- Division of Sustainable Development, College of Science and Engineering, Hamad Bin Khalifa University, Qatar Foundation, Doha, Qatar
The transportation and consumption of kitchen food waste is a major contribution to greenhouse gas (GHG) emissions in global warming. To reduce this risk, it is important to recycle food waste into energy production and agricultural byproduct for nutrient management. Dark fermentation is one of the most suitable nutrient recovery techniques for generating hydrogen (H2) gas and serves as a clean energy carrier for a sustainable environment. Potatoes (Solanum tuberosum L.) and watermelon (Citrullus lanatus) are an important vegetable and fruit in demand in markets worldwide. Each year, almost 8,000 kilotons of potato peel is generated, with a GHG emission of 5 million tons of carbon dioxide (CO2) equivalent. More than 90% of watermelon rind is considered waste and is discarded. A small-scale preliminary study was conducted on these two waste products to produce H2 gas from potato peel, watermelon rind, and a mixture of peel and rind by the dark fermentation process. After volume analysis of the H2 gas produced, the remaining residue was used to produce biochar. The highest volume of 149 mL H2 gas was achieved from the peel, followed by 140 mL and 135 mL of H2 gas from the rind and the mixture of peel and rind, respectively, with a biomass pH of 4.7–5.6 and volatile solids (VS) of 77%–88%. The biochar produced from all the sample types was alkaline in nature with a pH of 7.88 ± 0.33, electrical conductivity of 0.38 ± 0.03 mS/cm, zeta potential of −25.12 ± 0.32 mV, and had a nutrient richness that could be beneficial for soil quality improvement and plant growth. However, the outcomes of this small-scale analysis cycle requires additional analytical outcomes with field application that targets the future scope of research on sustainable H2 production and agricultural application.
Highlights
• Dark fermentation technique was used to generate H2 gas from kitchen waste.
• A higher volume of H2 gas was produced from potato peel than watermelon rind.
• The biochar produced from fermented residue has favorable agriculture properties.
• The biochar is nutrient-rich and appropriate for agriculture application.
1 Introduction
Over the past decade, food waste, including production, transportation, consumption, and landfill, released greenhouse gases (GHGs), which are a significant contributor to global warming. To reduce the risk, the valorization of food waste to clean energy production and sustainable agricultural byproducts is a major concern (Foong et al., 2021). According to the Food and Agriculture Organization (FAO), approximately 1.3 billion tons of food per year are wasted globally (Seberini, 2020). Every year, the largest quantity of food, almost 361 kg per capita, is wasted in Australia (Srivastava et al., 2021), while 200 kg of food per capita is wasted in Sweden, 287 kg in the United States, 56 kg in Russia, 44 kg in China, and 51 kg of food per capita is wasted in India. Food waste is a promising resource for producing bioenergy because of its high content of organics, cellulose, starch, protein, and fat, which are the best carbon sources for dark fermentation to produce hydrogen (H2) gas (Srivastava et al., 2021; Talapko et al., 2023). Fermentative acetogenic bacteria hydrolyze and ferment carbohydrates, proteins, and lipids to volatile fatty acids (VFAs) to produce H2 gas (Sanchez-Ledesma et al., 2024). Of all food waste, fruit and vegetable waste, especially peel, is generated the most from kitchens and could be a potential source for generating H2 gas.
Potatoes (Solanum tuberosum L.) are the world’s fourth most important starchy tuberous vegetable; according to FAOUN (Food and Agricultural Organization of the United Nations), 376 million metric tons (mMT) of potatoes were produced worldwide in 2021 (Khanal et al., 2023). It is anticipated that approximately 8,000 kilotons of potato peel waste is generated annually with GHG emissions of 5 million tons of carbon dioxide (CO2) gas (Khanal et al., 2023). Another food waste, watermelon (Citrullus lanatus), is a major global economy fruit that is cultivated in almost 122 countries across many continents at a rate of 101 million tons (mT) per annum (Mamiru and Gonfa, 2023). Watermelon consists of approximately 2% seeds, 30% rind, and flesh. More than 90% of watermelon rind is dumped as waste into the environment, which constitutes an environmental challenge because of its rapid degradation (Bellary et al., 2016). Although there are many reports on the potential use of watermelon rind, the waste is not used on an industrial scale. The utilization of watermelon rind is economically very important as it contains carbohydrates, proteins, fats, minerals, and vitamins (Petkowicz et al., 2017). There are various social, economic, and environmental concerns associated with the management of potato peel and watermelon rind, especially in terms of GHG emissions and leachate generated from landfills (Abubakar et al., 2022). Currently, the major barrier to producing H2 by the dark fermentation process is the high cost of feedstock, which could be fulfilled by recycling waste biomass to minimize the ecological footprint. Dark fermentation is a promising technique to valorize food waste biomass as a substrate by considering different operational conditions such as pH, hydraulic retention time (HRT), and organic loading rate (Kim et al., 2009). Dark fermentation is capable of valorizing a wide range of waste biomass with a simple reactor design and a high production rate of bacterial degradation under anaerobic conditions in the absence of light (Bundhoo and Mohee, 2016; Mohanakrishna et al., 2023).
Therefore, the techno-economic feasibility of the conversion of these two food wastes must be considered to produce important byproducts—mostly VFAs from dark fermentation (Strazzera et al., 2018). Volatile fatty acids are the byproduct produced from dark fermentation and have important carbon compounds such as formic acid, acetic acid, propionic acid, butyric acid, and valeric and caproic acids, which play a crucial role in chemical industries. VFAs have been used greatly to generate paraffins, carbonyl derivatives, and alcohols (Dahiya et al., 2018) in chemical industries and as a potential substrate for biopolymer production through polyhydroxyalkanoates (Chalima et al., 2017). Osman et al. (2024), (Osman et al., 2023) reported in a recent advanced study innovations of low-energy processes for organic biomass synthesis which could offer valuable insights to improve conversion processes. Dark fermentation is recommended as an excellent alternative for synthesizing bioproducts and extracting bioactive compounds with high industrial-added product that could expand the circular bioeconomy (Mu et al., 2018).
At present, the food market faces direct or indirect problems due to the drastic increase in fertilizer prices (Grzebisz and Łukowiak, 2021; Khan et al., 2024). To overcome this situation in the food production sector, farmers should pay more attention to using byproducts which are nutrient-rich and generated from waste biomass (Stepaniuk and Głowacka, 2022). Agricultural production is directly affected by climate variables such as temperature and precipitation which control crop growth and yield. Water deficit resulting from drought reduces plant growth and crop yield because of its negative impacts on plant growth (Karl et al., 2009). Agricultural crops have different water needs because some crops use water more efficiently than others. A drought can reduce crop yields due to less water and soil moisture. Biochar is a carbonaceous compound available from various biomasses such as wood, sawdust, and rice husk (Abdelaal et al., 2019) and is beneficial for both energy and agricultural practices. Biochar has a high cation exchange capacity (CEC), a high zeta potential, and a large surface area (Pradhan et al., 2020) to improve soil quality, soil-water, and nutrient retention and enhance plant growth (Lehmann and Joseph, 2009; Danish et al., 2024).
Biochar is generated by the pyrolysis process from different types of waste biomass in absence of oxygen at temperatures of 400–800°C (Tripathi et al., 2016; Khan et al., 2024). Of the advanced techniques, the valorization of food waste by pyrolysis allows the recovery of 80% of energy via pyrolyzed products such as biochar, bio-oil, and syngas with high calorific values (Parthasarathy et al., 2021; Rijo et al., 2023). The biochar has a massive impact on satisfying energy supply in the form of heat and represents the most practical real-world application for preventing global warming by capturing and sequestering atmospheric carbon (Andrzejewski et al., 2022). Osman et al. (2022) reported that biochar could be effectively utilized to store carbon in different sinks and improve soil physicochemical properties which are essential for a comprehensive understanding of biochar’s role in carbon sequestration, certain regulations, and the thresholds needed for biochar to be used in agronomy.
This literature underscores the significance of several key parameters in H2 production processes, but most research has predominantly focused on maximizing H2 yield. Additionally, it is also essential to keep attention on the reuse of the residue generated after fermentation. Efforts in this field should not only prioritize maximizing H2 yield but also consider the environmental implications of fermentation byproducts. Addressing this residue and its potential environmental impact aligns with broader sustainability goals and ensures a comprehensive understanding of the overall ecological footprint of H2 production processes. However, H2 production from starchy food waste such as potato peels and natural sugar fruit waste like watermelon rinds and valorizing the discarded residue to biochar is inadequately represented in the literature. The selection of waste biomass for higher H2 production is also a major factor.
Therefore, this study aims to generate H2 gas from potato peel, watermelon rind, and a mixture of these by dark fermentation and produce biochar from the leftover fermented residue by pyrolysis. This study aims to conduct a comparative analysis to pick a suitable waste biomass for higher H2 production from solitary and mixed feedstocks. This work attempts a preliminary investigation to produce biochar from the discarded residue and analyze various properties from an agricultural point of view.
2 Materials and methods
2.1 Collection of waste and preparation of feedstock
Food waste was collected from the Hamad Bin Khalifa University food court kitchen waste bin and potato peel and watermelon rind segregated. These were chopped into small pieces (Figures 1A, B). The solid-state dark fermentation (SSF) process has been employed for the production of H2 gas using potato peel, watermelon rind, and mixed feedstock at a 1:1 ratio to produce H2 gas. The primary benefit of these approaches lies in their ability to minimize waste and liquid effluent, thereby creating minimal environmental impact. SSF utilizes uncomplicated natural solids as the medium, offering a low-technology solution to reducing energy requirements and a lower demand for capital investment. A detailed methodology of H2 gas production from kitchen wastes and the recycling of leftover residue to produce biochar is shown in Figures 1A, B, and a list of samples is reported in Table 1.
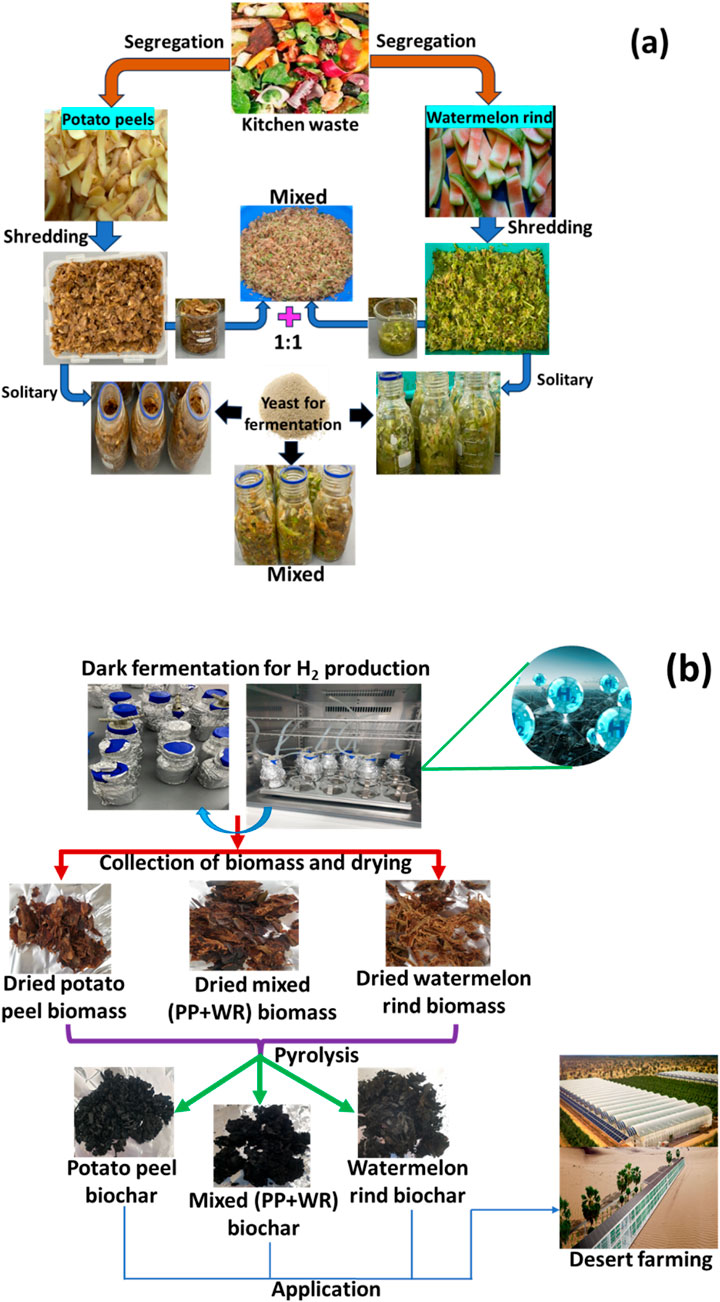
Figure 1. Detailed methodology of (A) feedstock preparation for dark fermentation process and (B) H2 gas and biochar production by dark fermentation and pyrolysis process, respectively.
Before proceeding with the H2 gas and biochar production, the initial pH, EC, total solids (TS), volatile solids (VS), and moisture content (MC) were measured following standard procedures (Turhal et al., 2019). Additionally, the pH and EC of the initial biomass of each source were measured before fermentation.
2.2 H2 gas production
After the preparation of feedstock, 150 g of each (potato peel, watermelon rind, and their mixture) was poured into 500-mL Schott bottles covered with aluminum foil (Figure 1) in triplicate. Anaerobic sludge was employed as an inoculum and subjected to heating at 100°C for 1 h to eliminate methanogenic bacteria. The pretreated sludge was allowed to cool at room temperature before being used in the experiments. The seed sludge was then grown in molasses for 20 h before inoculation, as per Turhal et al. (2019). Thereafter, 1 L of treated sludge was mixed with 0.5 g of baker’s yeast (Saccharomyces cerevisiae) to enhance H2 production by breaking down complex organic compounds into simpler sugars and organic acids, which are more easily fermentable by hydrogen-producing bacteria. Subsequently, 15 mL and 30 mL of the prepared inoculum were added to separate sets of triple bottles. No yeast was added to one set to serve as a blank for comparison of the yeast effect. Then, nitrogen (N2) gas was flushed for 5 min to supply anaerobic conditions before the bottles were tightly sealed with a rubber stopper. Following inoculation, the samples underwent agitation at 100 rpm within a dark shaker, maintaining a temperature of 35°C for two intervals of 24 and 48 h. The amount of H2 gas produced in the bottle was collected by a syringe after 24 and 48 h and measured. H2 yield was calculated as the cumulative volume of H2 produced per gram of volatile solids (mL H₂/g VS). Thereafter, the leftover residue was placed in a Fisher Scientific Isotemp mechanical convection laboratory oven at 105°C for 24 h for complete drying.
2.3 Biochar production
The dried biomass was used to produce biochar at 400°C by pyrolyzing in the absence of oxygen using a muffle furnace (Lindberg Blue M-3504) at a supply of 0.5 L/min N2 gas (Pradhan et al., 2020). This pyrolysis temperature was based upon previous research outcomes of the biochar properties for agriculture practice (Pradhan et al., 2020). The biomass and biochar were ground to finer particles to measure the pH, EC, and zeta (ζ) potential as per Pradhan et al. (2022) and Parthasarathy et al. (2021). After producing biochar at each temperature, the yield was determined using Equation 1:
The pH, EC, and zeta potential of biomass after fermentation and biochar produced from biomass were determined using an Orion Star A121 pH meter, A329 Thermo Scientific conductivity meter, and Zetasizer Nano-ZS (Malvern) meter, respectively. Samples were prepared by mixing media and water at a 1:10 ratio in a shaker for 1 h at 150 rpm before measurement of pH and EC (Dai et al., 2017; Pradhan et al., 2020). To standardize the pH levels, samples with lower pH were adjusted to 5.5 using a KOH solution.
The nutrient content of biomass and biochar was measured by an Agilent 5110 ICP-OES (inductively coupled plasma optical emission spectroscopy). Prior to sample analysis, the biomass and biochar samples were digested by microwave acid digestion. A sample weight of 200 mg was digested by 8 mL nitric acid (HNO3) and 2 mL hydrogen peroxide (H2O2) in an Ethos UP microwave digestion system (Milestone). After digestion, the samples were diluted with deionized water and filtered with 0.45-µm filter paper. A segmented flow analyzer (Sans+, Skalar) was used to measure the phosphorus concentration.
2.4 Statistical analysis
Statistical significance was determined using analysis of variance (ANOVA) with Fisher’s lowest significant difference test (LSD) at p < 0.05. The significance of variation was analyzed using a one-way and multivariate linear model in the Statistical Package for the Social Sciences (SPSS). All experiments were conducted in triplicate, and the results were presented as mean ± standard deviation.
3 Results and discussion
3.1 Characteristics of waste
The watermelon rind, potato peel, and their mixture exhibit approximately 6.8%–12% TS, 88%–95% MC, and 76%–88% VS (Figure 2A). Notably, the VS concentration in potato peel is higher than in watermelon rind, attributed to the elevated water content in watermelon. The initial pH values of the prepared samples ranged from 4.3 to 5.1, while the EC values were approximately 2 mS/cm (Figure 2B).
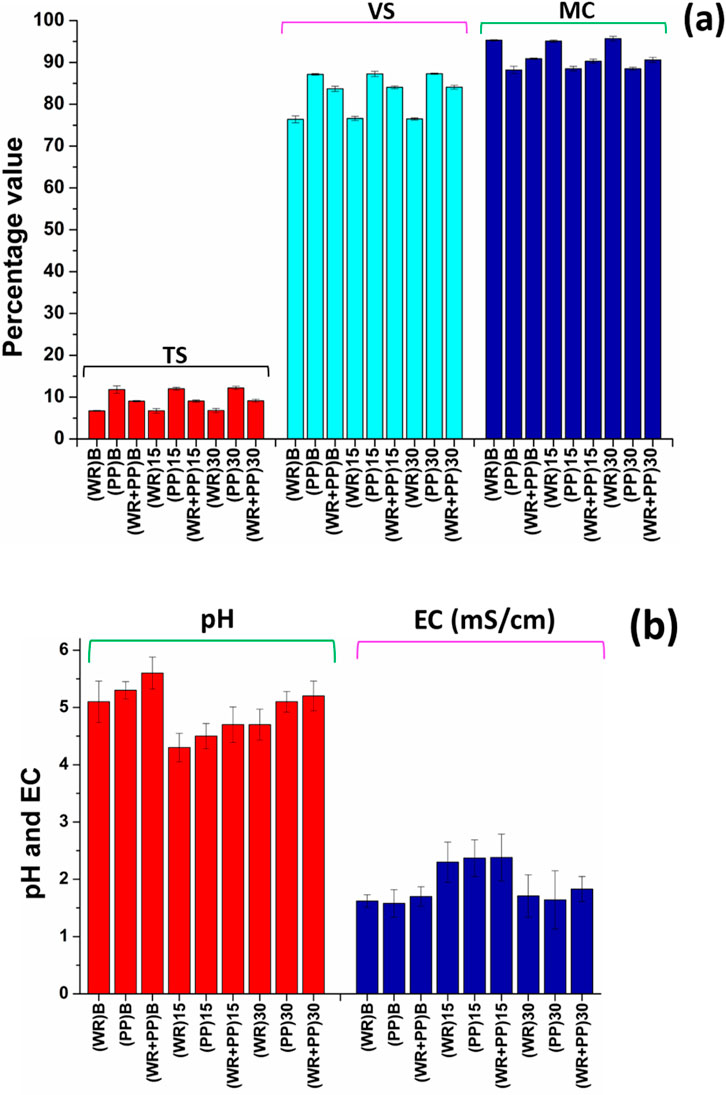
Figure 2. (A) TS (total solid), VS (volatile solid), and MC (moisture content) of the prepared feedstocks; (B) pH and EC (electrical conductivity) values of the feedstocks.
3.2 Quantification of H2 gas production
Many studies report that the H2 production rate remains stable beyond the 48-h mark by the dark fermentation process (Turhal et al., 2019; Dao et al., 2023). Consequently, H2 production yields were assessed at 24 and 48 h, and the volumes of generated H2 gas are illustrated in Figure 3A. Additionally, Figure 3B displays H2 production rates relative to the amount of VS removal rate. The calculated H2 production rate stands at approximately 50 mL/g VS removed. It is noteworthy that due to the higher water and sugar content in watermelon, an H2 production rate exceeding 50.5 mL/g VS removed was achieved. On the other hand, a greater volume of H2 was obtained with potato peel (p = 0.007); however, its production rate was observed to be lower than watermelon rind (p = 0.001). Notably, the application of yeast to the inoculum does not confer any advantages to H2 production. Cao et al. (2022) indicated a peak hydrogen yield of 71 mL/g of VS when utilizing aeration-enriched inoculum coupled with a notable achievement of 29% VS removal with an H2 yield of 71 mL/g from potato peel.
Turhal et al. (2019) used a mixture of melon and watermelon to produce H2 gas and observed that H2 production increased with increasing the substrate concentration because of higher initial sugar content at an elevated TS concentration. H2 production for natural microflora was 80.62 mLH2/Lreactor.h at 37 g TS/L, while it significantly increased to 351.12 mLH2/Lreactor.h at the same mixture concentration by external inoculation. This study reported that the most favorable nutrient and inoculum composition for H2 gas production was at 37 g TS/L. Vijayaraghavan et al. (2007) reported a mixed fruit peel waste of 46–84 g/L of VS produced 63% ± 2% H2 gas, while the average biogas generation was found to be 0.73 m3/kgVS. A maximum 932 mLH2/L.d of H2 could be generated with a 1-day HRT and a substrate loading rate of 90 g TOC/L.d from peach pulp (Dao et al., 2023). Our findings and its validation by previous research demonstrates that dark fermentation is as a great alternative for clean energy production.
3.3 Yield of biochar
After H2 production at two intervals (24 h and 48 h), the leftover residue was used to produce biochar, and the biochar yield was calculated. The yield of biochar for different feedstocks is shown in Figure 4A. The yield produced from watermelon rind was less than for potato peel and blended rind and peel (p = 0.001). A slight reduction in the yield of potato peel biochar was observed at 48 h in comparison to 24 h, whereas in the case of watermelon rind, biochar, and mixed biochar, the yield is almost identical (p = 0.88).
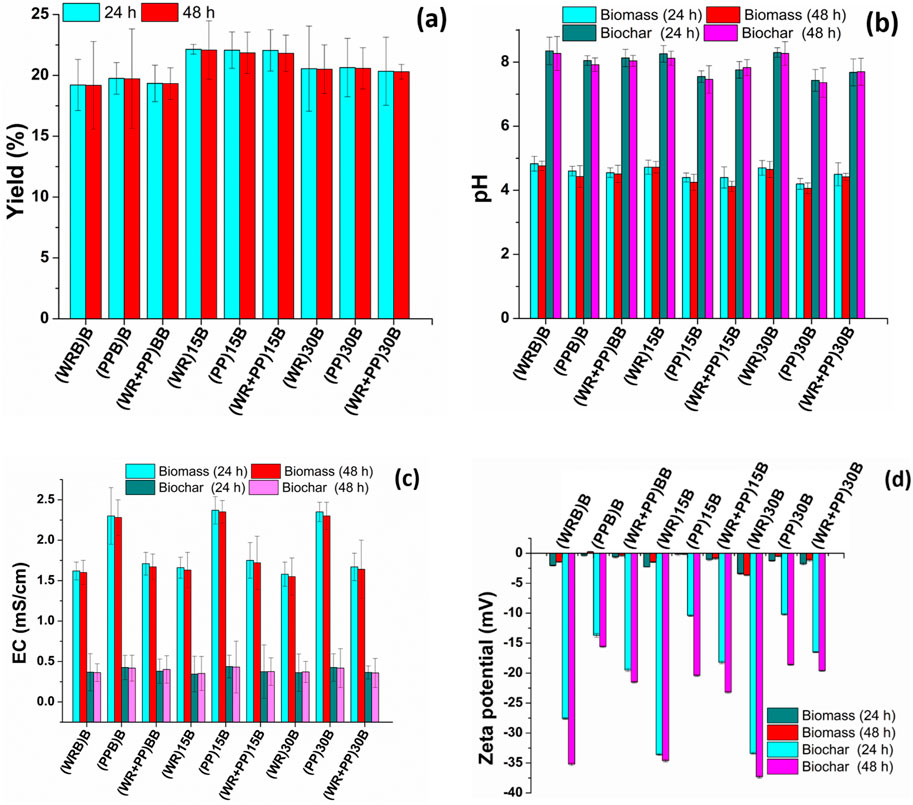
Figure 4. (A) Yield of biochar, (B) pH, (C) EC (electrical conductivity), and (D) zeta potential of biomass and biochar.
3.4 pH, EC, and zeta-potential
In each type of feedstock biomass, the pH was found <5 after 24 h of samples, while a slight reduction in pH was noticed after 48 h (Figure 4B). Compared to watermelon rind and mixed biomass samples, potato peel showed lower pH values (p = 0.02). It demonstrated more acidic compound formation that reflects the highest volume of H2 gas production. After biochar production from 24 h to 48 h feedstocks, the samples were alkaline in nature with a pH more than 7.5 in each feedstock type. A less significant variation was noticed in the pH of biochar produced at the 24 h and 48 h time spans (p = 0.53). Ding et al. (2016) reported that the application of higher alkaline biochar produced from agricultural waste could improve soil quality by reducing soil acidity and enhancing soil cation exchange capacity.
It was noticed that the application of the inoculum solution does not affect the EC of feedstock; therefore in each feedstock type, the blank (no yeast application) and fermented samples with inoculum solution showed equal EC (Figure 4C). It was observed that the potato peels had the highest EC values, and all other feedstock biomass had EC more than 1.5 mS/cm (p = 0.01). However, a tremendous reduction in EC was noticed in biochar for each feedstock type and was found to be less than 0.5 mS/cm (p = 0.001). A large variation was also noticed for zeta potential in biochar and biomass samples (Figure 4D). In each feedstock biomass type, the zeta potential tends to have less electronegativity strength, while after biochar production, the zeta potential increased and tended to have more electronegativity strength (>-15 mV), which could be beneficial for water and nutrient uptake after amending biochar with soil (Farhangi-Abriz and Ghassemi-Golezani, 2023). The more negative zeta potential of biochar enhanced cation exchange groups, which improves root cell walls and enhances maximum nutrient and the water sorption of plant roots (Farhangi-Abriz and Ghassemi-Golezani, 2023). This will also be more favorable for hydroponic agricultural production, with good crop yield and plant biomass (Wang et al., 2022).
3.5 Nutrient content of biomass and biochar
Considering these results, the nutrient content was measured for watermelon rind, potato peel, and their mixture for the blank samples only. The nutrient content in watermelon rind biomass is comparatively greater than potato peel biomass (Table 2). Biochar produced from all three types of feedstock had comparatively more nutrient concentration than biomass. Compared to individual potato peels and mixed biochar samples, watermelon rind biochar showed more nutrient content (p = 0.002). It was noticed that calcium (Ca), potassium (K), magnesium (Mg), and phosphorous (P) in watermelon rind biochar is higher than potato peel biochar (p < 0.03) compared to other nutrients. However, in each type of feedstock, biochar production was found to enhance nutrient content, which is appropriate to apply as an amendment to agricultural practices. Masto et al. (2013) reported that biochar generated from Lantana camara at 300°C was rich in nutrients such as P (0.64 mg/kg), K (711 mg/kg), Ca (5,880 mg/kg), and Mg (1,010 mg/kg). Similarly, biochar produced from other organic wastes had potential nutrient availability and could release large amounts of nutrient after addition to the soil (Mukherjee and Zimmerman, 2013; Zheng et al., 2013).
The business models for market value and economic potential demonstrate that biochar is a cost-effective amendment and carbon sequester with a wide range of environmental benefits associated with biochar application in agriculture production. The production cost of biochar is higher than the production cost of charcoal, but the return on investment is greater by reducing fertilizer cost, water demand cost, crop yield, and carbon value. Over the last decade, biochar production has established itself as a reliable way of valorizing food waste and fermented residue (Chen et al., 2014; Fawzy et al., 2022). Fawzy et al. (2022) confirmed that biochar production by pyrolysis is self-sufficient with the availability of surplus energy and represented 2.68 tCO2e per ton of biochar, whereas carbon emission costs vary according to feedstock costs and project strategy. Biochar achieved a return of 22.35% at a combined cost of EUR 110/ton CO2e removal and EUR 350/ton biochar with a feedstock cost of 45 EUR/ton, where service and product pricing are both within the lower bound of market pricing.
The assessment of net carbon management for recycling kitchen waste is an important pathway to reducing the environmental burden of agricultural production. Converting kitchen waste into biochar through pyrolysis is an important management strategy for achieving a circular carbon economy, generating both environmental and social benefits. Therefore, this study supports our upcoming research on the life cycle assessment (LCA) of the carbon footprint of the entire process, from waste collection to end-product biochar use.
4 Conclusion
This study is a preliminary laboratory-scale investigation on H2 gas and biochar production from two kitchen wastes which contain large amounts of starch, sugar, and cellulose. Dark fermentation is found promising for producing H2 gas directly from kitchen waste and the leftover residue for biochar production by pyrolysis for sustainable energy and crop production. A greater volume of H2 gas (150 mL) was produced from potato peel and less in watermelon rind. After fermentation, the biochar produced from fermented residue at 400°C was found to have the most beneficial properties for the sustainable amendment of soil-based and hydroponic agriculture. The suitable properties of biochar in comparison to biomass encourage the valorization of fermented residue by pyrolysis process as the best option for zero waste generation. However, this short-term analysis highlights the future direction of developing dark fermentation for long-term H2 gas production and biochar application for the real conditions of crop production. The novelty of this study encourages research on biochar-blended nutrient source production and application as a sustainable fertilizer solution for reducing traditional fertilizer costs. Additionally, this study aids the optimization of biochar production from fermented food residue by machine learning and modeling to interpret the influence of biochar properties in future agriculture production. This study encourages additional research in computational chemistry to control nutrient binding criteria.
Data availability statement
The raw data supporting the conclusions of this article will be made available by the authors, without undue reservation.
Author contributions
SP: conceptualization, formal analysis, investigation, methodology, and writing–original draft. BY: Conceptualization, formal analysis, methodology, and writing–original draft. YB: investigation, project administration, supervision, validation, and writing–review and editing. GM: conceptualization, funding acquisition, investigation, project administration, supervision, and writing–review and editing. TA-A: funding acquisition, project administration, supervision, and writing–review and editing.
Funding
The authors declare that no financial support was received for the research, authorship, and/or publication of this article.
Acknowledgments
The authors would like to thank Hamad Bin Khalifa University (Qatar Foundation) for supporting this research.
Conflict of interest
The authors declare that the research was conducted in the absence of any commercial or financial relationships that could be construed as a potential conflict of interest.
Publisher’s note
All claims expressed in this article are solely those of the authors and do not necessarily represent those of their affiliated organizations, or those of the publisher, the editors, and the reviewers. Any product that may be evaluated in this article, or claim that may be made by its manufacturer, is not guaranteed or endorsed by the publisher.
References
Abdelaal, A. H., McKay, G., and Mackey, H. R. (2019). Food waste from a university campus in the Middle East: drivers, composition, and resource recovery potential. Waste Manag. 98, 14–20. doi:10.1016/j.wasman.2019.08.007
Abubakar, I. R., Maniruzzaman, K. M., Dano, U. L., AlShihri, F. S., AlShammari, M. S., Ahmed, S. M. S., et al. (2022). Environmental sustainability impacts of solid waste management practices in the Global South. Int. J. Environ. Res. Public Health 19, 12717. doi:10.3390/ijerph191912717
Andrzejewski, J., Aniśko, J., and Szulc, J. (2022). A comparative study of biocarbon reinforced polyoxymethylene and polyamide: materials performance and durability. Compos. Part A Appl. Sci. Manuf. 152, 106715. doi:10.1016/j.compositesa.2021.106715
Bellary, A. N., Indiramma, A. R., Prakash, M., Baskaran, R., and Rastogi, N. K. (2016). Anthocyanin infused watermelon rind and its stability during storage. Innov. Food Sci. Emerg. Technol. 33, 554–562. doi:10.1016/j.ifset.2015.10.010
Bundhoo, M. A. Z., and Mohee, R. (2016). Inhibition of dark fermentative bio-hydrogen production: a review. Int. J. Hydrogen Energy 41, 6713–6733. doi:10.1016/j.ijhydene.2016.03.057
Cao, J., Xu, C., Zhou, R., Duan, G., Lin, A., Yang, X., et al. (2022). Potato peel waste for fermentative biohydrogen production using different pretreated culture. Bioresour Technol 362. doi:10.1016/j.biortech.2022.127866
Chalima, A., Oliver, L., Fernández de Castro, L., Karnaouri, A., Dietrich, T., and Topakas, E. (2017). Utilization of volatile fatty acids from microalgae for the production of high added value compounds. Fermentation 3, 54. doi:10.3390/fermentation3040054
Chen, T., Zhang, Y., Wang, H., Lu, W., Zhou, Z., Zhang, Y., et al. (2014). Influence of pyrolysis temperature on characteristics and heavy metal adsorptive performance of biochar derived from municipal sewage sludge. Bioresour. Technol. 164, 47–54. doi:10.1016/j.biortech.2014.04.048
Dahiya, S., Kumar, A. N., Shanthi Sravan, J., Chatterjee, S., Sarkar, O., and Mohan, S. V. (2018). Food waste biorefinery: sustainable strategy for circular bioeconomy. Bioresour. Technol. 248, 2–12. doi:10.1016/j.biortech.2017.07.176
Dai, Z., Zhang, X., Tang, C., Muhammad, N., Wu, J., Brookes, P. C., et al. (2017). Potential role of biochars in decreasing soil acidification - a critical review. Sci. Total Environ. 581–582, 601–611. doi:10.1016/j.scitotenv.2016.12.169
Danish, M., Pradhan, S., McKay, G., Al-Ansari, T., Mansour, S., and Mackey, H. R. (2024). Effect of biochar, potting mixture and their blends to improve ocimum basilicum growth in sandy soil. J. Soil Sci. Plant Nutr. 24, 1952–1967. doi:10.1007/s42729-024-01670-8
Dao, S., Turanbaev, M., and Argun, H. (2023). Dark fermentative hydrogen gas production from waste peach pulp by intermittent feeding: effects of hydraulic residence time and substrate loading rate. Int. J. Hydrogen Energy 48, 22889–22896. doi:10.1016/j.ijhydene.2023.01.122
Ding, Y., Liu, Y., Liu, S., Li, Z., Tan, X., Huang, X., et al. (2016). Biochar to improve soil fertility. A review. Agron. Sustain. Dev. 36, 36. doi:10.1007/s13593-016-0372-z
Farhangi-Abriz, S., and Ghassemi-Golezani, K. (2023). Improving electrochemical characteristics of plant roots by biochar is an efficient mechanism in increasing cations uptake by plants. Chemosphere 313, 137365. doi:10.1016/j.chemosphere.2022.137365
Fawzy, S., Osman, A. I., Mehta, N., Moran, D., Al-Muhtaseb, A. H., and Rooney, D. W. (2022). Atmospheric carbon removal via industrial biochar systems: a techno-economic-environmental study. J. Clean. Prod. 371, 133660. doi:10.1016/j.jclepro.2022.133660
Foong, S. Y., Chan, Y. H., Cheah, W. Y., Kamaludin, N. H., Tengku Ibrahim, T. N. B., Sonne, C., et al. (2021). Progress in waste valorization using advanced pyrolysis techniques for hydrogen and gaseous fuel production. Bioresour. Technol. 320, 124299. doi:10.1016/j.biortech.2020.124299
Grzebisz, W., and Łukowiak, R. (2021). Nitrogen gap amelioration is a core for sustainable intensification of agriculture—a concept. Agronomy 11, 419. doi:10.3390/agronomy11030419
Karl, T. R., Melillo, J., and Peterson, T. (2009). Global climate change impacts in the United States.
Khan, S., Irshad, S., Mehmood, K., Hasnain, Z., Nawaz, M., Rais, A., et al. (2024). Biochar production and characteristics, its impacts on soil health, crop production, and yield enhancement: a review. Plants 13, 166. doi:10.3390/plants13020166
Khanal, S., Karimi, K., Majumdar, S., Kumar, V., Verma, R., Bhatia, S. K., et al. (2023). Sustainable utilization and valorization of potato waste: state of the art, challenges, and perspectives. Biomass Conv. bioref. 14, 23335–23360. doi:10.1007/s13399-023-04521-1
Kim, D. H., Kim, S. H., and Shin, H. S. (2009). Hydrogen fermentation of food waste without inoculum addition. Enzyme Microb. Technol. 45, 181–187. doi:10.1016/j.enzmictec.2009.06.013
J. Lehmann, and S. Joseph (2009). Biochar for environmental management: science and technology (London; Sterling, VA: Earthscan).
Mamiru, D., and Gonfa, G. (2023). Extraction and characterization of pectin from watermelon rind using acetic acid. Heliyon 9, e13525. doi:10.1016/j.heliyon.2023.e13525
Masto, R. E., Ansari, Md. A., George, J., Selvi, V. A., and Ram, L. C. (2013). Co-application of biochar and lignite fly ash on soil nutrients and biological parameters at different crop growth stages of Zea mays. Ecol. Eng. 58, 314–322. doi:10.1016/j.ecoleng.2013.07.011
Mohanakrishna, G., Sneha, N. P., Rafi, S. M., and Sarkar, O. (2023). Dark fermentative hydrogen production: potential of food waste as future energy needs. Sci. Total Environ. 888, 163801. doi:10.1016/j.scitotenv.2023.163801
Mu, L., Zhang, L., Zhu, K., Ma, J., and Li, A. (2018). Semi-continuous anaerobic digestion of extruded OFMSW: process performance and energetics evaluation. Bioresour. Technol. 247, 103–115. doi:10.1016/j.biortech.2017.09.085
Mukherjee, A., and Zimmerman, A. R. (2013). Organic carbon and nutrient release from a range of laboratory-produced biochars and biochar–soil mixtures. Geoderma 193–194, 122–130. doi:10.1016/j.geoderma.2012.10.002
Osman, A., Zhang, Y., Lai, Z. Y., Rashwan, A., Farghali, M., Ahmed, A., et al. (2023). Machine learning and computational chemistry to improve biochar fertilizers: a review. Environ. Chem. Lett. 21, 3159–3244. doi:10.1007/s10311-023-01631-0
Osman, A. I., Ayati, A., Krivoshapkin, P., Tanhaei, B., Farghali, M., Yap, P.-S., et al. (2024). Coordination-driven innovations in low-energy catalytic processes: advancing sustainability in chemical production. Coord. Chem. Rev. 514, 215900. doi:10.1016/j.ccr.2024.215900
Osman, A. I., Fawzy, S., Farghali, M., El-Azazy, M., Elgarahy, A. M., Fahim, R. A., et al. (2022). Biochar for agronomy, animal farming, anaerobic digestion, composting, water treatment, soil remediation, construction, energy storage, and carbon sequestration: a review. Environ. Chem. Lett. 20, 2385–2485. doi:10.1007/s10311-022-01424-x
Parthasarathy, P., Mackey, H. R., Mariyam, S., Zuhara, S., Al-Ansari, T., and McKay, G. (2021). Char products from bamboo waste pyrolysis and acid activation. Front. Mater. 7, 482. doi:10.3389/fmats.2020.624791
Petkowicz, C. L. O., Vriesmann, L. C., and Williams, P. A. (2017). Pectins from food waste: extraction, characterization and properties of watermelon rind pectin. Food Hydrocoll. 65, 57–67. doi:10.1016/j.foodhyd.2016.10.040
Pradhan, S., Abdelaal, A. H., Mroue, K., Al-Ansari, T., Mackey, H. R., and McKay, G. (2020). Biochar from vegetable wastes: agro-environmental characterization. Biochar 2, 439–453. doi:10.1007/s42773-020-00069-9
Pradhan, S., Mackey, H. R., Al-Ansari, T. A., and McKay, G. (2022). Biochar from food waste: a sustainable amendment to reduce water stress and improve the growth of chickpea plants. Biomass Conv. bioref. 12, 4549–4562. doi:10.1007/s13399-022-02575-1
Rijo, B., Soares Dias, A. P., de Jesus, N., and Pereira, M. F. (2023). Home trash biomass valorization by catalytic pyrolysis. Environments 10, 186. doi:10.3390/environments10100186
Sanchez-Ledesma, L. M., Rodríguez-Victoria, J. A., and Ramírez-Malule, H. (2024). Effect of fermentation time, pH, and their interaction on the production of volatile fatty acids from cassava wastewater. Water 16, 1514. doi:10.3390/w16111514
Seberini, A. (2020). Economic, social and environmental world impacts of food waste on society and Zero waste as a global approach to their elimination. SHS Web Conf. 74, 03010. doi:10.1051/shsconf/20207403010
Srivastava, N., Srivastava, M., Abd_Allah, E. F., Singh, R., Hashem, A., and Gupta, V. K. (2021). Biohydrogen production using kitchen waste as the potential substrate: a sustainable approach. Chemosphere 271, 129537. doi:10.1016/j.chemosphere.2021.129537
Stepaniuk, M., and Głowacka, A. (2022). Yield of winter oilseed rape (Brassica napus L. var. napus) in a short-term monoculture and the macronutrient accumulation in relation to the dose and method of sulphur application. Agronomy 12, 68. doi:10.3390/agronomy12010068
Strazzera, G., Battista, F., Garcia, N. H., Frison, N., and Bolzonella, D. (2018). Volatile fatty acids production from food wastes for biorefinery platforms: a review. J. Environ. Manag. 226, 278–288. doi:10.1016/j.jenvman.2018.08.039
Talapko, D., Talapko, J., Erić, I., and Škrlec, I. (2023). Biological hydrogen production from biowaste using dark fermentation, storage and transportation. Energies 16, 3321. doi:10.3390/en16083321
Tripathi, M., Sahu, J. N., and Ganesan, P. (2016). Effect of process parameters on production of biochar from biomass waste through pyrolysis: a review. Renew. Sustain. Ener. Rev. 55, 467–481. doi:10.1016/j.rser.2015.10.122
Turhal, S., Turanbaev, M., and Argun, H. (2019). Hydrogen production from melon and watermelon mixture by dark fermentation. Intern. J. Hydrogen Energy 44, 18811–18817. doi:10.1016/j.ijhydene.2018.10.011
Vijayaraghavan, K., Ahmad, D., and Soning, C. (2007). Bio-hydrogen generation from mixed fruit peel waste using anaerobic contact filter. Intern. J. Hydrogen Energy 32, 4754–4760. doi:10.1016/j.ijhydene.2007.07.001
Wang, L., Olsen, M. N. P., Moni, C., Dieguez-Alonso, A., de la Rosa, J. M., Stenrød, M., et al. (2022). Comparison of properties of biochar produced from different types of lignocellulosic biomass by slow pyrolysis at 600°C. Appli. Energy Combust. Sci. 12, 100090. doi:10.1016/j.jaecs.2022.100090
Keywords: hydrogen, biochar, dark fermentation, agriculture, clean energy, sustainable environment
Citation: Pradhan S, Yuzer B, Bicer Y, McKay G and Al-Ansari T (2024) Hydrogen gas and biochar production from kitchen food waste through dark fermentation and pyrolysis. Front. Chem. Eng. 6:1450151. doi: 10.3389/fceng.2024.1450151
Received: 17 June 2024; Accepted: 22 October 2024;
Published: 14 November 2024.
Edited by:
Kavitha S., Karpagam Academy of Higher Education, IndiaReviewed by:
Ahmed Ibrahim Osman, Queen’s University Belfast, United KingdomElnaz Sohani, University of Nottingham, United Kingdom
Copyright © 2024 Pradhan, Yuzer, Bicer, McKay and Al-Ansari. This is an open-access article distributed under the terms of the Creative Commons Attribution License (CC BY). The use, distribution or reproduction in other forums is permitted, provided the original author(s) and the copyright owner(s) are credited and that the original publication in this journal is cited, in accordance with accepted academic practice. No use, distribution or reproduction is permitted which does not comply with these terms.
*Correspondence: Snigdhendubala Pradhan, c3ByYWRoYW5AaGJrdS5lZHUucWE=; Tareq Al-Ansari, dGFsYW5zYXJpQGhia3UuZWR1LnFh