying must follow fluid mechanics (Nield and Bejan, 1992; White, 1974; Cengel and Cimbala, 2014).
The pylorus has the capacity to act like a sieve (Bornhorst et al., 2016; Wang et al., 2022). Larger particles encounter difficulty exiting the stomach. This sieve-like behavior adds another complexity to the emptying process. This behavior has not been reflected in existing models.
For the thermal aspect of the stomach, it is interesting to observe the temperature change due to the ingestion of hot drinks (Yang et al., 2023) or cold treats like ice cream. Indeed, all fluid properties, such as viscosity and dissolution, are sensitive to temperature variations. In addition, the reactions taking place in the stomach, whether acid-induced hydrolysis or enzymatic processes, may contribute significantly to the heat balance and have not been evaluated quantitatively.
Example 2: Whole body and that without the GI tract
If one integrates the mass conservation (1) from the inside of the body to the body boundary, as shown in Figure 2B, one would think that there are two major outlets for our comfort: urine (U) and feces (F), that is, a human has liquid- and solid-discharging capacities. Other mass changes, such as sweating and exhaling/inhaling, etc., are also important and seem to operate at molecular scales.
Consider integrating mass conservation (1) over the whole body except the GI tract (refer to Figure 2C). The GI tract is assumed to be “separated” by “an interface” from the remaining body. The other boundary is the body boundary.
It may come as a surprise that there is only one major outlet for mass discharge from the body, which is urination (U). The GI tract is now taken “out of the body,” a tubular reactor with its own input (say food) and output (the fecal discharge). The remaining body is a “molecular machine” in that sense, with the molecules from the digestive tract entering the body and participating in many reactions and transport processes that the body needs. The discharges are expected to occur in molecular form, too, whether in liquid or in gas.
One point to note is that the insoluble residues of modern medicines (including some nanoparticles) could go through important organs like the liver and pancreas, potentially causing fouling and blockages in conduits. Over-processed foods, not yet established with definitive mechanisms (Lane et al., 2024), may present a similar threat to our health. One may suspect that materials contained inside our body that are difficult to degrade into molecules should be considered hazardous.
If the supply of amino acids from the digestion process exceeds the demand for muscle growth, it may lead to surplus amino acids (Bender, 2012) being excreted in urine. Due to the less limiting condition for fat increase in men (Jequier, 2002), the excessive amounts of carbohydrates and fatty acids taken up from the GI tract are undesirable.
If one integrates the energy Equation 2 over the entire body, one concern about the mass inputs would only be that taken into the mouth (hot or cold edibles), breathing stuff and waste discharged as urine and feces, and some as sweat, etc. Some heat is dissipated into the environment by conduction, convection, radiation, breathing, urination, sweating, etc. Notably, the healthy human body temperature is approximately 36°C–37°C, while the comfortable environmental temperature is usually some 10°C–15°C lower. Most people experience maintaining a mild heat loss condition as a pleasant sensation, which is interesting.
There is little knowledge about the rates of reactions and the heats of reactions in the manner shown in Equations 1, 2 for nutrition. There is little doubt that, once established, these will be so useful. The conservation laws are a powerful framework that can lead to a quantitative understanding of human nutrition (Crovetti et al., 1997; Tappy, 1996; Hui et al., 2017).
Control engineering aspects that are unique for chemical reaction engineering in human nutrition
Many biological aspects need to be addressed in addition to a “pure” conservation analysis. The nerve system plays a key role, an aspect well related to process control in chemical engineering (Romagnoli and Palazoglu, 2020; Kibble and Halsey, 2009; Thompson, 2019).
(1) Hormones play a significant role in regulating various bodily functions, and the interplay between the nervous and endocrine systems is essential for maintaining homeostasis. This may be viewed as the catalytic effects as investigated in chemical reaction engineering (Aris, 1989; Bailey and Ollis, 1986; Cooper and Jeffreys, 1971; Levenspiel, 1999).
(2) Feedback loops play a crucial role in maintaining balance within the human body. These loops allow the body to continuously adjust and regulate its physiological processes. One notable example is the role of the nervous system in receiving signals from various receptors, processing information, and sending commands to effectors to regulate physiological processes. The activities in the GI tract are sensed by relevant receptors that are connected to the central nervous system. It is known that any problematics in the “GI tract axis (intestinal axis)” profoundly affect the “nerve axis (the brain axis),” given their central location and roles in the human body.
(3) The immune system is another vital component that interacts with the nervous and endocrine systems (hormonal effects, too) to protect the body from various threats, including infections and injuries. These immune responses may suitably be formulated using the rate constants depicted in the conservation laws such as Equations 1, 2.
(4) The body strives to maintain a stable internal environment through homeostasis and adaptation, similar to a thermo-static system. The time responses from the human body also vary with varying levels of physical activity, reflecting its dynamic nature.
Concluding remarks
This chemical reaction engineering approach to human nutrition draws attention to reaction kinetics within the body, considering the generation and consumption of specific molecules while noting the spatial and temporal effects. This approach offers new possibilities for understanding human nutrition. Human nutrition is dynamic! Viewing the human body as a molecular machine highlights the need for caution in nanotechnological applications in nutrition and health. This may partially explain the adverse effects of overly processed foods, which could create hard-to-biodegrade clusters that interfere with the healthy operations of the organs and their interconnected conduits. The insights discussed here are expected to apply to understanding the processes affecting animal nutrition as well.
Data availability statement
The original contributions presented in the study are included in the article; further inquiries can be directed to the corresponding author.
Author contributions
XDC: conceptualization, formal analysis, writing–original draft, and writing–review and editing.
Funding
The author(s) declare that financial support was received for the research, authorship, and/or publication of this article. The author acknowledges Soochow University’s support to meet the publication cost.
Acknowledgments
The author appreciates the helpful comments and encouragement from Associate Professor Peng Wu, Associate Professor Timothy V. Kirk and Professor Jie Xiao, colleagues at the School of Chemical and Environmental Engineering, Soochow University, Suzhou City, China.
Conflict of interest
The author declares that the research was conducted in the absence of any commercial or financial relationships that could be construed as a potential conflict of interest.
Publisher’s note
All claims expressed in this article are solely those of the authors and do not necessarily represent those of their affiliated organizations, or those of the publisher, the editors, and the reviewers. Any product that may be evaluated in this article, or claim that may be made by its manufacturer, is not guaranteed or endorsed by the publisher.
References
Aguilera, J. M. (2018). Food engineering into the XXI century. AIChE J. 64 (1), 2–11. doi:10.1002/aic.16018
Alokaily, S., Feigl, K., and Tanner, F. X. (2019). Characterization of peristaltic flow during the mixing process in a model human stomach. Phys. Fluids 31 (10), 103105. doi:10.1063/1.5122665
Bailey, J. E., and Ollis, D. F. (1986). Biochemical engineering fundamentals. 2nd edition. New York: McGraw-Hill Book Company.
Barros, L., Retamal, C., Torres, H., Zuniga, R. N., and Troncoso, E. (2016). Development of an in vitro mechanical gastric system (IMGS) with realistic peristalsis to assess lipid digestibility. Food Res. Int. 90, 216–225. doi:10.1016/j.foodres.2016.10.049
Bellmann, S., Lelieveld, J., Gorissen, T., Minekus, M., and Havenaar, R. (2016). Development of an advanced in vitro model of the stomach and its evaluation versus human gastric physiology. Food Res. Int. 88, 191–198. doi:10.1016/j.foodres.2016.01.030
Bender, D. A. (2012). The metabolism of “surplus” amino acids. Br. J. Nutr. 108, S113–S121. doi:10.1017/s0007114512002292
Bird, R. B., Stewart, W. E., and Lightfoot, E. N. (2002). Transport phenomena. 2nd Edition. New York: John Wiley and Sons.
Bornhorst, G. M., Gouseti, O., Wickham, M. S. J., and Bakalis, S. (2016). Engineering digestion: multiscale processes of food digestion. J. Food Sci. 81 (3), R534–R543. doi:10.1111/1750-3841.13216
Buist, M. L., Cheng, L. K., Yassi, R., Bradshaw, L. A., Richards, W. O., and Pullan, A. J. (2004). An anatomical model of the gastric system for producing bioelectric and biomagnetic fields. Physiol. Meas. 25, 849–861. doi:10.1088/0967-3334/25/4/006
Cengel, Y. A., and Cimbala, J. M. (2014). Fluid mechanics: fundamentals and applications. 3rd Edition. New York: McGraw-Hill.
Chapra, S. C., and Canale, R. P. (2015). Numerical methods for engineers, 7th education. New York: McGraw Hill Education.
Chen, L., Wu, X., and Chen, X. D. (2013). Comparison between the digestive behaviors of a new in vitro rat soft stomach model with that of the in vivo experimentation on living rats - motility and morphological influences. J. Food Eng. 117 (2), 183–192. doi:10.1016/j.jfoodeng.2013.02.003
Chen, L., Xu, Y., Fan, T., Liao, Z., Wu, O., Wu, X., et al. (2016). Gastric emptying and morphology of a 'near real' in vitro human stomach model (RD-IV-HSM). J. Food Eng. 183, 1–8. doi:10.1016/j.jfoodeng.2016.02.025
Cooper, A. R., and Jeffreys, G. V. (1971). Chemical kinetics and reactor design. Edinburgh: Oliver & Boyd.
Crovetti, R., Porrini, M., Santangelo, A., and Testolin, G. (1997). The influence of thermic effect of food on satiety. Eur. J. Clin. Nutr. 52, 482–488. doi:10.1038/sj.ejcn.1600578
Cussler, E. L. (1984). Diffusion – mass transfer in fluid systems. 1st Edition. Cambridge University Press.
Deng, R., Janssen, A. E. M., Vergeldt, F. J., Van As, H., Graaf, C. D., Mars, M., et al. (2020). Exploring in vitro gastric digestion of whey protein by time-domain nuclear magnetic resonance and magnetic resonance imaging. Food Hydrocoll. 99, 105348. doi:10.1016/j.foodhyd.2019.105348
Deng, R., Mars, M., Hanssen, A. E. M., and Smeets, P. A. M. (2023). Gastric digestion of whey protein gels: a randomized cross-over trial with the use of MRI. Food Hydrocoll. 141, 108689. doi:10.1016/j.foodhyd.2023.108689
Deng, R., Seimys, A., Mars, M., Janssen, A. E. M., and Smeets, P. A. M. (2022). Monitoring pH and whey protein digestion by TD-NMR and MRI in a novel semi-dynamic in vitro gastric simulator (MR-GAS). Food Hydrocoll. 125, 107393. doi:10.1016/j.foodhyd.2021.107393
Ferrua, M., and Singh, R. P. (2010). Modeling the fluid dynamics in a human stomach to gain insight of food digestion. J. Food Sci. 75 (7), R151–R162. doi:10.1111/j.1750-3841.2010.01748.x
Finlayson, B. A. (2012). Introduction to chemical engineering computing. 2nd edition. New Jersey: John Wiley and Sons.
Halton, T. L., and Hu, F. B. (2004). The effects of high protein diets on thermogenesis, satiety and weight loss: a critical review. J. Am. Coll. Nutr. 23 (5), 373–385. doi:10.1080/07315724.2004.10719381
Hernalsteens, S., Huang, S., Cong, H., and Chen, X. D. (2021). The final fate of food: on the establishment of in vitro colon models. Food Res. Int. 150, 110743. doi:10.1016/j.foodres.2021.110743
Hui, S., Ghergurovich, J. M., Morscher, R. J., Jang, C., Teng, X., Lu, W., et al. (2017). Glucose feeds the TCA cycle via circulating lactate. Nature 551, 115–118. doi:10.1038/nature24057
Incropera, F. P., Dewitt, D. P., Bergman, T. L., and Lavine, A. S. (2007). Fundamentals of heat and mass transfer. 6th Edition. USA: John Wiley and Sons.
Jequier, E. (2002). Pathways to obesity. Int. J. Obes. 26 (Suppl. 2), S12–S17. doi:10.1038/sj.ijo.0802123
Kibble, J. D., and Halsey, C. R. (2009). Medical physiology: the big picture. New York: The McGraw-Hill Companies, Inc. published by.
Lane, M. M., Famage, E., Du, S., Ashtree, D. N., McGuinness, A. J., Gauci, S., et al. (2024). Ultra-processed food exposure and adverse health outcomes: umbrella review of epidemiological meta-analyses. BMJ 384, e077310. doi:10.1136/bmj-2023-077310
S. A. Lanham-New, T. R. Hill, A. M. Gallagher, and H. H. Vorster (2019). Introduction to human nutrition, the nutrition society. 3rd Edition (Wiley-Blackwell).
Li, C., and Jin, Y. (2020). A CFD model for investigating the dynamics of liquid gastric contents in human-stomach induced by gastric motility. J. Food Eng. 110461. doi:10.1016/j.jfoodeng.2020.110461
Li, C., Xiao, J., Chen, X. D., and Jin, Y. (2021). Mixing and emptying of gastric contents in human-stomach: a numerical study. J. Biomechanics 118, 110293. doi:10.1016/j.jbiomech.2021.110293
Lin, A. S.-H., Buist, M. L., Smith, N. P., and Pullan, A. J. (2006). Modelling slow wave activity in the small intestine. J. Theor. Biol. 242 (2), 356–362. doi:10.1016/j.jtbi.2006.03.004
Nadia, J., Bronlund, J., Singh, R. P., Singh, H., and Bornhorst, G. M. (2021a). Structural breakdown of starch-based foods during gastric digestion and its link to glycemic response: in vivo and in vitro considerations. Compr. Rev. Food Sci. Food Saf. 20 (3), 2660–2698. doi:10.1111/1541-4337.12749
Nadia, J., Olenskyj, A. O., Stroebinger, N., Hodgkinson, S., Estevz, T., Subranmanian, P., et al. (2021b). Tracking physical breakdown of rice- and wheat-based foods with varying structures during gastric digestion and its influence on gastric emptying in a growing pig model. Food Funct. 12 (13), 4349–4372. doi:10.1039/d0fo02917c
Peng, Z., Wu, P., Wang, J., Dupont, D., Menard, O., Jeantet, R., et al. (2021). Achieving realistic gastric emptying curve in an advanced dynamic in vitro human digestion system: experiences with cheese-a difficult to empty material. Food Funct. 12, 3965–3977. doi:10.1039/d0fo03364b
Qin, Y., Chen, X. D., Yu, A., and Xiao, J. (2022). New understanding from intestinal absorption model: how physiological features influence mass transfer and absorption. AIChE J. 69. doi:10.1002/aic.18099
Qin, Y., Xiao, J., Wang, Y., Dong, Z., Woo, M. W., and Chen, X. D. (2020). Mechanistic exploration of glycemic lowering by soluble dietary fiber ingestion: predictive modeling and simulation. Chem. Eng. Sci. 228, 115965. doi:10.1016/j.ces.2020.115965
Shang, Y., Miao, J., Zeng, J., Zhang, T., Zhang, R., Zhang, B., et al. (2022). Evaluation of digestibility differences for apple polyphenolics using in vitro elderly and adult digestion models. Food Chem. 390, 133154. doi:10.1016/j.foodchem.2022.133154
Snoeck, J., and Van Rompaey, A. (2021). The future of foods: a new recipe for the food sector, Lannoo Publishers, Belgium
Tappy, L. (1996). Thermic effect of food and sympathetic nervous system activity in humans. Reprod. Nutr. Dev. 36, 391–397. doi:10.1051/rnd:19960405
Thompson, G. S. (2019). Understanding anatomy and physiology: a visual, auditory, interactive approach. 3rd Edition. Philadelphia: F.A. Davis Company.
Wang, J., Wu, P., Liu, M., Liao, Z., Wang, Y., Dong, Z., et al. (2019). An advanced near real dynamic in vitro human stomach system to study gastric digestion and emptying of beef stew and cooked rice. Food Funct. 10, 2914–2925. doi:10.1039/c8fo02586j
Wang, J., Wu, P., Wang, J., Wang, J., Gu, B., Ge, F., et al. (2022). In vitro gastric digestion and emptying of cooked white and brown rice using a dynamic human stomach system. Food Struct. 31, 100245. doi:10.1016/j.foostr.2021.100245
Westerterp-Plantenga, M. S. (2008). Protein intake and energy balance. Regul. Pept. 149, 67–69. doi:10.1016/j.regpep.2007.08.026
Williams, F. A. (1985). “Combustion theory,” in Combustion science and engineering. 2nd Edition. Boca Raton: CRC Press. doi:10.1201/9780429494055
Wright, N. D., Kong, F., Williams, B. S., and Fortner, L. (2016). A human duodenum model (HDM) to study transport and digestion of intestinal contents. J. Food Eng. 171, 129–136. doi:10.1016/j.jfoodeng.2015.10.013
Xue, Z., Ferrua, M. J., and Singh, P. (2012). Computational fluid dynamics modeling of granular flow in human stomach. Aliment. Hoy 21 (27), 3–14.
Yang, M., Ye, A., Yang, Z., Everett, D. W., Gilbert, E. P., and Singh, H. (2023). Effect of ingestion temperature on the pepsin-induced coagulation and the in vitro gastric digestion behavior of milk. Food Hydrocoll. 139, 108550. doi:10.1016/j.foodhyd.2023.108550
Ye, Q., Ge, F., Wang, Y., Wu, P., Chen, X. D., and Selomulya, C. (2022). Digestion of curcumin-fortified yogurt in short/long gastric residence times using a near-real dynamic in vitro human stomach. Food Chem. 372, 131327. doi:10.1016/j.foodchem.2021.131327
Yoo, J., and Chen, X. D. (2006). GIT physicochemical modeling-a critical review. Int. J. Food Eng. 2 (4), 1–10. doi:10.2202/1556-3758.1144
Zha, J., Zou, S., Hao, J., Liu, X., Delaplace, G., Jeantet, R., et al. (2021). The role of circular folds in mixing intensification in the small intestine: a numerical study. Chem. Eng. Sci. 229, 116079. doi:10.1016/j.ces.2020.116079
Keywords: human nutrition, chemical reaction engineering, engineering science, mass conversation, energy conservation, reaction kinetics, heat of reaction
Citation: Chen XD (2024) Chemical reaction engineering of nutritional phenomena in the human body. Front. Chem. Eng. 6:1480523. doi: 10.3389/fceng.2024.1480523
Received: 14 August 2024; Accepted: 26 September 2024;
Published: 21 October 2024.
Edited by:
Florent Allais, URD ABI - AgroParisTech Innovation, FranceReviewed by:
Petra Foerst, Technical University of Munich, GermanyCopyright © 2024 Chen. This is an open-access article distributed under the terms of the Creative Commons Attribution License (CC BY). The use, distribution or reproduction in other forums is permitted, provided the original author(s) and the copyright owner(s) are credited and that the original publication in this journal is cited, in accordance with accepted academic practice. No use, distribution or reproduction is permitted which does not comply with these terms.
*Correspondence: Xiao Dong Chen, xdchen@mail.suda.edu.cn