- Sustainable Biofuels and Co-Products Unit, Eastern Regional Research Center, U.S. Department of Agriculture, Agricultural Research Service, Wyndmoor, PA, United States
In this work, the chemical composition, chemical structures, and sugar release were evaluated for sweet sorghum bagasse (SSB) biomass in response to alkaline pretreatments and enzymatic hydrolysis. Five different ratios of 2 M sodium hydroxide (NaOH) and sodium carbonate (
1 Introduction
Current energy, economic, and climatic factors are driving the search for fossil fuel substitutes that are renewable, locally accessible, and reduce greenhouse gas emissions (Condon et al., 2015; Paris, 2018; Gustavsson et al., 2021; Clauser et al., 2021). Lignocellulosic sources play a key role in these sectors due to their abundance of natural biopolymers found in plant cell walls. Composition varies depending on species, cultivar, and growing environment, but in general lignocellulosic plant cell walls contain cellulose (40%–50%), hemicellulose (20%–35%), and lignin (10%–25%) (de Jong, 2014; Lynd et al., 2017; Chandel et al., 2020; Zhang et al., 2021). All three components can be utilized in conversion processes that aim to produce bio-based fuels, chemicals, and materials (Baral et al., 2019). Regional variables, such as climate and soil conditions, are primary factors driving the decision-making of cultivating particular types of agricultural feedstocks. In the United States (U.S.) corn is cultivated throughout the Midwest due to these favorable conditions that offer high yields per acre which then allows the subsequent corn grain to be used in fermentation processes to generate ethanol for biofuel applications (Rausch et al., 2019). Corn cultivation also produces corn stover that can be used as a dedicated bioenergy crop. Other bioenergy crops such as switchgrass and wheat straw are grown in high yields in the U.S. due to similar favorable growing conditions (Dai et al., 2016; Jesus et al., 2016). The vast geographic size and variable weather conditions in the U.S. are also suitable for producing less valorized crops such as sweet sorghum.
In 2023, the U.S. produced approximately 5.3 million acres of sweet sorghum valued at over $1.2 billion and projections for 2024-2025 range between 279 and 373 million bushels (Ates et al., 2024). Sweet sorghum bagasse (SSB) is an attractive biomass for second-generation fuel ethanol production given its high fermentable sugar content, high biomass yields, and availability (Zhang et al., 2018; Nunta et al., 2023). Moreover, sweet sorghum is a versatile feedstock capable of enduring different environments and easily convertible into a variety of value-added products such as fuel, fertilizers, and food products (Nghiem and Toht, 2019; Lo et al., 2020; Hu et al., 2022). To efficiently extract lignin-derived aromatics and polysaccharide-derived sugars from the biomass, a variety of biofuel, mechanical, chemical, and thermal pretreatment methods are employed to promote cleavage of molecular bonds. This fractionation increases surface area, thus enabling access to polysaccharides during enzymatic hydrolysis to release sugars for fermentation (Venturin et al., 2018; Bhatia et al., 2020; Li et al., 2022; Hamadou et al., 2023). The general composition of SSB consists of 35%–50% cellulose, 25%–35% hemicellulose, and 20%–30% lignin (Mamma et al., 1995; Piskorz et al., 1998). Previous work presented SSB compositions of 32.3% cellulose, 21.2% hemicellulose, and 8.3% lignin (Dar et al., 2018). Moreover, the composition of sweet sorghum on a dry basis has been measured to be 36% total sugar content, 14%–16% lignin, 3.2%–4% ash, and 46%–48% combined cellulose and hemicellulose. While on a sugar-free basis results were 21.9%–25% lignin, 5%–6.3% ash, and 71.9%–75% combined cellulose and hemicellulose (Piskorz et al., 1998). The composition and sugar yields of sweet sorghum biomass have been previously studied using different parts of the plant and its juice (Kim and Day, 2011; Goshadrou et al., 2011; Yue et al., 2021). For example, sorghum stalks were reported to contain 31%–36.8% cellulose, 22%–29.7% hemicellulose, and 13.9%–22% lignin (Kamireddy et al., 2013; Deshavath et al., 2022). Additional results have shown sorghum stalk juice with a sugar content of 16%–28.1%, able to produce 40–86 L
Current key challenges for biomass valorization include identifying efficient biomass-specific pretreatment methods, developing technologies for processing lignocellulose and lignin-derived products, and reducing fermentative inhibitors from pretreated biomass (Zhao et al., 2022). The choice of pretreatment chemistry plays a significant role in determining process performance. Acidic pretreatments function well by disrupting the chemical bonds between plant cell wall components by hydrolyzing polysaccharide fractions, but degradation by dehydration to sugar monomers can easily occur at too high of processing severity (Junior et al., 2020). Prior research using dilute acid pretreatment on SSB could recover up to 100 g L−1 of monomeric sugars following enzymatic hydrolysis, although the byproduct formation of acetic acid and furfural are toxic inhibitors to most microbial fermentation processes (Qureshi et al., 2018). Ammonia pretreatments, which fall under alkali chemistry, are also effective on lignocellulose and function by re-orienting the surface structures of the plant cell wall instead of extracting components (Li et al., 2010; Yang and Rosentrater, 2017). Pretreatments that function by extracting and fractionating plant cell wall components are mostly applied using other types of alkaline chemistry or organosolv. Typically, alkaline pretreatments using sodium hydroxide (NaOH) are performed at high temperatures because it helps activate peeling reactions that remove hemicellulose components from the plant cell wall (Hutterer et al., 2016). Organosolv pretreatments can be conducted using several water-to-organic solvent combinations and with or without an acid or alkali catalyst, however, the pretreatment goal remains to produce separate streams of relatively pure polysaccharides remaining in the pretreated lignocellulose, and a dissolved stream of extracted lignin (Guragain et al., 2016). Enzymatic digestibility of SSB has been evaluated with different pretreatment techniques and alkaline mixtures (Zhang et al., 2011; Cao et al., 2012). Alkaline pretreatments on SSB have shown NaOH to be an effective low-energy input reagent compared to others as it alters lignin structures, loosening xylan cross-links, and partially removing hemicellulose (Karp et al., 2015; Kim et al., 2016; Loow et al., 2016; Mafa et al., 2020). Samples pretreated with NaOH have been shown to interact with the ester and ether bonds in hemicellulose and lignin, making these functional groups and linkage structures easier to remove (Wang et al., 2020). The efficiency of chemical pretreatment depends on NaOH concentration, treatment time, temperature, and biomass characteristics (Mirahmadi et al., 2010). Additionally, alkaline pretreatment with NaOH and sodium carbonate (
This research aims to further understand the impact of combining NaOH and
2 Materials and methods
2.1 Pretreatment
Sweet sorghum bagasse was obtained from Delta Biorenewables (Memphis, TN, USA) and milled in a Wiley Mill with a screen size of 1 mm. Pretreatment consisted of mixing the SSB in a solution of
2.2 Fourier-transform infrared (FTIR) spectroscopy
FTIR analysis with attenuated total reflectance (ATR) was conducted using a Spectrum 3 spectrometer (from Perkin Elmer, Inc). In ATR mode, infrared radiation has a high refractive index that allows radiation to reflect within the sample based on the specific vibration modes of chemical bonds corresponding to the energy levels of the molecules. Test samples were dried to 55°C for at least 12 h in a convection oven before FTIR analysis. Spectroscopy experiments were performed at room temperature and configured to a frequency range of 650–4,000 cm−1, a resolution of 32 cm−1, and 32 scans. Triplicate spectra were collected for each pretreated SSB sample.
2.3 Scanning electron microscopy (SEM)
Dried and milled biomass samples were mounted on stubs with carbon tape and gold sputter coated for 1 min (EMS150R ES from Electron Microscopy Sciences, Hatfield, PA, USA). Micrographs were collected with a FEI Quanta 200 F scanning electron microscope (Hillsboro, OR, USA), set to an accelerating voltage of 5 kV in high vacuum mode and at 500 times magnification.
2.4 Enzymatic hydrolysis
Hydrolysis experiments were performed for both the initial untreated biomass and the pretreated samples. For each case, 5.1 g of dry biomass was placed in a 250 mL Erlenmeyer flask at 10% (w/v) solid loading in a citric acid buffer with a concentration of 50 mmol L−1 and pH of 5. The enzymes used were Cellic CTec2 (a blend of cellulase,
2.5 Analytical methods
The laboratory analytical procedure from the National Renewable Energy Laboratory (NREL-TP-510-42618) was used to perform composition analysis on the untreated and pretreated biomass before and after enzymatic hydrolysis. After pretreatment, the dried solids were measured in triplicates, while after saccharification, the recovered solids were measured once due to the limited amount of solids recovered.
Sugar analysis was performed on the pretreated biomass samples using an Agilent HPLC Series 1,200. The collected frozen samples were thawed for the analysis and centrifuged to separate the liquid from the solids and then filtered. Calcium carbonate from Ricca Chemical Company (Arlington, TX, USA) was added to neutralize the aliquot and mixed with a vortex for 10 s. After reaching pH neutrality, liquid samples were filtered into HPLC vials with a 0.2 µm filter. A sample of 10 µL was added to a 1.5 mL HPLC vial with an insert from which two samples were set for injection of 5 µL each. The reference used was NREL-TP-510-42618. The column used was Aminex® HPX-87H ion exclusion from Bio-Rad Laboratories (Hercules, CA, USA) operated at 60°C. The solvent used was 0.5 wt%
2.6 Statistical methods
Statistical analyses were applied to determine significant factors between the different biomass pretreatments, composition measurements, and sugar yields. The independent variables consisted of the pretreatment ratios and heat treatment severity, in single
Visual (e.g., histogram, boxplot, Q-Q plot) and statistical (e.g., Shapiro-Wilk) normality tests were applied to biomass composition and sugar concentrations. Although measurements were assumed to belong to normal distributions, inconclusive normality results were attributed to using a limited count of sample measurements (
3 Results and discussion
3.1 Pretreatment
Preteated SSB biomass using varying concentrations of NaOH and
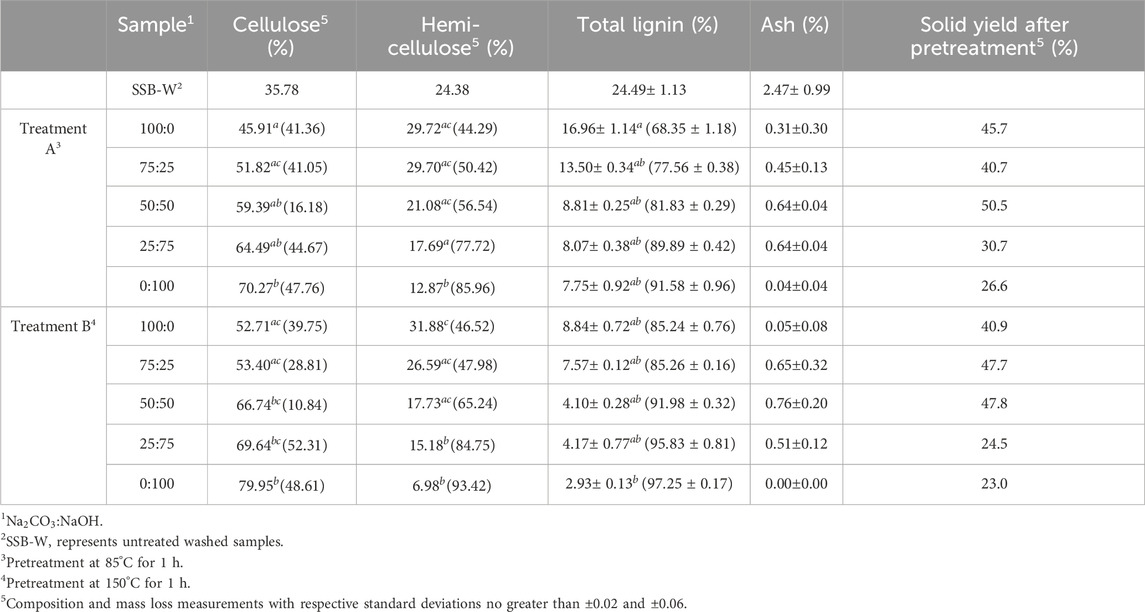
Table 1. Composition and mass balance analysis for untreated and pretreated SSB samples. For each lignocellulosic component, statistical differences (
Table 1 compositions correspond to the recovered solids after biomass pretreatment. The conditions that resulted with the least loss of cellulose content were pretreatments using equal concentrations of NaOH and
Statistical tests suggested there were differences between compositions of the sample and treatment groups. In the multivariate case, cellulose
Previous studies have evaluated different pretreatment strategies on sweet sorghum bagasse. The pretreatment schemes varied in terms of temperature ranges, solvents and concentrations, treatment time, and, in some cases, consisted of multistep approaches (Yu et al., 2011; Cao et al., 2012; Thanapimmetha et al., 2019). Alkaline pretreatments, lime and
3.2 ATR-FTIR spectroscopy analysis
Chemical structures in SSB pretreated biomass were studied using the FTIR spectra shown in Figure 1. The percent of normalized transmittance is presented against wavenumber as a function of different fractions of
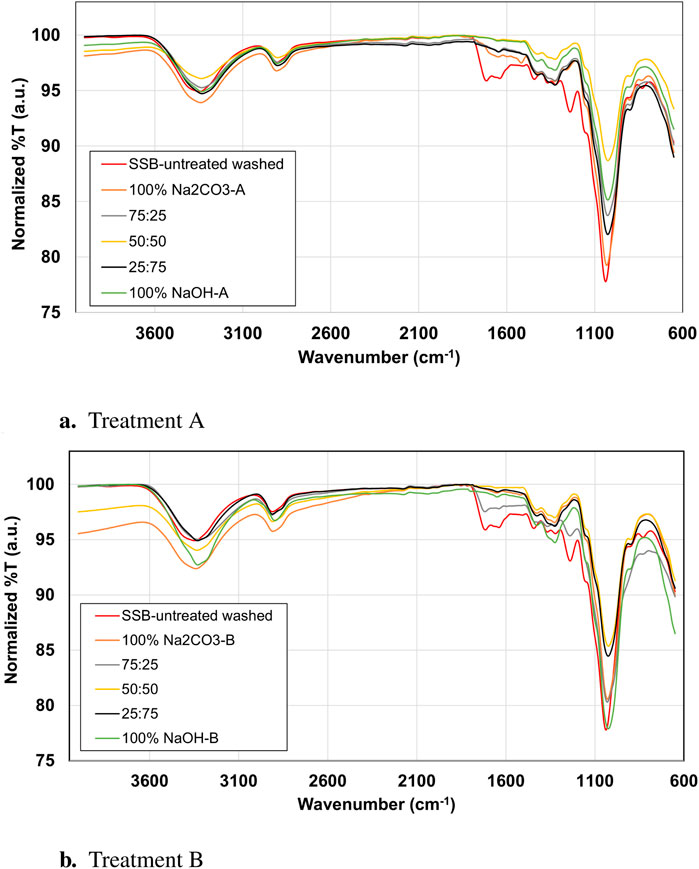
Figure 1. FTIR spectra of SSB samples pretreated with different ratios of
3.3 SEM analysis
SEM was used to study the morphological and textural features present in the untreated and pretreated SSB samples. Figure 2A shows the untreated washed sample as a representative lignocellulosic structure composed of rigid and ordered fibrils along with smooth cell walls containing few longitudinal grooves and having low specific surface areas. Figures 2B–F correspond to SSB samples pretreated with different alkaline mixtures using Treatment B. Pretreated samples showed that lignin was not completely removed but rather exhibited distorted fibers containing rougher surfaces with cracks and pores (Mafa et al., 2020). Hydroxyl groups found in cellulose, hemicellulose, and lignin break down as a consequence of the alkaline pretreatment, thus improving the accessibility to the biomass structure (Beukes and Pletschke, 2010; Jetti and Kishore, 2024). Particularly, samples pretreated with high concentrations of NaOH (
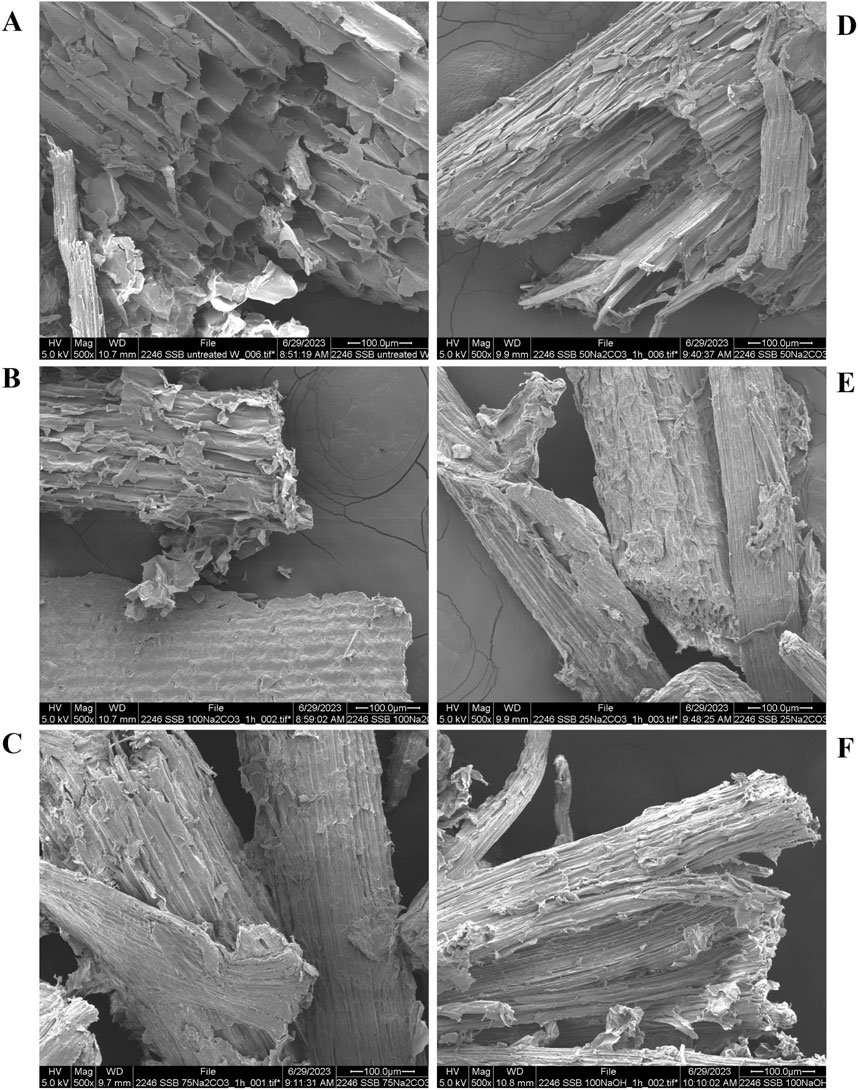
Figure 2. SEM micrographs of SSB samples pretreated with different ratios of
3.4 Enzymatic hydrolysis
Enzymatic hydrolysis was used to assess the saccharification of pretreated SSB biomass. Sugar yields were calculated to evaluate the efficiency of SSB characteristics, pretreatment chemical selection, heat treatment severity, and hydrolysis duration. Figures 3–5 present glucose, xylose, and arabinose yields measured at various times during hydrolysis. A higher yield was achieved for all sugars by the pretreated samples compared to the untreated samples which did not reached a 20% yield. The largest increments in yields occurred during the first 24 h of hydrolysis where samples reached approximately 85% of their maximum yield, indicative of a high concentration of amorphous cellulosic biomass.
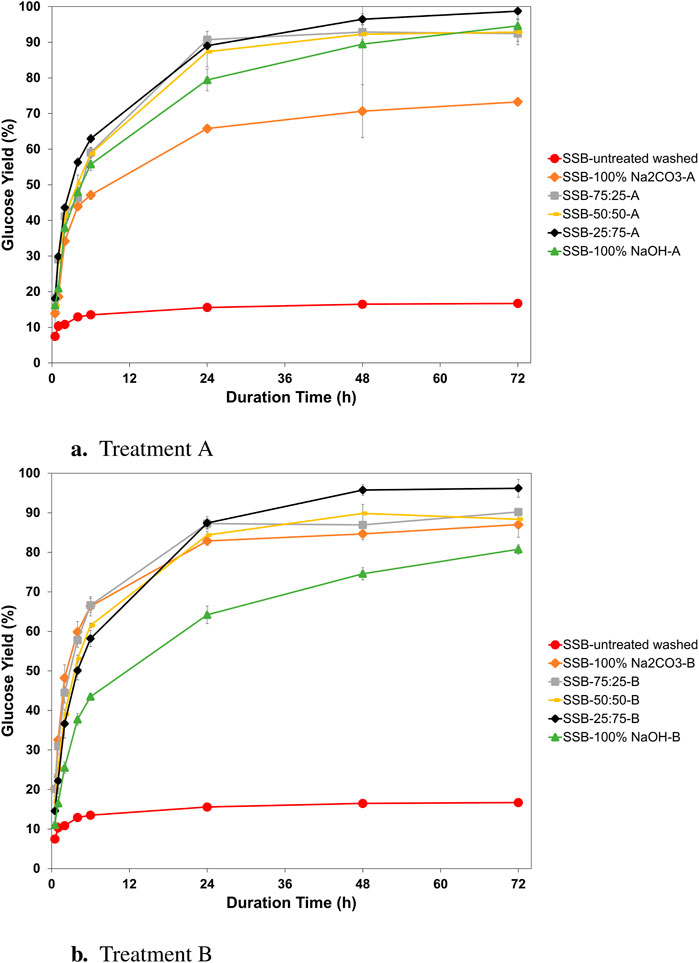
Figure 3. Glucose yields measured at various intervals during enzymatic hydrolysis for SSB samples pretreated with different ratios of
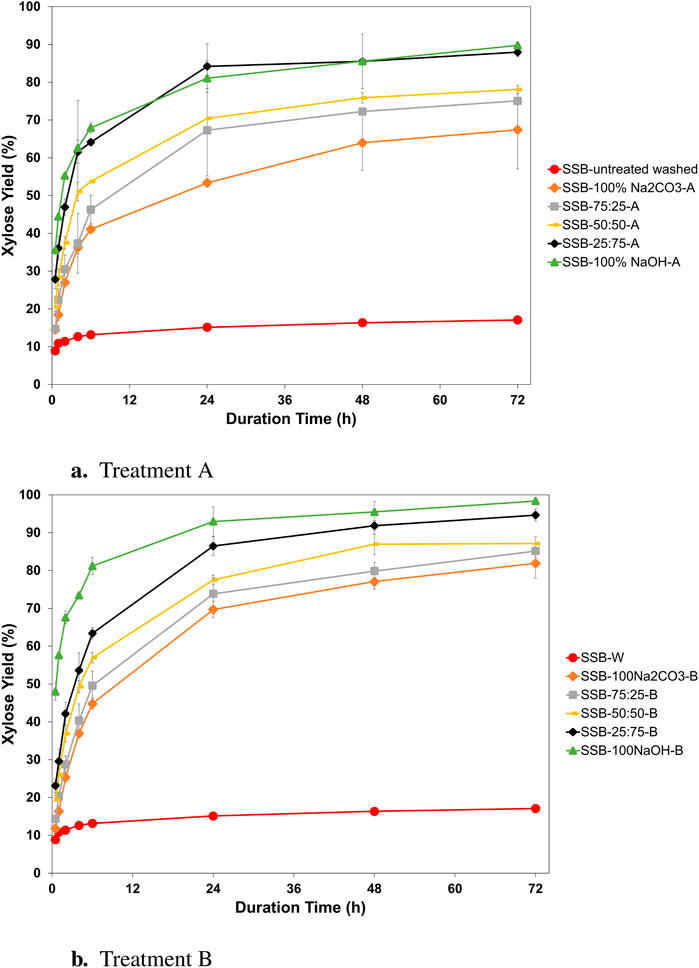
Figure 4. Xylose yields measured at various intervals during enzymatic hydrolysis for SSB samples pretreated with different ratios of
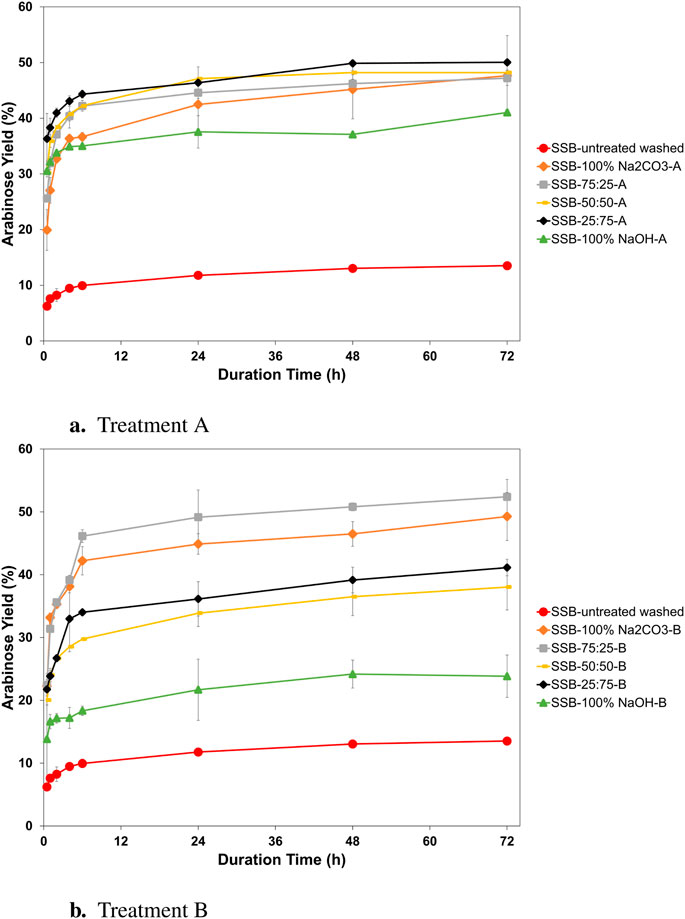
Figure 5. Arabinose yields measured at various intervals during enzymatic hydrolysis for SSB samples pretreated with different ratios of
Glucose yields (Figure 3) were highest when the pretreatment solution included both NaOH and
Statistical correlations indicated significant associations between sugar concentrations (glucose, xylose, arabinose) and pretreatment ratios (0.88, −0.87, −0.82), and low associations with respect to heat treatment (0.38, 0.07, −0.36). Single and multivariate Kruskal–Wallis tests showed significant differences
Sugar yields obtained for the SSB samples are comparable to related works that make use of a variety of pretreatments on sorghum biomass before hydrolysis. SSB enzymatic hydrolysis studies of up to 72 h showed maximum glucose yields of 92% for NaOH pretreatment and 79% for phosphoric acid pretreatment (Goshadrou et al., 2011). A case study evaluated four SSB pretreatment methods (ionic liquid, steam explosion, lime, and dilute acid) where the steam explosion produced a maximum cellulose conversion of 70% (Zhang et al., 2011). Previous work demonstrated SSB pretreatments combining ensiling and NaOH to attain reducing sugar yields ranging in 87%–94% (Zhao et al., 2024). Hydrolysis experiments of washed SSB and forage sorghum using ammonia fiber expansion pretreatment showed high glucan and xylan conversions at 140°C, and up to a 7% increase with xylanase loading. Glucan and xylan conversions were respectively about 80% and 90% for SSB and 80% and 83% for forage sorghum (Li et al., 2010). Dilute sulphuric acid hydrolysis of SSB after different pretreatment severity resulted in up to 82% C5 and 26% C6 sugar yields (Banerji et al., 2013). Note that some of these observations support processing SSB at high-temperature and with NaOH-based pretreatments to obtain high sugar yields during hydrolysis.
Table 2 presents the post-hydrolysis composition analysis of the recovered solids which includes the relative content of polysaccharides and lignin. The remaining contents of the mass balance correspond to other carbohydrates, extractives, hydrocarbons, polyphenolic compounds, sugar acids, and other components. Moderate-to-high temperature alkaline pretreatments helped extract additional phenol derivatives compared to low temperature pretreatments, in addition to acid hydrolysis promoting the release of lignans, low-molecular-weight compounds, and phenolic acids (Yu et al., 2011; Li et al., 2015; Crowe et al., 2019). These effects manifested in the mass balance of the recovered solids where Treatment B samples had more content attributed to cellulose, hemicellulose, and lignin (85% total average) compared to Treatment A (75% total average), given that more components were released during pretreatment. The untreated hydrolyzed samples still consisted of 25% cellulose indicating that enzymes were not able to easily access cellulosic structures. All pretreated hydrolyzed samples consisted mainly of lignin, in most cases over 60%, and low polysaccharide content, less than 20% for cellulose and 4% for hemicellulose. The amount of cellulose and hemicellulose decreased as more NaOH was present in the pretreatment solutions, although hemicellulose’s changes were relatively small. Biomass samples from Treatment B had cellulose content that was approximately half of those from Treatment A. Similarly, samples from Treatment B had less hemicellulose. The total lignin content was greater for mixtures and less variable between them, with the SSB 50:50 samples having the most lignin content which can be associated with the high cellulose-to-lignin content observed in the composition analysis after pretreatment. Moreover, increasing NaOH in the pretreatment made cellulose content in the hydrolyzed solids exhibit a larger difference compared to the content in the pretreated samples, indicating that NaOH was effective at cellulose fractionation and produced improved hydrolyzable structures.
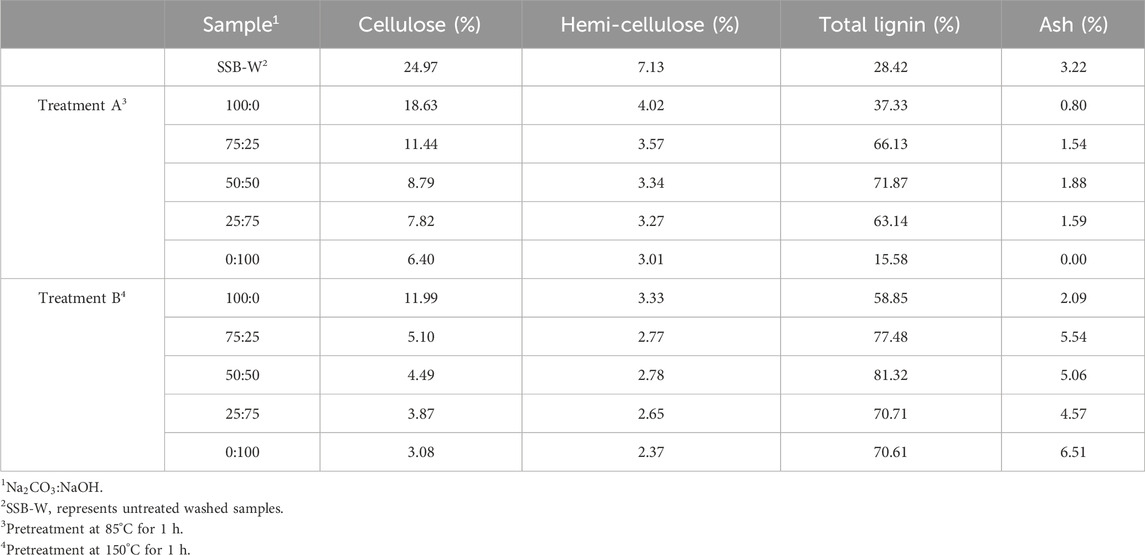
Table 2. Composition analysis for recovered solids from untreated and pretreated SSB samples after hydrolysis.
Glucose conversion efficiency during SSB hydrolysis was evaluated by relating the initial conversion rate as a function of lignin concentration, see Figure 6. Samples exposed to the higher heat treatment severity exhibited increased delignification, with lignin concentrations reduced by 5%–10%, and those with 50% or more NaOH fraction had the lowest lignin concentration (less than 5%). Whereas changes in glucose rates varied differently based on both the heat treatment and pretreatment solution. For example, SSB samples pretreated with equal or more
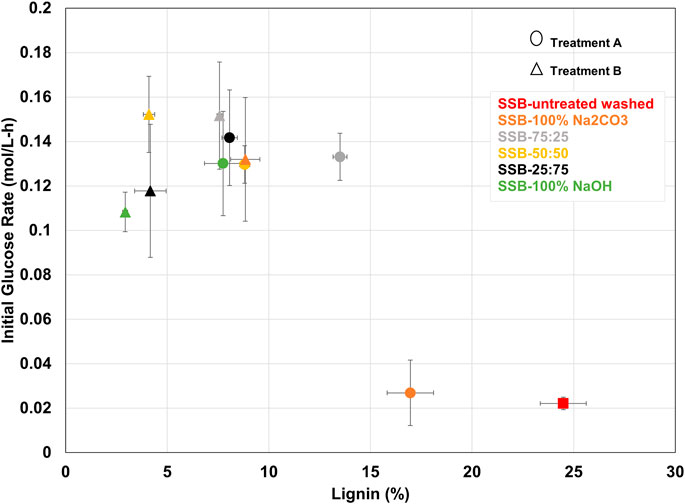
Figure 6. Glucose rates of the first 8 h of hydrolysis as a function of lignin concentration for SSB samples pretreated with different ratios of
4 Conclusion
Sweet sorghum bagasse biomass was used to understand the effects from varying ratios of
The performance of enzyme digestibility of SSB as a function of hydrolysis duration was evaluated by determining the sugar recovery of glucose, xylose, and arabinose via HPLC. Results confirmed pretreatment methods were effective at promoting SSB biomass conversion and increasing sugar recovery after enzymatic hydrolysis. The higher temperature pretreatment, Treatment B, produced additional changes in the chemical structures, thus providing access to more cellulose content and disintegrating more hemicellulose and lignin. Overall, samples pretreated with the conditions of Treatment B showed better stability and sugar release. Pretreatment solutions with
Data availability statement
The original contributions presented in the study are included in the article/supplementary material, further inquiries can be directed to the corresponding author.
Author contributions
VG-N: Conceptualization, Data curation, Investigation, Methodology, Supervision, Writing–original draft, Writing–review and editing, Formal Analysis, Project administration, Validation. RS: Conceptualization, Investigation, Writing–review and editing. MT: Investigation, Methodology, Writing–review and editing.
Funding
The author(s) declare that financial support was received for the research, authorship, and/or publication of this article. This research was supported by USDA’s Agricultural Research Service Project No. 8072-41000-111.
Acknowledgments
The authors want to thank Novozymes for donating the enzymes used in this research work and Joseph Uknalis from the Core Technology unit in our facility for the microscopy support.
Conflict of interest
The authors declare that the research was conducted in the absence of any commercial or financial relationships that could be construed as a potential conflict of interest.
Mention of trade names or commercial products in this article is solely for the purpose of providing scientific information and does not imply recommendation or endorsement by the United States Department of Agriculture (USDA). USDA is an equal employment provider and employer.
Publisher’s note
All claims expressed in this article are solely those of the authors and do not necessarily represent those of their affiliated organizations, or those of the publisher, the editors and the reviewers. Any product that may be evaluated in this article, or claim that may be made by its manufacturer, is not guaranteed or endorsed by the publisher.
References
Ates, A., Marsh, C., and Hutchins, C. (2024). Feed Outlook: August 2024. Tech. Rep. FDS-24h, USDA Econ. Res. Serv.
Banerji, A., Balakrishnan, M., and Kishore, V. (2013). Low severity dilute-acid hydrolysis of sweet sorghum bagasse. Appl. Energy 104, 197–206. doi:10.1016/j.apenergy.2012.11.012
Baral, N. R., Sundstrom, E. R., Das, L., Gladden, J., Eudes, A., Mortimer, J. C., et al. (2019). Approaches for more efficient biological conversion of lignocellulosic feedstocks to biofuels and bioproducts. ACS Sustain. Chem. & Eng. 7, 9062–9079. doi:10.1021/acssuschemeng.9b01229
Beukes, N., and Pletschke, B. I. (2010). Effect of lime pre-treatment on the synergistic hydrolysis of sugarcane bagasse by hemicellulases. Bioresour. Technol. 101, 4472–4478. doi:10.1016/j.biortech.2010.01.081
Bhatia, S. K., Jagtap, S. S., Bedekar, A. A., Bhatia, R. K., Patel, A. K., Pant, D., et al. (2020). Recent developments in pretreatment technologies on lignocellulosic biomass: effect of key parameters, technological improvements, and challenges. Bioresour. Technol. 300, 122724. doi:10.1016/j.biortech.2019.122724
Cao, W., Sun, C., Liu, R., Yin, R., and Wu, X. (2012). Comparison of the effects of five pretreatment methods on enhancing the enzymatic digestibility and ethanol production from sweet sorghum bagasse. Bioresour. Technol. 111, 215–221. doi:10.1016/j.biortech.2012.02.034
Chandel, A. K., Garlapati, V. K., Jeevan Kumar, S., Hans, M., Singh, A. K., and Kumar, S. (2020). The role of renewable chemicals and biofuels in building a bioeconomy. Biofuels, Bioprod. Biorefining 14, 830–844. doi:10.1002/bbb.2104
Clauser, N. M., González, G., Mendieta, C. M., Kruyeniski, J., Area, M. C., and Vallejos, M. E. (2021). Biomass waste as sustainable raw material for energy and fuels. Sustainability 13, 794. doi:10.3390/su13020794
Condon, N., Klemick, H., and Wolverton, A. (2015). Impacts of ethanol policy on corn prices: a review and meta-analysis of recent evidence. Food Policy 51, 63–73. doi:10.1016/j.foodpol.2014.12.007
Crowe, J. D., Li, M., Williams, D. L., Smith, A. D., Liu, T., and Hodge, D. B. (2019). Alkaline and alkaline-oxidative pretreatment and hydrolysis of herbaceous biomass for growth of oleaginous microbes. New York, NY: Springer, 173–182. doi:10.1007/978-1-4939-9484-7_11
Dai, J., Bean, B., Brown, B., Bruening, W., Edwards, J., Flowers, M., et al. (2016). Harvest index and straw yield of five classes of wheat. Biomass Bioenergy 85, 223–227. doi:10.1016/j.biombioe.2015.12.023
Dar, R. A., Dar, E. A., Kaur, A., and Phutela, U. G. (2018). Sweet sorghum–A promising alternative feedstock for biofuel production. Renew. Sustain. Energy Rev. 82, 4070–4090. doi:10.1016/j.rser.2017.10.066
de Jong, W. (2014). Biomass composition, properties, and characterization. Biomass as a Sustain. Energy Source Future Fundam. Convers. Process., 36–68doi. doi:10.1002/9781118916643.ch2
Deshavath, N. N., Mogili, N. V., Dutta, M., Goswami, L., Kushwaha, A., Veeranki, V. D., et al. (2022). “Role of lignocellulosic bioethanol in the transportation sector: limitations and advancements in bioethanol production from lignocellulosic biomass,” in Waste-to-Energy approaches towards zero waste (Elsevier), 57–85. doi:10.1016/B978-0-323-85387-3.00010-0
Ekefre, D. E., Mahapatra, A. K., Latimore Jr, M., Bellmer, D. D., Jena, U., Whitehead, G. J., et al. (2017). Evaluation of three cultivars of sweet sorghum as feedstocks for ethanol production in the Southeast United States. Heliyon 3, e00490. doi:10.1016/j.heliyon.2017.e00490
Gao, D., Haarmeyer, C., Balan, V., Whitehead, T. A., Dale, B. E., and Chundawat, S. P. (2014). Lignin triggers irreversible cellulase loss during pretreated lignocellulosic biomass saccharification. Biotechnol. Biofuels 7, 1–13. doi:10.1186/s13068-014-0175-x
García-Negrón, V., and Toht, M. J. (2022). Corn stover pretreatment with Na2Co3 solution from absorption of recovered CO2. Fermentation 8, 600. doi:10.3390/fermentation8110600
Goshadrou, A., Karimi, K., and Taherzadeh, M. J. (2011). “Improvement of sweet sorghum bagasse hydrolysis by alkali and acidic pretreatments,” in WREC11 World renewable energy Conference 2011 (Linköping, Sweden: Linköping University Electronic Press), 1–7. doi:10.3384/ecp11057374
Guragain, Y. N., Bastola, K. P., Madl, R. L., and Vadlani, P. V. (2016). Novel biomass pretreatment using alkaline organic solvents: a green approach for biomass fractionation and 2, 3-butanediol production. BioEnergy Res. 9, 643–655. doi:10.1007/s12155-015-9706-y
Gustavsson, L., Nguyen, T., Sathre, R., and Tettey, U. Y. A. (2021). Climate effects of forestry and substitution of concrete buildings and fossil energy. Renew. Sustain. Energy Rev. 136, 110435. doi:10.1016/j.rser.2020.110435
Hamadou, B., Djomdi, D., Zieba Falama, R., Djouldé Darnan, R., Audonnet, F., Fontanille, P., et al. (2023). Optimization of energy recovery efficiency from sweet sorghum stems by ethanol and methane fermentation processes coupling. Bioengineered 14, 228–244. doi:10.1080/21655979.2023.2234135
Hu, W., Zhou, L., and Chen, J.-h. (2022). Conversion sweet sorghum biomass to produce value-added products. Biotechnol. Biofuels Bioprod. 15, 72–11. doi:10.1186/s13068-022-02170-6
Huang, C., Jiang, X., Shen, X., Hu, J., Tang, W., Wu, X., et al. (2022). Lignin-enzyme interaction: a roadblock for efficient enzymatic hydrolysis of lignocellulosics. Renew. Sustain. Energy Rev. 154, 111822. doi:10.1016/j.rser.2021.111822
Hutterer, C., Schild, G., and Potthast, A. (2016). A precise study on effects that trigger alkaline hemicellulose extraction efficiency. Bioresour. Technol. 214, 460–467. doi:10.1016/j.biortech.2016.04.114
Jafari, Y., Amiri, H., and Karimi, K. (2016). Acetone pretreatment for improvement of acetone, butanol, and ethanol production from sweet sorghum bagasse. Appl. Energy 168, 216–225. doi:10.1016/j.apenergy.2016.01.090
Jesus, E. d. C., Liang, C., Quensen, J. F., Susilawati, E., Jackson, R. D., Balser, T. C., et al. (2016). Influence of corn, switchgrass, and prairie cropping systems on soil microbial communities in the upper Midwest of the United States. GCB Bioenergy 8, 481–494. doi:10.1111/gcbb.12289
Jetti, K. D., and Kishore, N. S. (2024). Impact of alkali pretreatment methods of bagasse from different sweet sorghum varieties for bioethanol production. Sugar Tech. 26, 562–572. doi:10.1007/s12355-024-01370-8
Jia, F., Chawhuaymak, J., Riley, M. R., Zimmt, W., and Ogden, K. L. (2013). Efficient extraction method to collect sugar from sweet sorghum. J. Biol. Eng. 7, 1–9. doi:10.1186/1754-1611-7-1
Jin, Y., Huang, T., Geng, W., and Yang, L. (2013). Comparison of sodium carbonate pretreatment for enzymatic hydrolysis of wheat straw stem and leaf to produce fermentable sugars. Bioresour. Technol. 137, 294–301. doi:10.1016/j.biortech.2013.03.140
Junior, W. G. M., Pacheco, T. F., Corrêa, P. S., Martins, A. A., Mata, T. M., and Caetano, N. S. (2020). Acid pretreatment of sugarcane biomass to obtain hemicellulosic hydrolisate rich in fermentable sugar. Energy Rep. 6, 18–23. doi:10.1016/j.egyr.2020.10.015
Kamireddy, S. R., Li, J., Abbina, S., Berti, M., Tucker, M., and Ji, Y. (2013). Converting forage sorghum and sunn hemp into biofuels through dilute acid pretreatment. Industrial Crops Prod. 49, 598–609. doi:10.1016/j.indcrop.2013.06.018
Karp, E. M., Resch, M. G., Donohoe, B. S., Ciesielski, P. N., O’Brien, M. H., Nill, J. E., et al. (2015). Alkaline pretreatment of switchgrass. ACS Sustain. Chem. & Eng. 3, 1479–1491. doi:10.1021/acssuschemeng.5b00201
Kim, J. S., Lee, Y., and Kim, T. H. (2016). A review on alkaline pretreatment technology for bioconversion of lignocellulosic biomass. Bioresour. Technol. 199, 42–48. doi:10.1016/j.biortech.2015.08.085
Kim, M., and Day, D. F. (2011). Composition of sugar cane, energy cane, and sweet sorghum suitable for ethanol production at Louisiana sugar mills. J. Industrial Microbiol. Biotechnol. 38, 803–807. doi:10.1007/s10295-010-0812-8
Kim, M., Han, K.-J., Jeong, Y., and Day, D. F. (2012). Utilization of whole sweet sorghum containing juice, leaves, and bagasse for bio-ethanol production. Food Sci. Biotechnol. 21, 1075–1080. doi:10.1007/s10068-012-0139-5
Kotaiah Naik, D., Monika, K., Prabhakar, S., Parthasarathy, R., and Satyavathi, B. (2017). Pyrolysis of sorghum bagasse biomass into bio-char and bio-oil products. J. Therm. Analysis Calorim. 127, 1277–1289. doi:10.1007/s10973-016-6061-y
Li, B.-Z., Balan, V., Yuan, Y.-J., and Dale, B. E. (2010). Process optimization to convert forage and sweet sorghum bagasse to ethanol based on ammonia fiber expansion (AFEX) pretreatment. Bioresour. Technol. 101, 1285–1292. doi:10.1016/j.biortech.2009.09.044
Li, H., Li, Z., Peng, P., She, D., Xu, Q., and Zhang, X. (2015). Characteristics of hemicelluloses obtained from sweet sorghum based on successive extractions. J. Appl. Polym. Sci. 132. doi:10.1002/app.42790
Li, X., Shi, Y., Kong, W., Wei, J., Song, W., and Wang, S. (2022). Improving enzymatic hydrolysis of lignocellulosic biomass by bio-coordinated physicochemical pretreatment: a review. Energy Rep. 8, 696–709. doi:10.1016/j.egyr.2021.12.015
Lo, E., Brabo-Catala, L., Dogaris, I., Ammar, E. M., and Philippidis, G. P. (2020). Biochemical conversion of sweet sorghum bagasse to succinic acid. J. Biosci. Bioeng. 129, 104–109. doi:10.1016/j.jbiosc.2019.07.003
Loow, Y.-L., Wu, T. Y., Md. Jahim, J., Mohammad, A. W., and Teoh, W. H. (2016). Typical conversion of lignocellulosic biomass into reducing sugars using dilute acid hydrolysis and alkaline pretreatment. Cellulose 23, 1491–1520. doi:10.1007/s10570-016-0936-8
Lynd, L. R., Liang, X., Biddy, M. J., Allee, A., Cai, H., Foust, T., et al. (2017). Cellulosic ethanol: status and innovation. Curr. Opin. Biotechnol. 45, 202–211. doi:10.1016/j.copbio.2017.03.008
Mafa, M. S., Malgas, S., Bhattacharya, A., Rashamuse, K., and Pletschke, B. I. (2020). The effects of alkaline pretreatment on agricultural biomasses (corn cob and sweet sorghum bagasse) and their hydrolysis by a termite-derived enzyme cocktail. Agronomy 10, 1211. doi:10.3390/agronomy10081211
Mamma, D., Christakopoulos, P., Koullas, D., Kekos, D., Macris, B., and Koukios, E. (1995). An alternative approach to the bioconversion of sweet sorghum carbohydrates to ethanol. Biomass Bioenergy 8, 99–103. doi:10.1016/0961-9534(95)00006-S
Medina-Martos, E., Gálvez-Martos, J.-L., Almarza, J., Lirio, C., Iribarren, D., Valente, A., and et al., (2022). Environmental and economic performance of carbon capture with sodium hydroxide. J. CO2 Util. 60, 101991. doi:10.1016/j.jcou.2022.101991
Meng, X., and Ragauskas, A. J. (2014). Recent advances in understanding the role of cellulose accessibility in enzymatic hydrolysis of lignocellulosic substrates. Curr. Opin. Biotechnol. 27, 150–158. doi:10.1016/j.copbio.2014.01.014
Mirahmadi, K., Kabir, M. M., Jeihanipour, A., Karimi, K., and Taherzadeh, M. (2010). Alkaline pretreatment of spruce and birch to improve bioethanol and biogas production. BioResources 5, 928–938. doi:10.15376/biores.5.2.928-938
Mirmohamadsadeghi, S., Chen, Z., and Wan, C. (2016). Reducing biomass recalcitrance via mild sodium carbonate pretreatment. Bioresour. Technol. 209, 386–390. doi:10.1016/j.biortech.2016.02.096
Nghiem, N. P., and Toht, M. J. (2019). Pretreatment of sweet sorghum bagasse for ethanol production using Na2CO3 obtained by NaOH absorption of CO2 generated in sweet sorghum juice ethanol fermentation. Fermentation 5, 91. doi:10.3390/fermentation5040091
Nunta, R., Techapun, C., Sommanee, S., Mahakuntha, C., Porninta, K., Punyodom, W., et al. (2023). Valorization of rice straw, sugarcane bagasse and sweet sorghum bagasse for the production of bioethanol and phenylacetylcarbinol. Sci. Rep. 13, 727. doi:10.1038/s41598-023-27451-4
Paris, A. (2018). On the link between oil and agricultural commodity prices: do biofuels matter? Int. Econ. 155, 48–60. doi:10.1016/j.inteco.2017.12.003
Perrone, O. M., de Souza Moretti, M. M., Bordignon, S. E., de Cassia Pereira, J., da Silva, R., Gomes, E., et al. (2021). Improving cellulosic ethanol production using ozonolysis and acid as a sugarcane biomass pretreatment in mild conditions. Bioresour. Technol. Rep. 13, 100628. doi:10.1016/j.biteb.2021.100628
Piskorz, J., Majerski, P., Radlein, D., Scott, D. S., and Bridgwater, A. (1998). Fast pyrolysis of sweet sorghum and sweet sorghum bagasse. J. Anal. Appl. Pyrolysis 46, 15–29. doi:10.1016/S0165-2370(98)00067-9
Qureshi, N., Saha, B. C., Klasson, K. T., and Liu, S. (2018). Butanol production from sweet sorghum bagasse with high solids content: Part I–Comparison of liquid hot water pretreatment with dilute sulfuric acid. Biotechnol. Prog. 34, 960–966. doi:10.1002/btpr.2639
Rausch, K. D., Hummel, D., Johnson, L. A., and May, J. B. (2019). Wet milling: the basis for corn biorefineries. Corn. (Elsevier), 501–535. doi:10.1016/B978-0-12-811971-6.00018-8
R Core Team (2024). R: a language and environment for statistical computing. Vienna, Austria: R Foundation for Statistical Computing.
Saini, J. K., Anurag, R. K., Arya, A., Kumbhar, B., and Tewari, L. (2013). Optimization of saccharification of sweet sorghum bagasse using response surface methodology. Industrial Crops Prod. 44, 211–219. doi:10.1016/j.indcrop.2012.11.011
Sehume, T. Z., Strydom, C. A., Bunt, J. R., and Schobert, H. H. (2020). Bio-oil production from sweet sorghum bagasse via liquefaction using alkaline solutions and identification of phenolic products. Waste biomass valorization 11, 3593–3607. doi:10.1007/s12649-019-00893-6
Thanapimmetha, A., Saisriyoot, M., Khomlaem, C., Chisti, Y., and Srinophakun, P. (2019). A comparison of methods of ethanol production from sweet sorghum bagasse. Biochem. Eng. J. 151, 107352. doi:10.1016/j.bej.2019.107352
Tye, Y. Y., Lee, K. T., Abdullah, W. N. W., and Leh, C. P. (2016). The world availability of non-wood lignocellulosic biomass for the production of cellulosic ethanol and potential pretreatments for the enhancement of enzymatic saccharification. Renew. Sustain. Energy Rev. 60, 155–172. doi:10.1016/j.rser.2016.01.072
Velasco, D., Senit, J. J., De la Torre, I., Santos, T. M., Yustos, P., Santos, V. E., et al. (2017). Optimization of the enzymatic saccharification process of milled orange wastes. Fermentation 3, 37. doi:10.3390/fermentation3030037
Venturin, B., Camargo, A. F., Scapini, T., Mulinari, J., Bonatto, C., Bazoti, S., et al. (2018). Effect of pretreatments on corn stalk chemical properties for biogas production purposes. Bioresour. Technol. 266, 116–124. doi:10.1016/j.biortech.2018.06.069
Wang, M., Wang, J., Li, Y., Li, Q., Li, P., Luo, L., et al. (2022). Low-temperature pretreatment of biomass for enhancing biogas production: a review. Fermentation 8, 562. doi:10.3390/fermentation8100562
Wang, W., Wang, X., Zhang, Y., Yu, Q., Tan, X., Zhuang, X., et al. (2020). Effect of sodium hydroxide pretreatment on physicochemical changes and enzymatic hydrolysis of herbaceous and woody lignocelluloses. Industrial Crops Prod. 145, 112145. doi:10.1016/j.indcrop.2020.112145
Yang, M., and Rosentrater, K. A. (2017). Small-scale low-moisture anhydrous ammonia (LMAA) pretreatment of corn stover. Biomass Bioenergy 97, 38–42. doi:10.1016/j.biombioe.2016.12.013
Yu, Q., Zhuang, X., Yuan, Z., Qi, W., Wang, Q., and Tan, X. (2011). The effect of metal salts on the decomposition of sweet sorghum bagasse in flow-through liquid hot water. Bioresour. Technol. 102, 3445–3450. doi:10.1016/j.biortech.2010.10.084
Yuan, Y., Jiang, B., Chen, H., Wu, W., Wu, S., Jin, Y., et al. (2021). Recent advances in understanding the effects of lignin structural characteristics on enzymatic hydrolysis. Biotechnol. Biofuels 14, 205–220. doi:10.1186/s13068-021-02054-1
Yue, M.-Q., Wang, Z., Dun, B.-Q., Han, F.-X., and Li, G.-Y. (2021). Simplified methods of estimating fermentable sugar yield in sweet sorghum [Sorghum bicolor (L.) Moench] stems. Industrial Crops Prod. 169, 113652. doi:10.1016/j.indcrop.2021.113652
Zhang, C., Wen, H., Zheng, J., Fu, C., Chen, C., Cai, D., et al. (2018). A combination of evaporation and chemical preservation for long-term storage of fresh sweet sorghum juice and subsequent bioethanol production. J. Food Process. Preserv. 42, e13825. doi:10.1111/jfpp.13825
Zhang, H., Han, L., and Dong, H. (2021). An insight to pretreatment, enzyme adsorption and enzymatic hydrolysis of lignocellulosic biomass: experimental and modeling studies. Renew. Sustain. Energy Rev. 140, 110758. doi:10.1016/j.rser.2021.110758
Zhang, J., Ma, X., Yu, J., Zhang, X., and Tan, T. (2011). The effects of four different pretreatments on enzymatic hydrolysis of sweet sorghum bagasse. Bioresour. Technol. 102, 4585–4589. doi:10.1016/j.biortech.2010.12.093
Zhao, L., Sun, Z.-F., Zhang, C.-C., Nan, J., Ren, N.-Q., Lee, D.-J., et al. (2022). Advances in pretreatment of lignocellulosic biomass for bioenergy production: challenges and perspectives. Bioresour. Technol. 343, 126123. doi:10.1016/j.biortech.2021.126123
Zhao, S., Li, H., Sumpradit, T., Khan, A., and Salama, E.-S. (2024). Enhancing biomass conservation and enzymatic hydrolysis of sweet sorghum bagasse by combining pretreatment with ensiling and NaOH. Front. Microbiol. 15, 1370686. doi:10.3389/fmicb.2024.1370686
Keywords: sweet sorghum bagasse, biomass pretreatment, NaOH, Na2CO3, enzymatic hydrolysis, sugar yields
Citation: García-Negrón V, Stoklosa RJ and Toht MJ (2024) Effects of NaOH and Na2CO3 pretreatment on the saccharification of sweet sorghum bagasse. Front. Chem. Eng. 6:1449114. doi: 10.3389/fceng.2024.1449114
Received: 14 June 2024; Accepted: 14 October 2024;
Published: 29 October 2024.
Edited by:
Sameh Samir Ali, Jiangsu University, ChinaReviewed by:
André M. Da Costa Lopes, University of Aveiro, PortugalXin Li, Nanjing Forestry University, China
Mpho Mafa, University of the Free State, South Africa
Copyright © 2024 García-Negrón, Stoklosa and Toht. This is an open-access article distributed under the terms of the Creative Commons Attribution License (CC BY). The use, distribution or reproduction in other forums is permitted, provided the original author(s) and the copyright owner(s) are credited and that the original publication in this journal is cited, in accordance with accepted academic practice. No use, distribution or reproduction is permitted which does not comply with these terms.
*Correspondence: Valerie García-Negrón, dmFsZXJpZS5nYXJjaWFuZWdyb25AdXNkYS5nb3Y=