- Materials and Chemistry (MATCH), Vlaamse Instelling voor Technologisch Onderzoek (VITO NV), Mol, Belgium
Chiral amines are very valued constituents of many important pharmaceutical compounds and their intermediates. However, the production of a chiral amine encounters some challenges, like the use of harsh conditions and the unfavorable thermodynamic equilibrium. In this research the possibilities of tight membrane extraction (ME) for amines separation has been investigated to improve the reaction equilibrium. A specific transaminase reaction was selected for the study in which product amine 1-methyl-3-phenylpropylamine (MPPA) or methyl benzylamine (MBA) needs to be separated from the donor amine isopropylamine (IPA). Tight ME is an innovative separation process in which the membrane is not only an interface, but also a way to add extra selectivity to the process. In the present work, we thoroughly discuss the main factors influencing this novel technique by evaluating the extraction efficiency and extraction rates for the different amines. Then we also determine the optimal parameters for the selected reaction. Supported liquid membrane extraction (SLM), as well as pressure driven filtration, more specifically, nanofiltration (NF), were also studied as benchmark technologies, showing that tight ME has a greater advantage over the two in this specific case, due to the extra affinity factor offered by the membrane. The selectivity of MPPA/IPA in tight ME for the optimized parameters was significantly higher than for SLM and NF.
1 Introduction
Chiral amines are valuable building blocks for pharmaceutical drugs and agrochemicals. As stated by Patil et al. (2018), almost 40% of currently used pharmaceuticals contain chiral amine functional groups in their structure. Therefore, sustainable, low waste and highly efficient synthesis of chiral amines is one of the top priorities for pharmaceutical industry. Although production is possible via both chemical and biocatalytic way and a number of different enzymes are already employed, biocatalytic transamination is considered the most promising synthesis route (Tufvesson et al., 2011). As can be seen in Figure 1, a transaminase enzyme catalyzes the transfer of an amine group from an amine donor (typically an amino acid or a simple amine) to a pro-chiral ketone, yielding a chiral amine and a co-product ketone (Rehn et al., 2014).
The use of transaminases has several advantages including high stereoselectivity and the ability to work under mild conditions. Nevertheless, the reaction is reversible and prone to both substrate and product inhibition. Throughout the last decade, several strategies were developed to overcome these limitations, including supply of an excess of amine donor pushing the reaction to the right and in situ removal of the co-product ketone and/or desired product amine in order to prevent the reverse reaction and avoid enzyme inhibition (Börner et al., 2015). Applying an excess of the donor amine is only sufficient when equilibrium is slightly unfavorable since amine donor solubility has its limitations, and the presence of unreacted amine donor is considered as waste or requires further downstream processing resulting in bad process economics (Tufvesson et al., 2011; Matassa et al., 2019). Therefore, in situ removal of co-product ketone and/or product amine seems to be the better option. Many different in situ product recovery (ISPR) strategies are already studied, including evaporation (Yun et al., 2004), extraction or enzymatic removal of the co-product ketone (Shin et al., 2001), simultaneous removal of product and co-product using ion exchange resins (Han and Shin, 2020) and liquid-liquid extraction (Tufvesson et al., 2011) or membrane separation (Matassa et al., 2019) of the desired product amine. Biggest hurdle for all these strategies is the limited selectivity of the processes since co-product ketones, donor amines and product amines have similar sizes and distribution behavior. An additional drawback of the commonly used liquid-liquid extraction process is the direct contact between solvent and biocatalyst, since many solvents will have a negative impact on the activity of the catalyst. This can be avoided by using a membrane contactor set-up. While in first instance, microporous membranes were used to separate the two phases (Shin et al., 2001; Satyawali et al., 2017), more recently studies were performed on the use of supported liquid membranes (SLM) (Rehn et al., 2014; Börner et al., 2015; Rehn et al., 2016; Van Eygen et al., 2021). SLM typically consists of a microporous membrane impregnated with a hydrophobic liquid and separates the donor phase (reaction mixture) and the acceptor phase. In this type of process, the membrane does not have an active function, it only acts as an interface (Jönsson and Pawliszyn, 2012). By adjusting the pH of donor (alkaline) and acceptor (acidic) phase, Rehn et al. were able to avoid back-extraction and to selectively remove the amines from the reaction mixture, thereby avoiding product inhibition (Rehn et al., 2014; Rehn et al., 2016). However, the extraction selectivity between donor and product amine, was relatively poor due to similar pKa values. In an attempt to avoid simultaneous removal of donor amine, Matassa et al. (2019) replaced the commonly used amine donors isopropylamine (IPA) and alanine by novel high molecular weight (HMW) amine donors allowing separation based on size exclusion mechanism using nanofiltration (NF) membranes. Although promising results were obtained, also several limitations were revealed including the low acceptance of HMW amines by most enzymes and the simultaneous removal of ketones, which have a similar size as the product amines.
In order to combine the avoidance of product inhibition (feasible using SLM extraction) with the selective removal of product amines (feasible by combining HMW and ISPR using NF), in this study an alternative ISPR technology was used, called tight membrane extraction (ME) on a model mixture containing three different amines. Instead of using a dry microporous or SLM, hydrophobic NF membranes were used in a contactor set-up similar to the one used for SLM extraction. By this innovative way of working, the use of an apolar liquid and the associated risk of leaching can be avoided. In this case the membrane is not an interface anymore, but it adds to the selectivity of the process. Membranes and process conditions were varied aiming to find the optimal set of parameters resulting in selective removal of the desired product amines.
Additionally, SLM and NF were studied on the same model mixture with the aim of determining the capabilities and limitations of both technologies and compare the results with tight ME.
2 Materials and methods
2.1 Membrane extraction tests
2.1.1 Preparation of feed and extractant solutions
As feed solution, a model mixture was prepared containing isopropylamine (IPA, ≥99.5%, Sigma-Aldrich) as donor amine and methyl benzylamine (MBA, ≥98.5%, Sigma-Aldrich) and 1-methyl-3-phenylpropylamine (MPPA, ≥97.5%, Sigma-Aldrich) as product amines. Some properties of these compounds can be found in Supplementary Table S1, including Log P, which is one of the most popular scales to measure polarity (Laane et al., 1987). From the Log P values, it is quite clear that MBA and MPPA are both rather apolar amines (MPPA the most apolar), while the donor amine IPA is quite polar. While pKa of the product amine MPPA is similar to the one of donor IPA, the pKa of product amine MBA is significantly lower. By including both product amine, effect of pKa can be studied.
The three amines were present in a concentration of 1 g/L each, unless otherwise stated, and were solved in an alkaline buffer (Na2CO3.10H2O/NaHCO3). A pure acid buffer (C6H8O7.H2O/Na2HPO4) was used as receiving solution at the other side of the membrane. Both buffer solutions were from Sigma-Aldrich.
The Hansen Solubility Parameters (HSP) of the three amines are included in Supplementary Table S2. HSP were developed by Charles M. Hansen in 1967 (Hansen, 1967) as a way of predicting if one material would dissolve in another and form a solution (Hansen, 2007). They are based on the idea that like dissolves like where one molecule is defined as being ”like” another if it bonds to itself in a similar way. The total solubility parameter can be divided up by the three-dimensional solubility parameter, δ (Equation 1):
where δD is the contribution from the dispersion forces, δP is the contribution from the polar forces, and δH is the contribution from the hydrogen forces.
Heptane (≥99% Merck) and undecane (≥98% Merck) were the apolar organic solvents used in some of the tests to impregnate the membranes. HSP of both solvents (Hansen, 2024) are also included in Supplementary Table S2.
2.1.2 Experimental set-up
Tight ME experiments were performed with the set-up presented in Figure 2. The membrane module physically separates the alkaline feed solution and the acidic extractant solution which are both kept in glass bottles with 0.4 L working volume. In order to avoid leakage of the acidic solution into the feed solution, the system was always started by first switching on the feed phase pump. At all times, the feed phase pressure was kept at least 0.1 bar above the extractant phase pressure (in most experiments the over pressure was 200 mbar, unless specified differently). The overpressure is only needed to avoid back diffusion. Extraction time was set at 24 h. Samples of feed and extractant solution were taken at the start and after 1, 3, 6 and 24 h.
Test coupons from the selected membranes were cut from the sheets and placed in a rectangular housing (PS Prozesstechnik GmbH, Switzerland) offering a surface area of approx. 100 cm2.
2.1.3 Test conditions
A whole range of tight ME experiments were performed in order to study the effect of different parameters and to find the optimal set of parameters resulting in selective removal of the desired product amines. Therefore, test conditions were varied one by one. Standard test conditions (based on previous experience with ME) and ranges for the different parameters are summarized in Supplementary Table S3. The standard test was done in triplicate, obtaining quite comparable results (average and standard deviation of the standard test reported in 3.1.
2.1.4 Membranes
Membrane screening experiments were performed at standard conditions using polymeric tight membranes. The membranes had an active surface of 100 cm2. All membranes were commercial and were selected due to their hydrophobicity, to avoid water transport from the alkaline to the acidic solution. An overview of all membranes used and their properties can be found in Supplementary Table S4. The hydrophobicity of the membranes is characterized by their water contact angle. The microporous PTFE membranes were immersed in heptane/undecane and used to compare results of tight ME with SLM extraction using the same extraction set-up. The HSP values of PDMS, POMS and PEBA are included in Supplementary Table S5 (Knozowska et al., 2017, Kujawska et al., 2016).
2.1.5 Sample analysis
MPPA and MBA concentrations in alkaline and acid samples were measured using liquid chromatography (LC). LC is highly effective for separating and analyzing complex mixtures of compounds, especially non-volatile, thermally unstable, or polar substances. Before analysis, the samples were diluted in methanol. The amines were separated on an Acquity UPLC BEH C18 column (50 mm × 2.1 mm; 1.7 µm). The column temperature was kept at 40°C. Optimum separation was obtained with a binary mobile phase constituted of ultrapure water (solvent A) and acetonitrile (solvent B), both solvents acidified with 0.1% formic acid. The flow rate of the mobile phase was 0.4 mL/min. An aliquot of 0.5 µL of the diluted sample was injected into the LC system. The UPLC system was coupled to a PDA (photodiode-array detector) and UV wavelengths of 210 and 245 nm were used for quantification.
IPA concentration in alkaline environment was determined using head-space gas chromatography (HS-GC) coupled with Mass spectrometry (MS). HS-GC/MS is highly effective for analyzing volatile and semi-volatile organic compounds, offering high sensitivity and selectivity. Measuring IPA in acid environment was not possible using LC or HS-GC. Therefore, pH of these samples was adapted to pH 10 using 25% NaOH solution, making HS-GC feasible. pH was adapted immediately after sampling to avoid additional IPA evaporation by reopening the vials.
HS-GC/MS analysis were carried out using a trace GC with a headspace sampler. Injections were made in the split mode onto a HP-VOC column (30 m × 0.2 mm i.d. and 1.12 μm film thickness). The column temperature program was isothermal at 70°C. The injector temperature was set at 250 °C. Helium was used as carrier gas at a constant flow rate of 1 mL min-1 through the column. For every analysis, the purge time was set to 60 s at a purge flow rate of 20 mL min-1 and an equilibration time of 1 min. The column temperature was initially kept at 35°C for 3 min and then increased from 35°C to 120°C at 5°C min-1 and from 120°C to 200°C at 10°C min-1 with a total runtime of 30 min. Helium was used as the carrier gas. Data were acquired in a SIM mode. The injector and transfer line temperatures were 250°C and the split ratio was 25:1. For the quantification of IPA, the ions m/z 44 (quantification ion) and m/z 58 (qualifier ion) were monitored. Triethylamine (TEA) was used as an internal standard.
2.1.6 Membrane extraction performance
The extraction efficiency (EE) expresses the quantity of amines extracted from the alkaline phase into the acid phase and was calculated as follows (Equation 2):
where calkaline is the concentration of the component in the alkaline feed solution after extraction and cacid is the concentration of the component in the acid phase after extraction.
The extraction rate k can be calculated based on the mass balance around the feed reservoir for a given compound (Equation 3):
where V is the volume of the feed solution (L), A is the membrane surface (m2), c is the concentration of the compound in the feed solution at time t and c* is the concentration of the compound in the alkaline feed phase in equilibrium with the acidic phase after extraction (g/L). Integration of this equation leads to Equation 4:
n cases were the extraction equilibrium is reached and extraction efficiency is almost 100%, c* can be assumed to be zero, and equation can be simplified to Equation 5:
Extraction rate k (g/hm2) can be calculated by plotting the left hand site of this equation against time and fitting a straight line to the initial data points (measured before equilibrium was reached), since the slope of this line (-k.A/V) is proportional to the extraction rate k.
The Henderson-Hasselbalch equation was used to calculate the % of charged and uncharged amines at different pHs. The equation relates the pH of a chemical solution of a weak acid to the numerical value of acid dissociation constant and the ratio of the concentrations of the acid and its conjugate base in an equilibrium (Equation 6):
2.2 Nanofiltration
2.2.1 Experimental set-up
The NF screening trials were conducted on a bench-top cross-flow membrane filtration rig (Figure 3). This stainless-steel high-pressure unit allows testing of flat sheet and small tubular membranes on aqueous as well as organic solvent borne mixtures at pressures up to 35 bar and temperatures up to 90°C. It basically consists of a temperature-controlled feed tank (capacity of approx. 1.2 L), a circulation pump (feed flow of max. 800 L/h) and a membrane test cell, and is fitted with a feed flow meter and pressure/temperature transducers. Transmembrane pressure (TMP) is generated by nitrogen gas and the produced permeate is collected in a recipient placed on a balance. The latter is connected to a pc with in-house developed software, hence enabling in-line monitoring of the permeate flux.
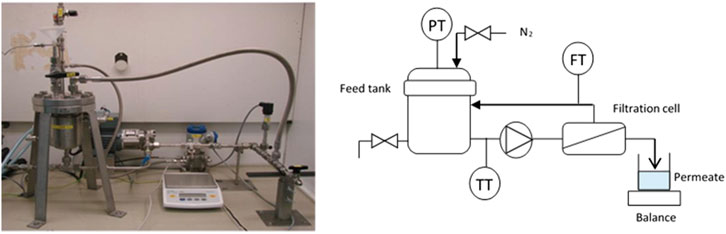
Figure 3. Bench-top cross-flow membrane filtration test unit: Placement under fume hood (left), simplified flow diagram (right).
2.2.2 NF membranes
Five membranes were tested on the model mixture at room temperature. The main characteristics of the selected membranes are described in Supplementary Table S6. The FunMem-phenyl membrane is grafted with Grignard chemistry to make it more hydrophobic compared to the native ceramic one (0.9 nm TiO2) (Buekenhoudt et al., 2010).
For the flat polymeric membranes, test coupons were cut from the sheets and placed in a rectangular housing offering a surface area of approx. 100 cm2 (Figure 2). The ceramic membrane tubes (outer/inner diameter 10/7 mm, length 25 cm, approx. 50 cm2) were placed in a laboratory-made stainless-steel housing, depicted in Figure 4. All membranes were sealed with Kalrez® o-rings.
At the onset of the experimental campaign, the filtration loop and the membrane test cells were thoroughly rinsed with deionized water. Prior to the actual screening tests, the polymeric membranes were subjected to filtration with demineralized water to wash out the preservatives used for dry storage, after which the membranes were kept wet. The ceramic membranes were used without any pretreatment.
2.2.3 Test protocol and conditions
The selected membrane was installed in its housing which was then mounted in the filtration rig using quick connectors, after which approx. 1.2 L of the feed solution was poured into the feed tank and circulated at room temperature. After temperature stabilization, a feed sample was taken. The test solution was pressurized, and point permeate and retentate samples were taken at steady-state conditions. Permeate fluxes, TMPs and temperature were continuously monitored. All the tests were conducted at room temperature, tested pH and TMPs are indicated per membrane in Supplementary Table S7.
For preparing the feed solution at pH 7, the amines were dissolved in a phosphate buffer (Na2HPO4.2H2O/NaH2PO4.H2O) from Sigma-Aldrich. For the experiments at pH 10 and 11 the amines were dissolved in an alkaline buffer as described in Section 2.1.1.
2.2.4 Nanofiltration performance
Performance of the NF membranes was evaluated by calculating fluxes (in L/m2h), defined as the permeate flow per unit of membrane surface area, and retentions (%) which were calculated as follows (Equation 7):
where cp and cr are respectively the concentrations of the amines in permeate and retentate. These concentrations were determined as explained in Section 2.1.5.
3 Results and discussion
3.1 Feasibility of removal of product amines using tight ME
In order to check the feasibility of using tight ME for removal of product amines, a standard test was defined (Supplementary Table S3) and performed with a dry dense polymeric PDMS membrane. Results were used to elucidate the mechanism of this technology.
Within Figure 5, the evolution of the concentration of product and donor amines is plotted as function of time. Both product amines (MBA and MPPA) and donor amine (IPA) moved clearly from the feed to the extractant side within the timeframe of the performed experiment, but with a significant difference in extraction rate. For MPPA and MBA, extraction rates of respectively 10.9 g/hm2 and 6.0 g/hm2 were measured, while for IPA an extraction of 2.2 g/hm2 was obtained.
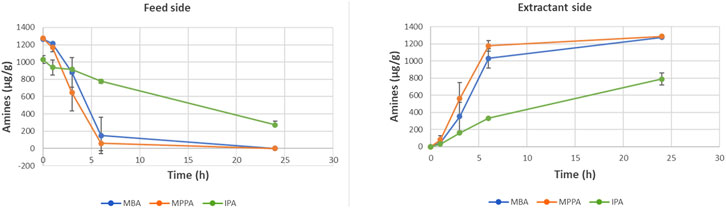
Figure 5. Evolution of concentration of donor and product amines at feed and extractant side (standard experiment with PDMS).
Figure 6 presents the extraction efficiency of the different amines. After 6 h, both product amines were 100% extracted, while the donor amine IPA was only extracted for 30%. For IPA, equilibrium was not reached within the timeframe of the experiment. Most likely, also this compound would be transferred completely in a longer experiment.
The mass balance of all three amines was correct over the 24 h period and as expected based on the hydrophobicity of the used PDMS membrane, no water was transported from feed to extractant side of the membrane or the other way around. The membrane top layer was dry during the full extraction process, only the amines entered from the feed into the PDMS layer (sorption) and traveled through it by diffusion due to their affinity for the membrane material. The amines were released to the extractant phase (desorption), making this process a solution-diffusion process (see Figure 7) (Bozorg, 2019). In such a process, permeants dissolve in the membrane material and then diffuse through the membrane down a concentration gradient. A separation is achieved between different permeants because of differences in the amount of material that dissolves in the membrane and the rate at which the material diffuses through the membrane.
In Figure 8, extraction rates are shown as function of Log P of the different amines. This shows a nice correlation between extraction rate and amine polarity. The most apolar amine (MPPA) was extracted the fastest, and the most polar one (IPA) the slowest. Similar trends can be found with other parameters describing the amine polarity as polar Hansen parameter or hydrogen bonding Hansen parameter, indicating polarity differences lead the selectivity during tight ME. This is a known phenomenon for solvent extraction, where the similarity between solute and extractant polarity determines how easily the solute is transferred to the extractant phase. In this case, however, no solvent is involved, but it seems the membrane is acting as such. This is different from standard ME using porous membranes where the membrane adds no selectivity to the system. In NF on the other hand, the role of solute-membrane affinity is generally very important (Verliefde et al., 2009).
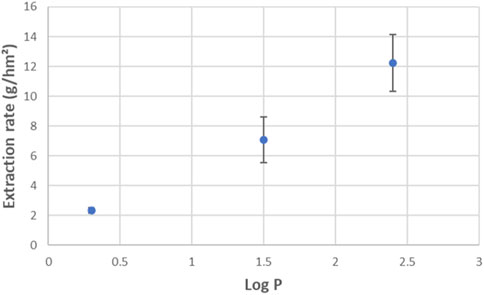
Figure 8. Extraction rates for donor and product amines as function of their Log P values (standard experiment with PDMS).
Besides polarity, also the dissociation state of the amine is known as a parameter affecting extraction efficiency in solvent extraction (Satyawali et al., 2017): charge affects the distribution coefficient, because charge neutrality reduces electrostatic interactions between solute and water and, hence, lowers its aqueous solubility. If the solute is charged, the polarity of the compound greatly increases, therefore, decreasing is affinity with the membrane and decreasing extraction towards a less polar organic solvent. In this case however, the percentage of uncharged amines does not drive the extraction rate, as in that case, IPA and MPPA with about the same pKa would extract at similar rate. The apolar amines were extracted for 100% over 6 h, although at pH 10 only part of these amines are uncharged (according to the Henderson-Hasselbach equation, 19% for MPPA and 74% of the MBA are uncharged). In tight ME, the membrane is acting as a selective barrier and only transport of uncharged amines is possible through the hydrophobic membrane. Since the percentage of uncharged MBA is much higher in the feed, it was expected that this amine would be transferred the fastest, however, this is not the case. Most likely this is because the pH of the feed solution is buffered and extracted amines are trapped in the extractant solution, causing a continuous shift in equilibrium at the feed side (keeping % of uncharged molecules at feed side constant). The amines are trapped in the acidic extractant because charged amines cannot travel through the membrane (no back-extraction). The use of buffer solutions plays a crucial role in this case study, since pH is kept almost constant and, therefore, a new equilibrium is established.
The polar amine IPA was extracted for ∼73% over 24 h. But, for a longer experiment 100% extraction would also be reached for this amine. Again the amount extracted is not related to the amount uncharged, which is ∼16% in this case. Correlated to the transport of amines, the pH of the feed side changed from ∼10.3 to ∼10, and the pH of the extractant side changed from ∼3 to ∼3.4.
From the definition of the extraction rate it can also be deduced how large the ratio between two extraction rates has to be for sufficient purification of a mixture. For example, if a recovery of 90% of the wanted amine product is desired and removal of the unwanted donor amine down to 10% (or 1%) of the original concentration, then the ratio of the 2 extraction rates needs to be >22 (or >230). As it can be seen from Figures 6, 8, in this case the rates are not sufficiently different to reach this target.
3.2 Influence of ME parameters on extraction rate and efficiency
Based on the results of the standard test described in Section 3.1, it seems mass transfer of the amines through the tight membrane into the extractant phase is governed by the solution-diffusion mechanism and the role of the extractant in the process is fulfilled by the membrane. In order to confirm this, a parameter study was performed. A variety of membranes was tested to confirm or deny the role of the membrane chemistry in extraction selectivity and the pH of feed and stripping solution were varied to study the effect of amine charge more thoroughly. Furthermore, also the parameters with a known effect on the solution-diffusion mechanism were varied one-by-one, including flow rates at both side of the membrane, solute concentration at the feed side and feed temperature.
3.2.1 Effect of feed temperature and flow rate
Within Figure 9, effect of feed temperature on extraction efficiency and extraction rate was shown, revealing extraction becomes faster at higher temperature, leading to an extraction efficiency of 100% in 24 h for both the apolar MPPA and MBA and the polar IPA. At 30°C, all extraction rates are 1.5 to more than 2 times higher than the rates measured at 20°C. Consequently, the selectivity does not really change. Higher extraction rates are most likely due to faster diffusion; molecules move faster at higher temperatures.
Also increasing feed flow rate had a positive impact on the extraction rates. As can be seen in Figure 10, especially the extraction rates of the apolar amines are strongly improved. At higher flow rate, the evolution of the extraction rates is linearly dependent on Log P. For comparison we also include in this figure the extraction rates at 30°C,. For MPPA and MBA the effect of temperature is much less, while for IPA at 30°C gives higher rates than the flow rate increase.
This strong influence of the flow rate on the fastest extracting amines, might point to the fact that, without sufficient flow at feed and/or extractant side, the fastest amines start to deplete near the membrane feed surface due to their fast sorption and diffusion, or they start to concentrate near the membrane extractant surface due to their fast desorption. The high flow rates help to supply or remove sufficient amines, avoiding the depletion/concentration at the feed/extractant side of the fast extracting amines. This depletion/concentration effect is logically much less for the slowest extracting IPA.
From the obtained results it is unclear if the flow rate at the feed side or at the extractant side has the largest effect. Also the influence of the relative high TMP or the temperature on the results is unknown. Therefore, some extra tests were performed with the following conditions:
• PDMS 20°C 40 L/h at feed side, 20 L/h at extractant side, ∼200 mbar TMP
• PDMS 20°C 40 L/h both sides, ∼100 mbar TMP
• PDMS 30°C 40 L/h both sides, ∼850 mbar TMP
Figure 11 shows the extraction rates of the 3 amines as function of their Log P, for all measured situations of flow, temperature and TMP. From these results it is clear that TMP has no big influence (result of 40 L/h 20°C with low or high TMP very similar). The low flow at the extractant side, also has a relative low effect (result of 40/40 or 40/20 L/h 20°C very similar). In other words, it is especially the flow rate at the feed side that leads to the higher extraction rates, and thus, it is especially the depletion at the feed side that leads to slower extraction of the fastest amines. The temperature of 30°C has a further positive effect on the extraction rates. With this high temperature, flow rates of 40 L/h seem to be also sufficient to avoid depletion effects.
It is also important to remark that for all conditions with 40 L/h at the feed side, the MPPA extraction rate is about 10 times higher than the IPA extraction rate, also at 30°C. For the 20 L/h conditions, the ratio of the 2 rates is only about 4. In other words, a sufficiently high flow rate at the feed side clearly increases not only the extraction rates, but also the selectivity. Remark that this ratio of 10 between MPPA and IPA is still not high enough to obtain 90% recovery of MPPA combined with the removal of IPA down to 10% (for this a ratio >22 is needed).
3.2.2 Effect of polymer membrane chemistry
Since solute-membrane affinity seemed to be one of the determining parameters for process selectivity, the standard test was repeated with polymeric membranes with other chemistry: next to PDMS, also POMS and PEBA membranes were tested. Figure 12 shows the extraction efficiencies obtained with the different membrane materials, showing highest extraction rates for the POMS membrane and lowest for the PEBA membrane.
An extra PDMS membrane was also tested, but from a different supplier, oNF1 (Borsig). The results of this membrane are compared with the other PDMS membrane and the POMS at different flow rates as function of the log P (Figure 13). The POMS rates at 20 L/h are higher than the PDMS rates at the same flow, and they evolve linearly with the amine polarity (log P). This might mean that the incorporation of the amines in the POMS membranes i.e., sorption/desorption is slower than for PDMS, and, therefore, does not lead to depletion effects as we see for the PDMS membranes at the same flow rate. This conclusion is confirmed by the test performed with the POMS membrane at 40 L/h, showing virtually no change in the extraction rates.
However, the rates for the PDMS membranes at 40 L/h are higher than for the ones of POMS at 20 L/h, at least for the apolar MPPA and MBA. This is not the case for IPA, making the selectivity of the POMS membrane lower than for PDMS. At this point, the reason for this lower selectivity is unclear.
For the oNF1 membrane at 40 L/h the desired ratio MPPA/IPA >22 is already met (43).
We also tested the performance of a PDMS membrane on tubular alumina support (supplier Pervatech), using the same amine mixture at pH 10°C and 30°C. Using high flow rates of 60 L/h and an metallic insert in the membrane to further increase the cross-flow velocity at feed side, the following extraction rates (in g/hm2) were measured: 7.8 for MMA, 3.5 for MBA and 1.9 for IPA. Remark that the use of high feed flow rates is important to get good behavior for this ceramic supported membrane.
3.2.3 Effect of pH at feed side
In order to elucidate the effect of dissociation state of the amine further, experiments were performed using the same alkaline feed buffer as in the standard experiment, but with different pH values varying from 8.9 till 11. The extraction efficiencies show that there is a clear impact of the buffer feed pH, especially for the most polar amine IPA. Figure 14 shows that the highest pH leads to the fastest extraction. For the apolar MPPA and MBA, the effect is similar, but much less (only MPPA is shown, but results for MBA are very similar). As a consequence, feed pH decrease is an elegant way to increase the selectivity. This effect of pH and amount of uncharged amines, comes on top of the influence of the polarity.
The IPA extraction rates confirm the strong pH dependence: extraction rates evolve from 0.54 g/hm2 at pH 8.5–8.9 g/hm2 at pH 11. Most likely this strong influence of feed pH is caused by the changing amount of uncharged amines with pH, as can be calculated from the Henderson-Hasselbalch equation. The deduced evolution of uncharged IPA as function of pH (using pK = 10.73 for IPA) is shown in Figure 15 (left). These results lead to Figure 15 (right) that plots the IPA extraction rates as function of the percentage of uncharged IPA (as calculated for the feed pH after addition of the amines). A near linear dependency can be observed, with a clearly higher extraction rate for conditions with higher percentage of uncharged IPA.
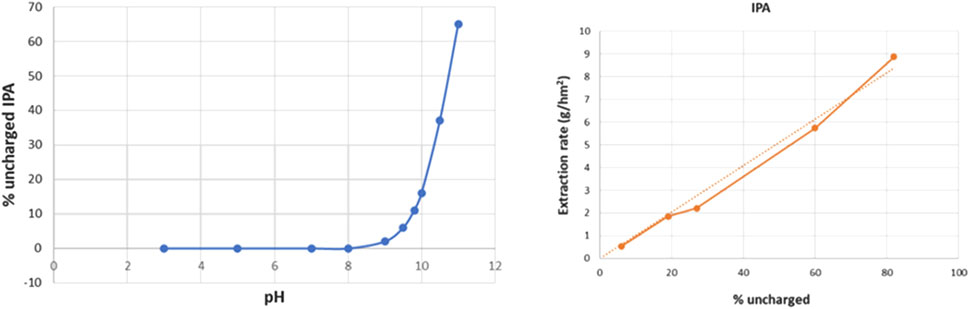
Figure 15. Percentage of uncharged IPA as function of pH (left); IPA extraction rate as function of % uncharged IPA (right).
3.2.4 Effect of pH at extractant side
Experiments were also performed varying the pH of the acid buffer at the extractant side. When pH 5 was used instead of pH 3, there was no significant effect on the IPA extraction rate, but extraction of apolar MPPA and MBA was slowed down a bit (Figure 16). According to Figure 15 (left) about 100% of the IPA amines are charged (and all other amines too) in all situations with extractant pH ranging from 3 up to 8. However, it is to be expected that the thermodynamic equilibrium at the extractant side of the membrane favors more the desorption in case of pH 3, explaining the results. Moreover, the tendency to stay in the membrane (not desorb) at pH 5 will be bigger for the more apolar amines, explaining the stronger influence for MPPA and MBA.
A totally different picture occurred using an alkaline buffer at pH 10 as extractant solution. At that point, about the same extraction rate was measured for all three amines with a lack of selectivity as consequence. In this situation, the back extraction from extractant to feed phase was expected to be similar to the extraction. There is no thermodynamic incentive to desorb from the membrane to the extractant. The small transport of amines is caused by the small overpressure at feed side.
3.2.5 Effect of amine concentration
In a real transaminase reaction, the donor amine like IPA is mostly used in excess. Therefore some experiments were also performed using an IPA concentration varying from 0.5 g/L to 2 g/L. Figure 17 shows the extraction efficiencies of MPPA (MBA similar) and IPA. As expected, the efficiency of IPA is not really influenced by its concentration. As a consequence, the IPA extraction rates (in g/hm2) are proportional to the IPA concentration since driving force is related to this concentration. The other amine rates stay about the same, however, it can be remarked that for 2 g/L IPA, the MPPA extracts unexpectedly, relatively slow.
Another experiment was performed with the double concentration of MPPA. Figure 18 shows the extraction rates of the different amines as function of amine Log P. From the results it is clear that IPA and MBA extraction stays about the same, as expected. In principle double MPPA concentration would double the MPPA extraction rate, but we see a bigger increase, a factor 2.5, instead of 2. This might point to the fact that a higher MPPA concentration helps to overcome the possible depletion of MPPA on the feed side due to its fast extraction. Depletion of MPPA in the standard experimental conditions is also confirmed by the effect of higher flow rates, as explained in Section 3.2.1. Depletion is expected to be less of a problem for the slower extracting IPA as is confirmed in Figure 17.
3.3 Comparison with benchmark technologies
Since tight ME seems to combine transport mechanisms of both extraction with SLM and NF, these two well-known technologies were studied and compared with tight ME in this concrete case of chiral amine synthesis.
3.3.1 Extraction with supported liquid membranes (SLM)
An experiment was performed with an open porous PTFE membrane (commercially available from the company GORE, with pores of 0.05 µm according to the supplier) using standard conditions defined in Supplementary Table S3. The methodology and set-up used for this experiment were exactly the same as for tight ME, only that for this experiment, the PTFE membrane was soaked with heptane (soaking overnight). Additionally, it was decided to investigate in parallel the effect of impregnating the PDMS dense membrane with a solvent, as comparison. Therefore, the standard experiment was repeated with the PDMS membrane impregnated with heptane (soaking the membrane overnight). The heptane might enhance the transport of the amines through the membrane. No leakage of heptane was noticed during the test.
Figure 19 shows the extraction rates of donor and product amines for different membrane systems with and without heptane impregnation. For PDMS, heptane impregnation clearly enhances the extraction rate of all amines, but most for the apolar amines, increasing the selectivity. According to (Hansen, 2024) solubility parameters, heptane and PDMS are very much alike (Supplementary Tables S2, S5), leading most likely to swelling of the PDMS membrane due to the heptane. This might increase the diffusion and, thus, the extraction rate of the most apolar amines. On the other hand, it does seem to influence the extraction of the polar IPA much less, due to the lower heptane/PDMS–solute affinity of this amine. The following extraction rates were found: k (MPPA) = 18.8 g/hm2, k (MBA) = 14.8 g/hm2, (IPA) = 2.8 g/hm2.
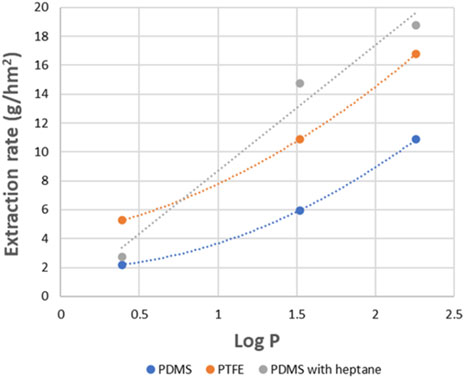
Figure 19. Comparison of extraction rates obtained with PDMS, impregnated PDMS and impregnated PTFE (SLM) for amines with different Log P.
For the PTFE membrane, a relatively high volume transport of about 5.4 mL/h was observed during the experiment, while the TMP was only 100 mbar. It does not seem to be caused by any heptane leakage, nor by damage of the rather thin membranes. Remark that this volume transport corresponds to 5.4 mg/h i.e. 0.54 g/hm2 over this 100 cm2 membrane which is small compared to the total extraction rates observed for the PTFE membrane, and, therefore, it is not expected to influence the conclusions of this experiment. The extraction rates of the PTFE membrane are about a factor 2 higher as those of the dry PDMS membrane. The selectivity for the dry PDMS membrane is somewhat higher, the ratio of MPPA rate versus IPA rate is 4.9 for the PDMS membrane, and 3.2 for the open porous PTFE membrane. This extra selectivity is caused by the dense PDMS membrane itself, since the affinity of the amine for the membrane plays an important role (see Figure 7). In SLM on the other hand, the selectivity is only caused by the heptane immobilized in the membrane pores since adsorption of the more apolar amines from the alkaline feed into the hydrophobic membrane is happening through the solvent present in the membrane, while thermodynamically favorable (Figure 20). Similarly, the desorption of the more apolar amines from the hydrophobic membrane into the acide extractant is expected to occur while again thermodynamically favorable.
The impregnated PDMS combining both mechanisms shows extraction rates of the apolar amines that are somewhat higher than for the heptane filled PTFE membrane, while the extraction rate for the polar IPA is much lower. In other words, the selectivity of the heptane filled PDMS is significantly higher (ratio of MPPA rate versus IPA rate 6.8) than for the heptane filled PTFE membrane. Particularly, the transport of the apolar amines seems to be at least as high in the dense heptane filled membrane, compared to the open porous heptane filled membrane. On the contrary, the difficulty for the more polar IPA to dissolve/adsorb into the dense membrane, slows down its transport, increasing the selectivity of the process.
3.3.2 Nanofiltration (NF)
Since amine-membrane affinity plays also a role in NF, also this technology was tested as alternative for separation of donor and product amines. Table 1 shows the fluxes and amine retentions obtained for a selection of NF membranes at different TMP and pH (depending on pH stability mentioned by the supplier).
The NFS and SE membranes showed quite high retention of the three amines, while the ceramic FunMem-Phenyl membrane had lower retention (45%–55%), well in line with the fact that it is an opener membrane. It is clear that the three amines are retained in a similar extent by each membrane, independent of their polarity and, therefore, it seems like separation is determined by size exclusion and not by affinity. The differences in molecular weight of the different amines does not have an important effect in the separation.
The Duramem membrane showed almost complete retention of the three amines, so again no distinction between the compounds. Fluxes were lower than with previous membranes. Increasing TMP increased flux, but retention remained constant. At pH 7, the percentage of uncharged amines is zero, which can be the reason of having an enhanced amine rejection compared to the NFS and SE membranes.
The 0.9 nm TiO2 membrane was tested at three different pHs, membrane fluxes increased with increasing pH. Retention profiles were similar for MBA and MPPA at pH 7 and 10, while for IPA retention dropped at pH 10 (compared to pH 7). At pH 11, retention of all the amines dropped. At pH 11 the amount of uncharged amines is higher and that seems to be linked to the lower retention of the amines. The isoelectric point of TiO2 is between pH 5 and 6, according to literature and above that pH range, the membrane is negatively charged. Although varying pH seems an interesting parameter for changing amine selectivity, differences between the three amines are not large enough to have a proper and efficient separation process.
Based on the obtained results, it seems in this case separation with NF is purely driven by size exclusion (Figure 21) and affinity is not playing a significant role. All the amines were retained by the different membranes in a similar way, independently of their properties. pH influenced retentions when using a ceramic membrane, but without differentiating between the amines and, therefore, without significant effect on the selectivity.
3.4 Optimized tight membrane extraction
Since highest selectivities were obtained with tight membrane extraction using an impregnated PDMS membrane, this experiment was repeated under optimal conditions presented in Supplementary Table S8.
Obtained extraction efficiencies and extraction rates are summarized in Figure 22; Table 2. After 2.5 h, 90% of MPPA was extracted, while extraction efficiency of IPA was kept down to 2%. Under optimized conditions, extraction rate was 3.4 times higher for MPPA, 4.7 times higher for MBA and 3.7 times lower for IPA.
Table 2 also includes a detailed comparison between the standard and optimized tight ME tests, together with the benchmark technologies. The selectivity for NF has been calculated using the following Equation 8:
where S is selectivity, R, retention, A and B the solutes, in this case MPPA and IPA.
From the table one can see the clear advantage of using tight ME and tight SLM (both with optimized parameters) compared to SLM and, specially, to NF, where the selectivity MPPA/IPA is much lower.
4 Conclusion
The potential of tight ME has been extensively studied for the selective removal of product amines in chiral amine synthesis. The process clearly combines the mechanisms of two technologies resulting in an improved selectivity. Just as mentioned before for NF, polarity and solute-membrane affinity plays a role in selectively removing components, which is different from the standard ME process where the membrane itself adds no selectivity to the process. While in NF size exclusion is still the dominant factor, this is not the case in tight ME, making it possible to separate components with similar size. By impregnating the membrane as in extraction with SLM, extra selectivity of the solvent is added to the selectivity of the membrane. Nevertheless, in some cases where the use of solvent can hinder the process, preference can go towards working solvent free despite lower selectivity.
Transport through the membrane was influenced by different factors as summarized in Table 3. By optimizing these parameters, it was possible to remove 90% of the product amine while extraction of the donor amine was kept below 2%.
In view of this promising results, further research towards the original application of chiral amine synthesis should be conducted. Based on this research, 90% recovery of MPPA from an aqueous solution, combined with the removal of IPA down to 10%, is possible, However, the presence of ketones should be also taken into account. Additionally, a proof-of concept test of ISPR is required towards industrial application, coupled with a techno-economic assessment [such an assessment was already conducted by Yang et al. (2022)] and environmental impact study.
Data availability statement
The raw data supporting the conclusions of this article will be made available by the authors, without undue reservation.
Author contributions
SS: Investigation, Methodology, Validation, Visualization, Writing–review and editing. KD: Conceptualization, Data curation, Investigation, Methodology, Validation, Visualization, Writing–original draft, Writing–review and editing. AB: Conceptualization, Data curation, Funding acquisition, Investigation, Methodology, Project administration, Supervision, Writing–original draft.
Funding
The author(s) declare that financial support was received for the research, authorship, and/or publication of this article. This research was funded by VLAIO, grant number HBC.2018.0484, through the Catalisti cluster cSBO project Easichem.
Conflict of interest
The authors declare that the research was conducted in the absence of any commercial or financial relationships that could be construed as a potential conflict of interest.
Publisher’s note
All claims expressed in this article are solely those of the authors and do not necessarily represent those of their affiliated organizations, or those of the publisher, the editors and the reviewers. Any product that may be evaluated in this article, or claim that may be made by its manufacturer, is not guaranteed or endorsed by the publisher.
Supplementary material
The Supplementary Material for this article can be found online at: https://www.frontiersin.org/articles/10.3389/fceng.2024.1449088/full#supplementary-material
References
Börner, T., Rehn, G., Grey, C., and Adlercreutz, P. (2015). A process concept for high-purity production of amines by transaminase-catalyzed asymmetric synthesis: combining enzyme cascade and membrane-assisted ISPR. Org. Process Res. and Dev. 19 (7), 793–799. doi:10.1021/acs.oprd.5b00055
Bozorg, M. (2019). Optimization of membrane process architecture: Université de Lorraine and Università degli studi di Roma. Università degli studi di Roma “Tor Vergata”: Chemical and Process Engineering, Université de Lorraine.
Buekenhoudt, A., Wyns, K., Meynen, V., Maes, B., and Cool, P. (2010). inventorsSurface-modified inorganic matrix and method for preparation thereof 2010.
Han, S.-W., and Shin, J.-S. (2020). In situ removal of inhibitory products with ion exchange resins for enhanced synthesis of chiral amines using ω-transaminase. Biochem. Eng. J. 162, 107718. doi:10.1016/j.bej.2020.107718
Hansen, C. M. (1967). The three dimensional solubility parameter and solvent diffusion coefficient: their importance in surface coating formulation. Copenhagen: Danish Technical Press.
Hansen, C. M. (2007). “Hansen Solubility Parameters: a user's handbook,” in Boca ratón. Second edition. 2nd Edition (Boca Ratón, Florida: CRC Press), 544.
Hansen, C. M. (2024). HSPiP software. Available at: https://www.hansen-solubility.com/HSPiPn.d.
Jönsson, J. Å. (2012). “2.22 - membrane extraction: general overview and basic techniques,” in Comprehensive sampling and sample preparation. Editor J. Pawliszyn (Oxford: Academic Press), 461–474.
Knozowska, K., Kujawska, A., Kujawa, J., Kujawski, W., Bryjak, M., Chrzanowska, E., et al. (2017). Performance of commercial composite hydrophobic membranes applied forpervaporative reclamation of acetone, butanol, and ethanol from aqueous solutions: binary mixtures. Sep. Purif. Technol. 188, 512–522. doi:10.1016/j.seppur.2017.07.072
Kujawska, A., Knozowska, K., Kujawa, J., and Kujawski, W. (2016). Influence of downstream pressure on pervaporation properties of PDMS and POMS based membranes. Sep. Purif. Technol. 159, 68–80. doi:10.1016/j.seppur.2015.12.057
Laane, C., Boeren, S., Vos, K., and Veeger, C. (1987). Rules for optimization of biocatalysis in organic solvents. Biotechnol. Bioeng. 30 (1), 81–88. doi:10.1002/bit.22209
Matassa, C., Ormerod, D., Bornscheuer, U. T., Höhne, M., and Satyawali, Y. (2019). Application of novel High Molecular Weight amine donors in chiral amine synthesis facilitates integrated downstream processing and provides in situ product recovery opportunities. Process Biochem. 80, 17–25. doi:10.1016/j.procbio.2019.02.018
Patil, M. D., Grogan, G., Bommarius, A., and Yun, H. (2018). Oxidoreductase-Catalyzed synthesis of chiral amines. ACS Catal. 8 (12), 10985–11015. doi:10.1021/acscatal.8b02924
Rehn, G., Adlercreutz, P., and Grey, C. (2014). Supported liquid membrane as a novel tool for driving the equilibrium of ω-transaminase catalyzed asymmetric synthesis. J. Biotechnol. 179, 50–55. doi:10.1016/j.jbiotec.2014.03.022
Rehn, G., Ayres, B., Adlercreutz, P., and Grey, C. (2016). An improved process for biocatalytic asymmetric amine synthesis by in situ product removal using a supported liquid membrane. J. Mol. Catal. B Enzym. 123, 1–7. doi:10.1016/j.molcatb.2015.10.010
Satyawali, Y., Ehimen, E., Cauwenberghs, L., Maesen, M., Vandezande, P., and Dejonghe, W. (2017). Asymmetric synthesis of chiral amine in organic solvent and in-situ product recovery for process intensification: a case study. Biochem. Eng. J. 117, 97–104. doi:10.1016/j.bej.2016.11.006
Shin, J. S., Kim, B. G., Liese, A., and Wandrey, C. (2001). Kinetic resolution of chiral amines with omega-transaminase using an enzyme-membrane reactor. Biotechnol. Bioeng. 73 (3), 179–187. doi:10.1002/bit.1050
Tufvesson, P., Lima-Ramos, J., Jensen, J. S., Al-Haque, N., Neto, W., and Woodley, J. M. (2011). Process considerations for the asymmetric synthesis of chiral amines using transaminases. Biotechnol. Bioeng. 108 (7), 1479–1493. doi:10.1002/bit.23154
Van Eygen, G., Van der Bruggen, B., Buekenhoudt, A., and Luis Alconero, P. (2021). Efficient membrane-based affinity separations for chemical applications: a review. Chem. Eng. Process. - Process Intensif. 169, 108613. doi:10.1016/j.cep.2021.108613
Verliefde, A. R. D., Cornelissen, E. R., Heijman, S. G. J., Hoek, E. M. V., Amy, G. L., Bruggen, B. Vd, et al. (2009). Influence of Solute−Membrane affinity on rejection of uncharged organic solutes by nanofiltration membranes. Environ. Sci. and Technol. 43 (7), 2400–2406. doi:10.1021/es803146r
Yang, J., Buekenhoudt, A., Dael, M. V., Luis, P., Satyawali, Y., Malina, R., et al. (2022). A techno-economic assessment of a biocatalytic chiral amine production process integrated with in situ membrane extraction. Org. Process Res. and Dev. 26 (7), 2052–2066. doi:10.1021/acs.oprd.1c00464
Keywords: tight membrane extraction, chiral amines, affinity separation, process optimization, supported liquid membranes (SLM), nanofiltration (NF)
Citation: Salvador Cob S, De Sitter K and Buekenhoudt A (2024) Application of tight membrane extraction for amines separation. Front. Chem. Eng. 6:1449088. doi: 10.3389/fceng.2024.1449088
Received: 14 June 2024; Accepted: 04 November 2024;
Published: 20 November 2024.
Edited by:
Patricia Luis, Université Catholique de Louvain, BelgiumReviewed by:
Nevena Milčić, University of Zagreb, CroatiaJulien Estager, Centre of Technological Resources in Chemistry (CERTECH), Belgium
Copyright © 2024 Salvador Cob, De Sitter and Buekenhoudt. This is an open-access article distributed under the terms of the Creative Commons Attribution License (CC BY). The use, distribution or reproduction in other forums is permitted, provided the original author(s) and the copyright owner(s) are credited and that the original publication in this journal is cited, in accordance with accepted academic practice. No use, distribution or reproduction is permitted which does not comply with these terms.
*Correspondence: Kristien De Sitter, a3Jpc3RpZW4uZGVzaXR0ZXJAdml0by5iZQ==