- 1Future Industries Institute, UniSA STEM, University of South Australia, Adelaide, SA, Australia
- 2Australian National Fabrication Facility - South Australia Node, University of South Australia, Adelaide, SA, Australia
Microfluidic and lab-on-a-chip devices offer exquisite temporal and spatial control over chemical and physical processes that are important in mineral exploration and mining. These include mineral-water interfacial reactions, dissolution, and adsorption/desorption in pores, fractures, or other micro/nanostructures. Microfluidic mineral studies offer advantages of small sample and reagent volumes, high throughout, and short analytical cycles that may enable in-field mining decisions. However, not many microfluidic studies have targeted these mining sector challenges for mineral leaching. In this review, special attention is given to microscale experimental platforms for predicting extraction and leaching of industrially-relevant samples (real ore samples). Advantages and challenges of these platforms are given. The review concludes that there are significant opportunities for microfluidics in mineral analysis, screening, process intensification, and process control in the resource and minerals sector.
1 Introduction
Microfluidic and lab-on-a-chip technologies offer a potential path to fast, precise, and inexpensive industrial process control and analysis but are not yet widely employed in the resource and minerals sector. Promising research has identified significant technical and practical advantages, such as small sample and reagent volumes, high-resolution analysis, high throughput, enhanced mass and heat transfer, and small footprint for analytical devices (Gerami et al., 2019). The steady increase in research on this topic indicates a growing acceptance of microfluidic and lab-on-a-chip technologies as a strategy for studying resources and minerals. Microfluidic techniques have been increasingly applied to enhanced oil recovery (Gogoi and Gogoi, 2019; Lifton, 2016), heavy oil/bitumen production (Bazazi et al., 2019; Keshmiri et al., 2019), carbon transport and storage (Abolhasani et al., 2014; Bao et al., 2017; Morais et al., 2020), reservoir fluid analysis (Song et al., 2014), mine waste remediation (Yang et al., 2020), high-level radioactive waste disposal (Oh et al., 2017) and other geochemical processes (Ciceri and Allanore, 2015; Gerami et al., 2017; Park et al., 2021; Zhu et al., 2022). The use of microfluidics to study flow and transport in porous media that is relevant to hydrocarbon recovery processes and geological CO2 sequestration has been reviewed extensively (Gerami et al., 2019; Lifton, 2016; Abolhasani et al., 2014; Bao et al., 2017; Morais et al., 2020; Song et al., 2014; Cao et al., 2019; Lei et al., 2020; Yew et al., 2019; Yang et al., 2021). However, its application to studying interfacial chemistry in mineral systems has received less attention.
Microfluidics is primarily used to study the science of fluid flow and transport phenomena in microstructures where pore-scale features (e.g., surface roughness or wettability) influence the macroscopic behavior (e.g., reactivity or transport properties) of the system (Gerami et al., 2019; Bao et al., 2017). Whether naturally occurring or resulting from subsurface engineering, open rock fractures act as preferential flow pathways that typically control fluid migration and solute transport. For this reason, fractures are avoided when siting and designing geological isolation systems, such as for nuclear waste and CO2 storage, to prevent undesired fluid and chemical migration (Abolhasani et al., 2014; Mariet et al., 2019). While in other cases, such as mineral processing, mined ores are inherently structured, porous, or fractured and create micro/nano-scale environments that are chemically reactive and critical to the effectiveness of leach strategies (Yang and Priest, 2018; Yang et al., 2019). Fractures can also be artificially created for enhanced energy recovery, through excess fluid pressure and change of thermal stress, as in the case of geothermal energy extraction and unconventional oil or gas production (Bao et al., 2017). Through integration with current optical tools like optical/confocal microscopy, microfluidic models offer direct analytical access to study or validate the process of geological related pore-scale transport phenomena. They also provide microscopic data for understanding the reactive and/or transport mechanisms leading to mineral precipitation and dissolution in fractures, that are often not achievable with conventional analytical methods (Ciceri and Allanore, 2015). Thus, the approach has been widely used to model or observe fluid-rock interactions that are relevant to several applications as listed above. This is equally crucial to the development of predictive tools that can be applied in mineral processing such as engineered system design and optimization, mineral recovery feasibility studies, and environmental impact assessments.
The scale of traditional environmental samples (e.g., minerals or rocks) requires conventional analytical techniques that are often time- and cost-intensive in sample preparation and analyses, resulting in intermittent and slower detections. In contrast, microfluidics enables the rapid handling and analysis of samples on so-called lab-on-a-chip (LOC) systems (Lim et al., 2010). LOC systems are often favorable for analytical purposes. They require small quantities of samples and reagents, produce minimal waste, reduce sampling time and handling steps, and, in many cases, reduce the cost and complexity of chemical analysis. The capacity to integrate many processing steps on a small and often portable platform may enable fast analytical answers close to a mineral processing site, providing real-time intervention for step-change operational efficiencies. However, at present, LOC systems are underutilized in mineral processing, with many engineering problems still being tackled using conventional centralized observation, diagnostics, and analysis. In contrast, applications of LOC systems are widespread in the biomedical and life sciences, such as DNA and cell assays (Andersson and van den Berg, 2003; Yi et al., 2006; Wang and Li, 2011), and are increasingly applied to environmental monitoring and food industry analysis (Yew et al., 2019; Atalay et al., 2011). New opportunities in automation and rapid online analysis or screening procedures for a variety of different engineering applications including mining industry are a promising way forward. Nonetheless, next-generation process optimization and control approaches will be limited by the speed and accuracy of process-specific chemical information. In this respect, developing LOC systems for mineral processing is increasingly urgent. Figure 1 shows typical mineral processing stages and highlights hydrometallurgy stages that can benefit, or are already benefiting, from microfluidic or LOC strategies: froth flotation, leaching, solvent extraction, and electrowinning or precipitation. Froth flotation separates mineral particles in an aqueous phase slurry based on selective bubble-particle attachment. Bubbles carry hydrophobic particles to the slurry surface to form a froth containing the valuable mineral particles. This “concentrate” of mineral particles is then collected at a froth overflow for downstream processing. Acid leaching (aqueous phase) then digests the mineral concentrate to liberate the targeted metal ions into aqueous solution (leachate). Acid leaching may take hours to months, depending on the ore and specific leaching approach chosen. The leachate contains a mixture of metal ions, which can be isolated by liquid-liquid solvent extraction from the aqueous leachate into an organic phase, aided by an extractant that selectively binds to the metal ion. The metal-extractant species is preferentially soluble in the organic phase, isolating the target metal in that phase. Solvent extraction typically requires many stages of extraction and back-extraction (stripping) to achieve a high purity metal ion aqueous solution for the final step of electrochemical metal plating or precipitation of a high purity metal salt.
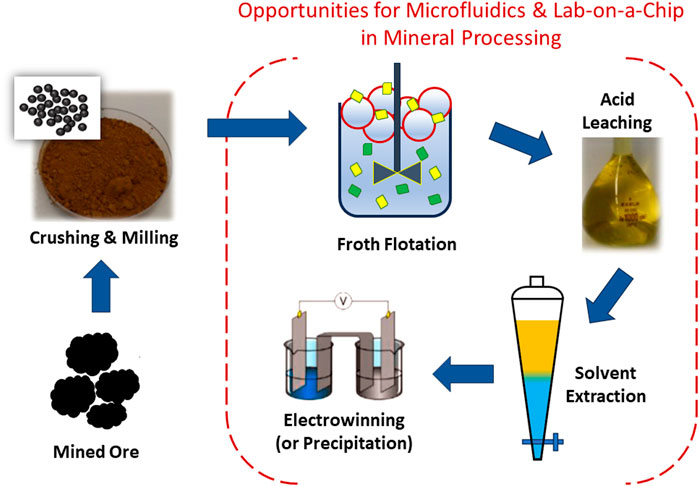
Figure 1. Common process steps in mineral processing and opportunities for microfluidics-based research in hydrometallurgy stages: froth flotation, leaching, solvent extraction, and electrowinning or precipitation. See main text for discussion of these mineral processing steps.
Microfluidic platforms can deliver new insights by (1) obtaining pore-scale phenomenon and mechanisms through visualization, modeling, and comparison with macroscopic results for better understanding of mineral reactivity in porous and fractured porous media; (2) providing in situ real-time information (e.g., dynamics, reaction rates and concentrations) through LOC technologies for process control and optimization of mineral operations; (3) using only a small amount of sample and/or reagent in rapid screening and parallel experiments; and (4) facilitating process intensification through enhanced mass and heat transfer rates. These four attributes are relevant to many mineral processing scenarios (depicted in Figure 1). The majority of studies to date have focused on microfluidics for solvent extraction, due to the high surface-to-volume ratios, mass transfer, and interfacial phenomena.
Microfluidic solvent extraction (microSX) has been demonstrated for many metals, including base (e.g., Cu, Ni) (Priest et al., 2011; Jiang et al., 2018; Zhang et al., 2014), precious (e.g., Au, Ag, Pt) (Yin et al., 2013; Kriel et al., 2015), rare earths (Kolar et al., 2016; Yin et al., 2018), and scarce (e.g., Ga, In) metals (Li et al., 2015; Li et al., 2016). The successful demonstration of small-volume metal extraction and increasing recognition of the potential benefits to the mining industry has encouraged researchers to pursue higher-throughput microSX. These benefits are likely to play a significant role in meeting environmental, societal, and governance (ESG) responsibilities in global ambitions for a green economy (Le et al., 2022). However, it is important to note here that the scale of reactors should be tailored to the specific process requirements. For example, Vural Gürsel et al. (2016) demonstrated that milli-fluidic reactors can be suitable for high volumetric throughputs. In this case, extraction of cobalt was accelerated by running dispersed phase (segmented flow) through coiled milli-scale tubing and online phase separator, cf. Figure 2A. Pilot scale throughputs of 53 m3/y were reported to be possible. Returning to the microscale, high-throughputs require numbering-up strategies. Numbering-up increases volumetric throughput by coupling many parallel processing units, which retain the identical processing dimensions and operational performance. Internal numbering-up occurs through parallel operations within the extractor, while external numbering-up replicates the entire operation in parallel (including pumps and hardware) (Schenk et al., 2003; Stee et al., 2022). Internal numbering-up is favored for hardware efficiency. Numbering-up is distinct from scale-up, where process vessels are scaled to be physically larger and risks changes to reaction/extraction conditions (e.g., mass or heat transfer) that may adversely affect the process outcomes. MicroSX of high-value metals processed at low-volumes is now feasible. For example, Figure 2B shows a three-stage counter-current microSX of high value metals (Pt and rare earths) that was successfully numbered-up 100-fold without losing extraction efficiency (Yang et al., 2022).
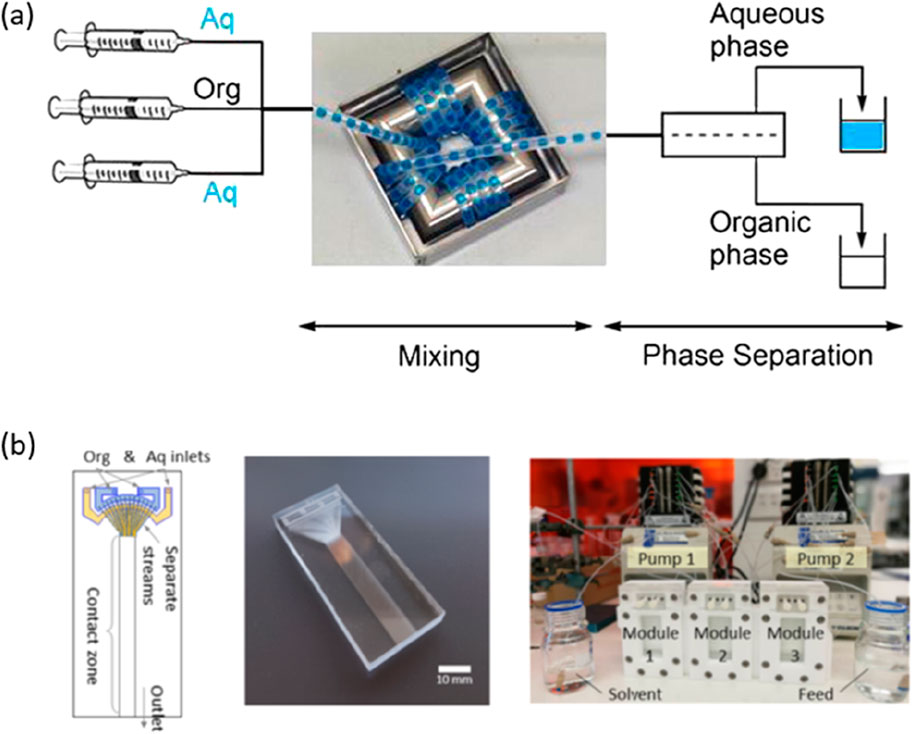
Figure 2. (A) A milli-fluidic extraction configuration, based on coiled tubing and in-line phase separator, capable of pilot-scale volumetric throughput for cobalt extraction. Reprinted from I.V. Gürsel, S.K. Kurt, J. Aalders, Q. Wang, T. Noël, K.D.P. Nigam, N. Kockmann, V. Hessel, Utilization of milli-scale coiled flow inverter in combination with phase separator for continuous flow liquid–liquid extraction processes, Chemical Engineering Journal (2016) 283, 855–868. Copyright (2016), with permission from Elsevier (Vural Gürsel et al., 2016). (B) Numbering-up microSX of Pt or rare earths. Numbering-up was achieved by creating multilayer glass chips (shown) and operating three multi-layer chips in a counter-current or parallel configuration. Reprinted from D. Yang, M. Navvab Kashani, C. Priest, Pilot-scale microfluidic solvent extraction of high-value metals, Minerals Engineering, 182, 107,536 (2022). Copyright (2022), with permission from Elsevier (Yang et al., 2022)
Where full-scale microfluidic processing is not possible, new insight can be obtained through microfluidic analytical strategies. Mineral processing is governed by complex physicochemical processes that occur at the mineral-water interface, including transport and separation, adsorption and desorption, precipitation and dissolution, and atom exchange or redox reactions. These critically control the productivity and efficiency of plant operations at every stage of mineral processing, and may be extremely sensitive to variability in ore chemistry (even within a single ore body). The chemistry of mineral surfaces is typically investigated using well-established laboratory methods, such as batch experiments, column experiments, and spectroscopic analysis. Studying leaching on planar or particulate mineral samples in contact with fluids in micro- or nano-scale confinement offer realistic scenarios for industry-relevant research, allowing process optimization.
2 Micromodels for mineral studies
Microfluidic models provide fluid confinements of variable complexity, ranging from single microchannel of different shapes to patterns representative of natural porous media obtained from imaging or 3D models of real rocks (Anbari et al., 2018). When coupled with optical microscopy, the microfluidic platform enables time-resolved observation of fluid flow behavior within various rock matrix, providing new insights into the effects of dynamic conditions on mineralization processes and dissolution phenomenon. Microfluidic models are being developed to understand the process of mineral dissolution/precipitation from rock matrix for applications mainly in CO2 sequestration (Abolhasani et al., 2014), soil leaching (Zhu et al., 2022), nuclear waste management (Mariet et al., 2019), and more recently in mineral leaching (Yang and Priest, 2018; Yang et al., 2019). The principal challenge in understanding or modeling reactive transport in mineral ores is to account for the subtle effects of fluctuations in geological setting, mineralogy, microorganisms, and environmental variables such as temperature, oxygen, and water that will determine the final composition of the mineral reaction products (Yang et al., 2020). Moreover, it is critical to ground model conceptualizations and test model outputs against laboratory experiments and field measurements.
Early micromodels were generally quasi-two-dimensional models, or simply “2D models”, made from glass, silicon or poly-dimethylsiloxane (PDMS) materials; however, the chosen materials are only limited by fabrication methods and necessary chemical resistance, mechanical stability, and/or optical transparency. These 2D models consist of a planar material with patterned 1–100 μm pore structures in a “chip” with overall dimensions from 1 cm × 1 cm – 10 cm × 10 cm. 2D micromodels offer easy visualization, simplified fabrication, and controlled pore geometry. These 2D models exclude complex 3D phenomena (e.g., flow profiles) but can still offer valuable physicochemical insight. Fabrication of 2D micromodels involves either: (1) etching a porous medium framework into silicon or glass substrates using reactive ion etching or hydrofluoric acid (Chomsurin and Werth, 2003; Zhang et al., 2010; Kim et al., 2013); or (2) allowing a liquid phase polymer (e.g., PDMS) to cure and harden after pouring it into a mold fabricated from either etched silicon or patterned photoresist (i.e., soft-lithography) (Wu et al., 2012; Conn et al., 2014; Xu et al., 2014). They represent excellent geometric representation of rock fractures and enable direct visualization of the fundamental fluid-mineral interactions at the pore-scale, thus have often been used to provide data for development and verification of pore scale modeling (Yoon et al., 2012; Tang et al., 2013; Aufrecht et al., 2019; Yiotis et al., 2021). Several reviews exist in the area covering separately advances in fabrication methods (Gerami et al., 2019; Anbari et al., 2018), visualization techniques (Gerami et al., 2019; Lifton, 2016; Jahanbakhsh et al., 2020) and their application in different areas including petroleum, geological, and environmental engineering (Gogoi and Gogoi, 2019; Lifton, 2016; Zhu et al., 2022; Peng et al., 2021).
Traditional micromodels, however, struggle to capture the physical and geochemical fluid–rock interactions expected in rock. Specifically, wettability, pore-scale heterogeneities, and geochemical interactions are not replicated well. As the transport and reaction dynamics also depend on the surface chemistry of rock itself, visualizing these processes in an environment similar to that of rock (mineral ore) is a typical consideration in the design of microfluidic devices in this field of study. Where successful, this approach can mimic the fundamental physics and chemistry that occur in fractured rocks (cf. Figure 3) or the porosity of particle beds. Micromodels that include real geomaterial have emerged, where microchannels are either etched or milled into thin sections of different rock types (e.g., shale, sandstone, and siltstone (Porter et al., 2015) or coal (Gerami et al., 2017)) and sealed against a flat PDMS, or prepared in the opposite way (i.e., a PDMS microchannel is sealed against a flat sample such as thin gold film (Yang and Priest, 2018; Kotova et al., 2017), calcite (Ciceri and Allanore, 2015), chalcopyrite (Yang et al., 2019)).
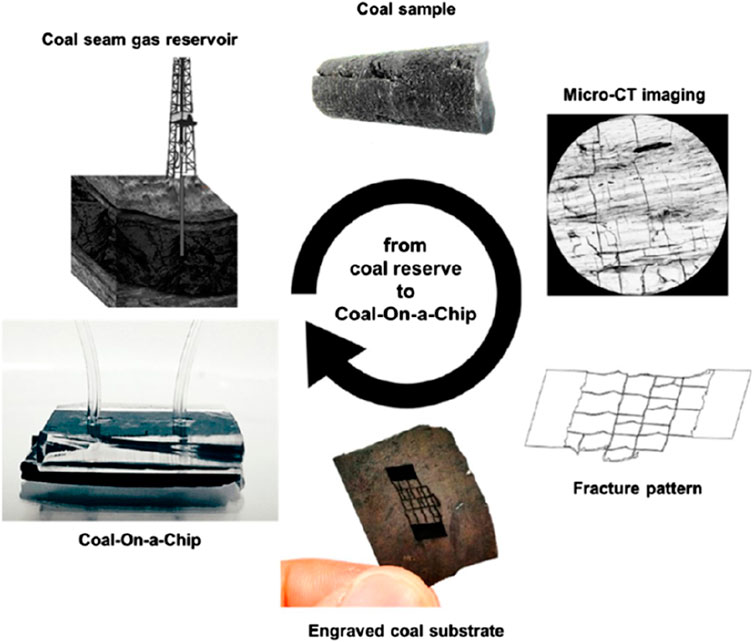
Figure 3. Preparation of realistic porous structures by laser etching of coal based on microtomography of fracture patterns. Reprinted with permission from (Gerami et al., 2017), Energy Fuels (2017) 31, 10,393–10403. Copyright 2020 American Chemical Society.
Other microfluidic device modifications that aid in representing real rock were also reported, including functionalized microfluidic platforms that provide attachment of real rock minerals onto silicon or PDMS. For example, Song and Kovscek (Song et al., 2015) created a functionalized two-dimensional silicon micromodel with pore surfaces coated with kaolinite clay for direct and real-time visualization of fluid–solid interactions. Lee et al. (2016) and Wang et al. (2017) developed a method to fabricate calcium carbonate micromodels by in situ growing a thin layer of CaCO3 nanocrystals onto glass microfluidic channels that allows the direct visualization of the complex multiphase flows and geochemical fluid–calcite interactions. Lee et al. (Lee et al., 2016) tracked the thickness of CaCO3 films while exposed to flow of a Ca-rich aqueous phase past posts or pores. The spatial confinement effects on local flow were shown to affect the CaCO3 growth. Furthermore, these CaCO3-coated channels were subjected to acid dissolution, which revealed inhomogeneous progression of CaCO3 dissolution. Wang et al. (Wang et al., 2017) followed CaCO3 growth with exposure to crude oil and aging (150°C for 12 h) to modify the microenvironment wettability to the oil-wet (hydrophobic) calcite reservoirs encountered in real systems. The latter proved useful in studying oil-water (two-phase) flow in these environments, where the liquid phases were fluorescently labelled and observed by optical microscopy. Using the same fabrication method, Yun et al. (Yun et al., 2020) studied the effect of surfactants and interfacial tension on oil-water phase behavior in calcite-coated micromodel, which may provide a quick assessment of surfactants for enhanced oil recovery. The volumetric flow rate was 0.1 μL/min and the Darcy velocity was 2.4 ft/day in these experiments. Shaik et al. adapted the CaCO3 coating method to promote calcite over vaterite mineralogy in the microchannel (Shaik et al., 2021) and studied the effect of aqueous phase salinity on the alteration of calcite reservoir wettability (Shaik et al., 2022). Alzahid et al. (2018) proposed a different process for functionalizing the microfluidic models with rock minerals (including quartz, kaolinite and calcite) through chemical bonding of functional groups of geomaterials with silanol groups on the plasma treated PDMS surface. Using bacteria to enable calcite precipitation and growth on solid surfaces were also reported for geomaterial functionalized micromodels (Song et al., 2018). All these geomaterial modified micromodels permitted detailed studies of dissolution, leaching and liquid displacement processes including the relevant fluid–solid chemical reactions which conventional microfluidics/micromodels cannot replicate in a naturally occurring mineral substrate. However, these methods are generally limited to sample materials that are readily fabricated and optically interrogated, such as calcite. Furthermore, mimicking the natural wall roughness of the fractures with high precision and having flexibility to allow fracture deformation has remained challenging.
To overcome these limitations, Osselin et al. (2016) designed a simple microfluidic setup by inserting a gypsum block in between two polycarbonate plates. This sandwich shape of design allows the flow through experiments to study the classical case of reactive infiltration, analogous to the processes taking place in reservoir stimulation or karst formation. Based on this design, Dutka et al. (2020) modified the microfluidic cell and used it to observe changes in size and shape as gypsum dissolved in flowing water. In addition, Singh et al. (2017) developed an analogous microfluidic platform called “Real Rock Microfluidic Flow Cell” by mounting a thin section (500 μm) of a sandstone rock between two PDMS layers that were bonded via a plasma generator, creating a dynamic flow-through cell for real-time tracking of subsurface reactive transport. Another PDMS micromodel flow cell was constructed more recently by Alarji et al. (2022) by placing thin section (1 mm thickness) of two carbonate rock wafers into a PDMS mold to allow the injection of reactive fluids into the rock sample. This setup enabled the direct measurement of the acidic fluid reaction rate with carbonate rocks under flowing conditions by quantifying the evolved CO2 gas over time. These microfluidic approaches have helped to investigate a variety of phenomena such as wettability, mass transfer, flow regime, the influence of flow rate on residual oil saturations, and three-phase flow in fractures (Anbari et al., 2018; Jahanbakhsh et al., 2020) In general, these microfluidic devices are enclosed microstructures to which geomaterials are grafted by either inserting delicately milled or machined rock wafers into micromodels or growing the mineral crystals in the confined space of the microfluidic systems.
3 Leaching minerals in microdevices
Traditional studies of mineral leaching are carried out in large columns, heaps, or laboratory apparatus over times ranging from hours to months. Sample sizes are often measured in kg or t, depending on the batch size, and the use of leaching reagents (often acids) is also high. Moreover, spatial analysis of leachate and mineral chemistry in these large systems is limited, due to the possible number, type, and location of suitable sensors. Visual analysis is generally impossible. In contrast, microfluidic mineral leaching platforms are small, give faster results, use orders of magnitude less sample and reagent, and offer in situ analysis of both leachate and mineral samples without compromising the microscale spatial confinement effects and relevance of the mineralogy studied, as discussed further in this section.
During leaching, the microscale environment and surface wettability dominates fluid behavior (capillary forces and laminar flow, which are defined by the particular spatial confinement and fluid properties). In addition, the high surface-to-volume ratio leads to interfacial reactions that are affected by local concentration gradients, the rate of local surface reactions, and surface reactions that may adjust the surface charge (affecting electrical double layer phenomena). It is therefore important that experimental investigations of mineral leaching mimic the microscale geometry, fluid properties (including reactivity), and surface chemistry accurately. Song et al. (2014) presented an initial attempt on the application of real rock micromodels in visualizing calcite dissolution that is relevant to oil/gas recovery, CO2 sequestration, and wastewater disposal in carbonate formations. The transparent chip allowed direct visualization of preferential dissolution along crystal planes and phenomena such as “wormholing”, where dissolution is faster along preferential flow pathways. Later, Ciceri and Allanore (Ciceri and Allanore, 2015) elucidated the microfluidic nature of K+ ions leaching from a K-feldspar-bearing rock (syenite) (a key step of weathering of soil mineral in agriculture) by measuring the leaching kinetics in a microfluidic environment. This study required the collection of time-resolved samples in vials, which is an approach commonly used to enable sensitive off-chip analysis methods (e.g., ICP-MS). Kotova et al. reported a hybrid gold-PDMS microfluidic chip for investigating gold leaching in a high aspect ratio microchannel, i.e., a crack or fracture, in real-time (Kotova et al., 2017). The method exploited the quantitative relationship between optical transparency and film thickness, which allowed in situ monitoring of the leach rate and profile. Later, Yang et al. (Yang and Priest, 2018) and Azadi et al. (Azadi et al., 2019) applied the method to investigate gold leach conditions under controlled flow, temperature, and fluid composition. Rapid screening of leach parameters, including aspects of mechanisms and kinetics, was also enabled using minimal reagent volumes and amounts of gold (mL/mg level) (Yang and Priest, 2018). Yang et al. extended the hybrid chip concept to include a planar chalcopyrite ore in a “ore-on-a-chip” for studying mineral leaching, as shown in Figure 4 (Yang et al., 2019). The new platform was shown to be suitable for studying leaching of the notoriously complex chalcopyrite chemistry, which has proven difficult to study using conventional techniques. Nonetheless, mineral ores are natural materials which are typically rough and exhibit significant geological diversity in terms of composition, structure, and abundance. This complexity significantly complicates the preparation of representative rock-on-a-chip micromodels.
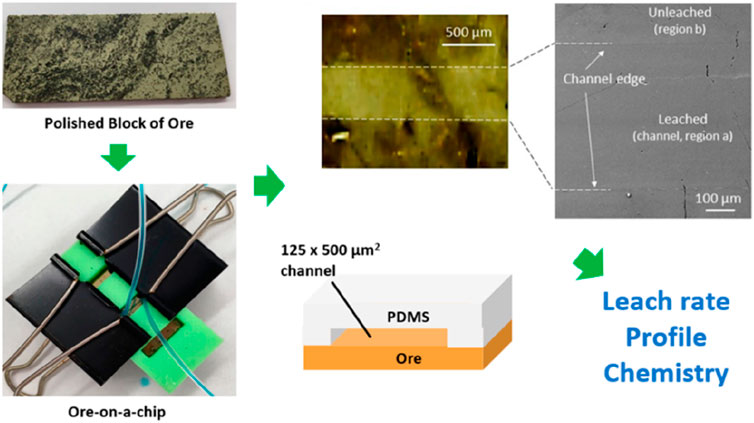
Figure 4. An “ore-on-a-chip” used to study mineral leaching. The polished chalcopyrite ore and poly (dimethysiloxane) microchannel were sandwiched to form the hybrid chip, in a custom holder (green) that allows visual inspection. Reprinted with permission from (Yang et al., 2019), Anal. Chem. 2019, 91, 1,557–1,562. Copyright 2020 American Chemical Society.
Unlike many of the planar micromodels, natural formations are typically rough and chemically heterogeneous. This significantly complicates dissolution, adsorption, leaching and other physio-chemical processes related geological character. Mineral leaching studies are particularly challenging where the sample exhibits a confluence of complex composition, shape, and species diversity. Heterogeneous microparticle samples are a prime example of this complexity and are ubiquitous to mineral processing in the form of particle beds (e.g., heap leaching) or slurries (e.g., froth flotation). To this end, Yang et al. (Yang et al., 2020) developed a microfluidic platform that allows the investigation of mineral dissolution occurring at the solid/liquid interface of particles, cf. Figure 5. The particulate mineral sample is used as received (i.e., ore particles after grind or flotation steps, cf. Figure 1) and can be directly loaded into this microfluidic device, without the need for flat, large areas (e.g., polished or embedded in resin). Samples can be obtained direct from mine sites and, in many cases, screening at the mineral processing site would be possible. The approach enables evaluation of mineral dissolution/leaching for a range of reaction conditions can be carried out using real rock samples with species diversity and may be useful in non-mining scenarios (such as contaminated soils or similar environmental issues).
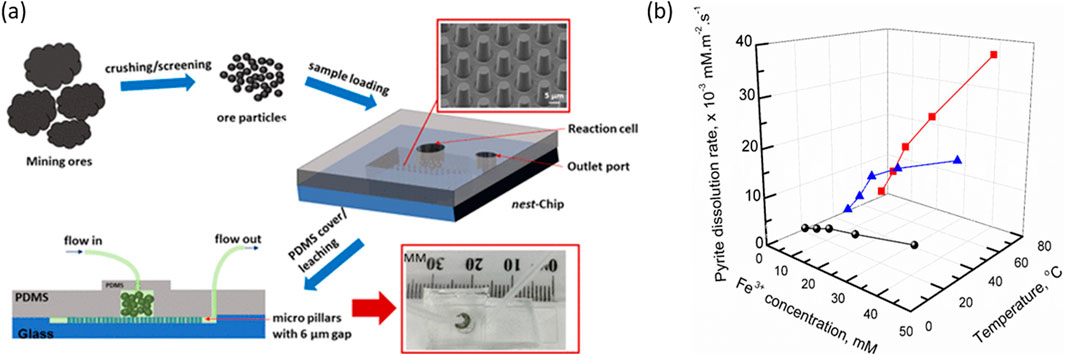
Figure 5. (A) Microparticle “nests” for high throughput screening of mineral leaching. (B) Dissolution rates obtained by microleaching pyrite particles at different temperature and Fe(III) concentration for the prediction of acid mine drainage. Adapted and reprinted with permission from (Yang et al., 2020), Environ. Sci. Technol. 2020, 54, 21, 14,000–14006. Copyright 2020 American Chemical Society.
A scenario that is encountered both in mining and environmental management is acid mine drainage (AMD). It is considered the most significant environmental pollution problem associated with mining industry. AMD forms when sulphide minerals are exposed to oxidizing conditions, e.g., water and oxygen, which occurs naturally where sulphide minerals exist in water-saturated zones. However, AMD can be accelerated by the production of broken waste rock and tailings through mining operations. AMD mineral-water reactions are typically slow and may take years to occur. Modelling of AMD processes is essential for the early diagnosis, prediction, and technological analyses, which can lead to improved energy, economic, and environmental outcomes. To understand AMD and develop predictive models that represent a wide variety of mine site chemistry, the analytical methods must deliver large and representative data sets across a wide parameter space (mineralogy and water chemistry). Bulk-scale methods are slow and inefficient in this regard, making studies of AMD thermodynamics practically challenging.
Applying microfluidic techniques to studying AMD is an emerging opportunity. Screening methods must be able to study a wide scope of rock-liquid interactions during AMD formation including adsorption, dissolution, and other surface chemistry phenomena across many different rock matrices. For example, formation of AMD from waste rock and tailings is highly dependent on local environments including geological setting, mineralogy, presence of microorganisms, and other environmental variables such as temperature, oxygen, and water. These factors are highly variable and, therefore, case-specific testing and/or wide parameter screening using microfluidic methods is highly desirable. Furthermore, accurate control of key physio-chemical variables such as temperature are straight-forward on a chip, while being energy intensive and/or impractical over the longer term (years) in conventional large-scale experiments.
Deng et al. (2020) demonstrated the application of a real-rock microfluidic cell coupled with synchrotron-based observations for the investigation of acid erosion of a rock surface with mixed mineral composition. Unlike many studies to date, the authors employed in situ X-ray attenuation imaging (in addition to ex-situ XRF and XCT). Park et al. (2021) used a microfluidic pore model to investigate the pore-scale mixing of FeSO4 solution with simulated groundwaters to assess the environmental implications of AMD infiltration into a shallow aquifer. Yang et al. (2020) developed a microfluidic screening method for AMD, which is able to screen reaction conditions with good spatiotemporal control over the process. Reagent and sample consumption were greatly reduced to mL and mg levels, compared with conventional bulk-scale screening (kg and L). These microfluidic AMD studies offer a path toward effective long-term management of AMD through a better understanding of leach behavior of sulphide minerals under actual field conditions.
4 Monitoring mineral slurries
During mineral processing, plant operators need accurate real-time data on key operational parameters at all stages of processing to make informed decisions for improved process control and optimization. The development of integrated microfluidic sensors using microfluidic technologies allows multistep chemical assays in a portable, robust, and user-friendly platform using small sample volumes (µL–nL range) with minimal sample loss and rapid assay time (Erickson and Li, 2004). Such sensors have proven to be successful tools for diagnostic applications in bioanalysis and health management, food safety and environmental quality monitoring, and chemical analysis (Schulte et al., 2002; Gao et al., 2020; Zhang et al., 2021). However, when applied to mineral streams, the microchannels become blocked by particulate matter because of their small size which is similar to that of some of the particles in the mineral stream. To solve this problem, Shallan et al. (Shallan et al., 2021) reported a new open-microfluidic strategy that prevents clogging by creating a dynamic fluidic obstacle outside of the channel. The approach excludes small particles, while allowing soluble ions to freely enter the channel for analysis, cf. Figure 6. This allows efficient on-chip separation of analytes from undiluted slurries and buffering of the reaction matrix. Using the same open-chip, Yang et al. reported successful continuous monitoring of ethylenediaminetetraacetic acid (EDTA) extractable iron from mineral slurries (Yang et al., 2023). These are good examples of how novel microfluidic flows may offer continuous and real-time monitoring of soluble chemistry, even in complex mineral slurry samples.
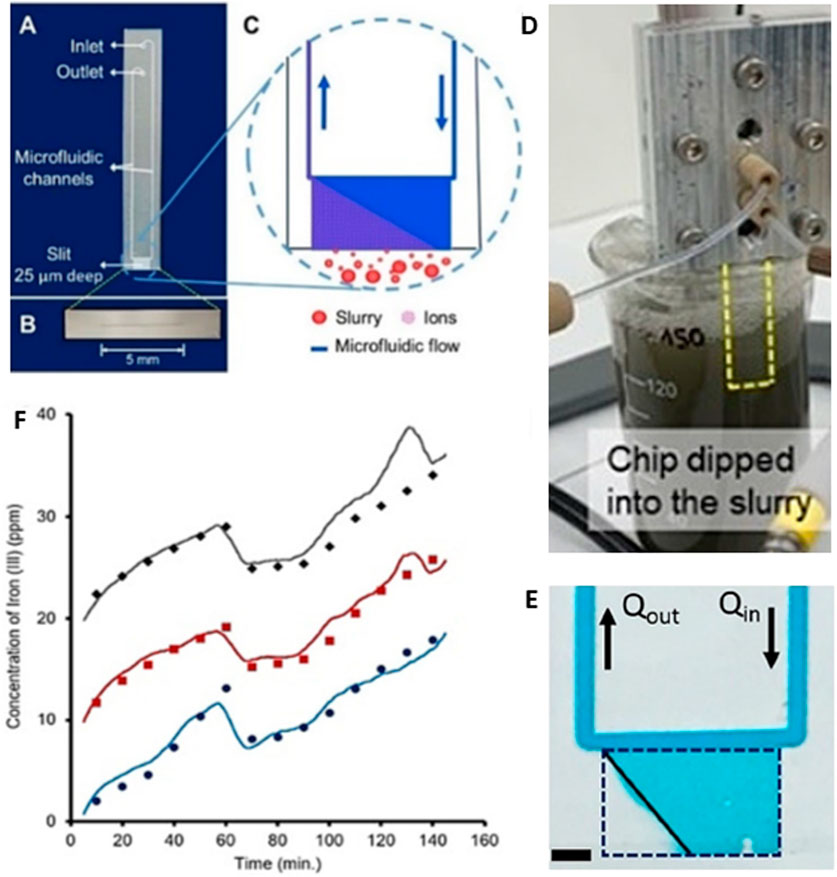
Figure 6. Continuous monitoring of Fe(III) leaching with EDTA from a mineral processing slurry. Chip design (A) including microscale slit-type open channel (B) and schematic of operation (C). An internal push-pull flow (1 mL/h) is generated within the chip by a syringe pump. A small portion of the internal aqueous fluid exchanges with the external (slurry) aqueous fluid, which flows downstream to a z-cell for continuous UV/Vis monitoring within ∼5 min (D) Experiment set-up showing the chip immersed in a stirred mineral particle slurry. (E) Image of chip in operation; the inner fluid is indicated by the blue dye and the clear fluid (triangular region in the mouth of the chip) indicates the external fluid entering the channel (F) Results for monitoring Fe(III) from the slurry using the chip (lines) and off-chip after filtration (points) over 2.5 h. Data is plotted offset for clarity. Figure adapted from (Shallan et al., 2021) and used with permission, © 2020 Wiley-VCH GmbH.
5 Summary, opportunities, and challenges
Table 1 provides a summary of the microfluidic studies of mineral leaching, with details of the chip configuration, minerals and fluids studied, and analyses employed. When gas and oil recovery applications are excluded (as they are in this table), it becomes clear that mineral leaching studies using rock-on-a-chip platforms are few, presenting an opportunity for further study. There is good agreement in the literature that using real mineral samples (rock-on-a-chip platforms) is more industrially-relevant when studying the complex physicochemical behavior encountered in the mining industry.
Complex leaching reactions on ores embedded in microscale confinement have been shown to elucidate fast and safe screening of leaching conditions at low cost and with minimal waste. Industrial application may include rapid mine site testing to forecast and optimize downstream processing. The approach may also be useful in developing novel leaching reagents, developing new strategies for processing low-grade ores, or studying synergistic or antagonistic interactions between minerals. Further, there is scope to vary solution chemistry, hydrodynamics, ore composition (e.g., minerology and structures), the presence of bacteria, and chemical transformations (including displacement, precipitation, dissolution, and redox reactions) that cause physicochemical feedback that directly affects the biological, physical, and/or chemical leaching environment. Quantitative information obtained from studies of this kind may be used to derive useful parameters for predictive models. Microfluidic devices themselves may completely replace complex and expensive equipment, along with manual handling of large samples; however, technical challenges remain.
Further opportunities exist where models and simulations of mineral leaching can be tested more readily with microfluidic rock-on-a-chip platforms. Conventional leaching experiments do not reveal the physicochemical phenomena with sufficient detail and certainty for reliable comparisons to be made with simulations. However, microfluidic platforms can reveal optical and spectroscopic information, and real-time solution chemistry. Furthermore, machine learning has been identified as a promising strategy for optimizing mineral leaching, as summarized by Saldaña et al. in a recent review (Saldaña et al., 2022). Coupling machine learning with high throughput microfluidic screening of mineral leaching is likely to have a major impact on the mining sector.
A challenge remaining is representative sampling and reliable scaling of throughput to achieve sufficient data for industrial decisions. For example, gold ore grades can range from <5 g/t (low-grade) to 30 g/t (Yang and Priest, 2018). As grade reduces, the liberation and recovery of metals from sample ores will require increased sample volume to ensure representative sampling for conventional leaching tests. One strategy is to complete a large number of parallel analyses to yield an average result with reasonable uncertainty. However, further research to ensure analytical results from microfluidic leaching are representative of those obtained using existing methodologies will be required.
Another challenge is the analysis and characterization methods for probing interfacial processes, reactions, and outcomes in the microfluidic systems. The advantages of microfluidic methods are less attractive if analysis requires the collection of large samples to be compatible with conventional (lab-scale) analytical methods. In some cases, typical sample volumes will be several microliters or tens of microliters, which is unsuitable for common bulk analytical instruments. This issue can sometimes be overcome by dilution of small volumes of concentrated samples or prolonged collection times. Neither approach is attractive. Dilution may affect the matrix, resulting in precipitation or shifts in industrially important solution parameters (e.g., pH). Long collection times reduce the opportunity for time-resolved data points and does not permit a fast answer. A more effective solution is to develop on-chip or in situ analysis techniques. This is technically challenging and requires more sophisticated process design and control but offers time-resolved data (Zhu et al., 2022). Currently, optical (e.g., UV-vis spectra) and imaging techniques are used for the on-chip monitoring of mineral dissolution during leaching processes (Ciceri and Allanore, 2015; Yang et al., 2019; Kotova et al., 2017; Azadi et al., 2019). There is a need to develop other spectroscopic methods (e.g., Raman and mass spectrometry) or chemical probes (e.g., electrodes) for quantitative on-chip measurements, so that both aqueous and interfacial chemical information can be acquired in situ for rapid diagnostic of mineral leaching.
Despite these challenges, the present authors expect that microfluidic devices will play an increasing role in industrial optimization of mineral leaching operations along the entire process chain, shown in Figure 1. This outlook, however, will depend on a sustained research effort and strategic industry partnerships that fully realize the opportunities and benefits of microfluidic leaching at the mine site in the near and long-term future.
6 Conclusion
Microfluidic and LOC technologies have emerged as key enablers in understanding fundamental chemical processes occurring in mineral processing. This review addresses the various micromodels developed for resource and mineral studies. Researchers have demonstrated that these small volume, high precision platforms offer greater control over experimental conditions than presently achievable in traditional bulk-scale testing. In particular, flow profiles and interfacial chemistry can mimic real scenarios, leading to results that inform predictive models or address industry case studies. Special attention was given to leaching from natural ores with typically high surface-to-volume ratios (particles or rock with cracks or fractures). The combination of relevant microstructure and relevant ore interfaces in the so-called “rock-on-a-chip” chips–a hybrid chip integrating a real ore sample - has proven particularly insightful to researchers. Screening of reaction rates over a wide range of conditions has provided greater insight into leaching reaction rates, while using small volumes of sample and reagent. This review also highlighted opportunities for studying acid mine drainage, which exhibits complex site-specific chemistry. The large parameter space accessible through microfluidic platforms offers a promising approach to predicting long-term outcomes. However, challenges remain: small samples require careful experimental design to ensure that sufficient volume is available for the chosen analytical methods and the results are representative of industrial processes. To address these and other challenges, microfluidic researchers will need to closely partner with industry to study rock-on-a-chip and similar microfluidic platforms that can incorporate industry-relevant samples.
Author contributions
DY: Writing–original draft, Writing–review and editing. CP: Writing–original draft, Writing–review and editing.
Funding
The author(s) declare that financial support was received for the research, authorship, and/or publication of this article. The authors acknowledge financial support from the Australian Research Council Integrated Devices for End-User Analysis at Low Levels Research (IDEAL) Hub (IH150100028).
Acknowledgments
The South Australian Node of the Australian National Fabrication Facility (ANFF-SA) is part of Australia’s National Collaborative Research Infrastructure Strategy (NCRIS), supported by the Australian Government, Government of South Australia, and University of South Australia.
Conflict of interest
The authors declare that the research was conducted in the absence of any commercial or financial relationships that could be construed as a potential conflict of interest.
The handling editor VH declared a past co-authorship with the author CP.
Publisher’s note
All claims expressed in this article are solely those of the authors and do not necessarily represent those of their affiliated organizations, or those of the publisher, the editors and the reviewers. Any product that may be evaluated in this article, or claim that may be made by its manufacturer, is not guaranteed or endorsed by the publisher.
References
Abolhasani, M., Günther, A., and Kumacheva, E. (2014). Microfluidic studies of carbon dioxide. Angew. Chem. Int. Ed. 53, 7992–8002. doi:10.1002/anie.201403719
Alarji, H., Alzahid, Y., and Regenauer-Lieb, K. (2022). Acid stimulation in carbonates: microfluidics allows accurate measurement of acidic fluid reaction rates in carbonate rocks by quantifying the produced co2 gas. J. Nat. Gas Sci. Eng. 99, 104444. doi:10.1016/j.jngse.2022.104444Available at: https://www.sciencedirect.com/science/article/pii/S1875510022000373.
Alzahid, Y. A., Mostaghimi, P., Gerami, A., Singh, A., Privat, K., Amirian, T., et al. (2018). Functionalisation of polydimethylsiloxane (pdms)- microfluidic devices coated with rock minerals. Sci. Rep. 8, 15518. doi:10.1038/s41598-018-33495-8
Anbari, A., Chien, H.-T., Datta, S. S., Deng, W., Weitz, D. A., and Fan, J. (2018). Microfluidic model porous media: fabrication and applications. Small 14, 1703575. doi:10.1002/smll.201703575
Andersson, H., and van den Berg, A. (2003). Microfluidic devices for cellomics: a review. Sensors Actuators B Chem. 92, 315–325. doi:10.1016/S0925-4005(03)00266-1Available at: https://www.sciencedirect.com/science/article/pii/S0925400503002661.
Atalay, Y. T., Vermeir, S., Witters, D., Vergauwe, N., Verbruggen, B., Verboven, P., et al. (2011). Microfluidic analytical systems for food analysis. Trends Food Sci. & Technol. 22, 386–404. doi:10.1016/j.tifs.2011.05.001Available at:. https://www.sciencedirect.com/science/article/pii/S0924224411000902.
Aufrecht, J. A., Fowlkes, J. D., Bible, A. N., Morrell-Falvey, J., Doktycz, M. J., and Retterer, S. T. (2019). Pore-scale hydrodynamics influence the spatial evolution of bacterial biofilms in a microfluidic porous network. PLOS ONE 14, e0218316. doi:10.1371/journal.pone.0218316
Azadi, M. R., Karrech, A., Elchalakani, M., and Attar, M. (2019). Microfluidic study of sustainable gold leaching using glycine solution. Hydrometallurgy 185, 186–193. doi:10.1016/j.hydromet.2019.02.009Available at: https://www.sciencedirect.com/science/article/pii/S0304386X18307035.
Bao, B., Riordon, J., Mostowfi, F., and Sinton, D. (2017). Microfluidic and nanofluidic phase behaviour characterization for industrial co2, oil and gas. Lab a Chip 17, 2740–2759. doi:10.1039/C7LC00301C
Bazazi, P., Sanati-Nezhad, A., and Hejazi, S. H. (2019). Role of chemical additives on water-based heavy oil mobilization: a microfluidic approach. Fuel 241, 1195–1202. doi:10.1016/j.fuel.2018.12.099Available at: https://www.sciencedirect.com/science/article/pii/S0016236118321707.
Cao, S. C., Jung, J., and Radonjic, M. (2019). Application of microfluidic pore models for flow, transport, and reaction in geological porous media: from a single test bed to multifunction real-time analysis tool. Microsyst. Technol. 25, 4035–4052. doi:10.1007/s00542-019-04612-y
Chomsurin, C., and Werth, C. J. (2003). Analysis of pore-scale nonaqueous phase liquid dissolution in etched silicon pore networks. Water Resour. Res. 39. doi:10.1029/2002WR001643Available at: https://agupubs.onlinelibrary.wiley.com/doi/abs/10.1029/2002WR001643.
Ciceri, D., and Allanore, A. (2015). Microfluidic leaching of soil minerals: release of k+ from k feldspar. PLOS ONE 10, e0139979. doi:10.1371/journal.pone.0139979
Conn, C. A., Ma, K., Hirasaki, G. J., and Biswal, S. L. (2014). Visualizing oil displacement with foam in a microfluidic device with permeability contrast. Lab a Chip 14, 3968–3977. doi:10.1039/C4LC00620H
Deng, H., Fitts, J. P., Tappero, R. V., Kim, J. J., and Peters, C. A. (2020). Acid erosion of carbonate fractures and accessibility of arsenic-bearing minerals: in operando synchrotron-based microfluidic experiment. Environ. Sci. & Technol. 54, 12502–12510. doi:10.1021/acs.est.0c03736
Dutka, F., Starchenko, V., Osselin, F., Magni, S., Szymczak, P., and Ladd, A. J. C. (2020). Time-dependent shapes of a dissolving mineral grain: comparisons of simulations with microfluidic experiments. Chem. Geol. 540, 119459. doi:10.1016/j.chemgeo.2019.119459Available at: https://www.sciencedirect.com/science/article/pii/S0009254119305881.
Erickson, D., and Li, D. (2004). Integrated microfluidic devices. Anal. Chim. Acta 507, 11–26. doi:10.1016/j.aca.2003.09.019Available at: https://www.sciencedirect.com/science/article/pii/S0003267003012261.
Gao, H., Yan, C., Wu, W., and Li, J. (2020). Application of microfluidic chip technology in food safety sensing. Sensors 20, 1792. doi:10.3390/s20061792Available at: https://www.mdpi.com/1424-8220/20/6/1792.
Gerami, A., Alzahid, Y., Mostaghimi, P., Kashaninejad, N., Kazemifar, F., Amirian, T., et al. (2019). Microfluidics for porous systems: fabrication, microscopy and applications. Transp. Porous Media 130, 277–304. doi:10.1007/s11242-018-1202-3
Gerami, A., Armstrong, R. T., Johnston, B., Warkiani, M. E., Mosavat, N., and Mostaghimi, P. (2017). Coal-on-a-chip: visualizing flow in coal fractures. Energy & Fuels 31, 10393–10403. doi:10.1021/acs.energyfuels.7b01046
Gogoi, S., and Gogoi, S. B. (2019). Review on microfluidic studies for eor application. J. Petroleum Explor. Prod. Technol. 9, 2263–2277. doi:10.1007/s13202-019-0610-4
Jahanbakhsh, A., Wlodarczyk, K. L., Hand, D. P., Maier, R. R. J., and Maroto-Valer, M. M. (2020). Review of microfluidic devices and imaging techniques for fluid flow study in porous geomaterials. Sensors 20, 4030. doi:10.3390/s20144030Available at: https://www.mdpi.com/1424-8220/20/14/4030.
Jiang, F., Pei, J., Yin, S., Zhang, L., Peng, J., Ju, S., et al. (2018). Solvent extraction and stripping of copper in a y-y type microchannel reactor. Miner. Eng. 127, 296–304. doi:10.1016/j.mineng.2018.08.014Available at: https://www.sciencedirect.com/science/article/pii/S0892687518303558.
Keshmiri, K., Huang, H., and Nazemifard, N. (2019). Microfluidic platform to evaluate asphaltene deposition during solvent-based extraction of bitumen. Fuel 239, 841–851. doi:10.1016/j.fuel.2018.11.044Available at: https://www.sciencedirect.com/science/article/pii/S001623611831932X.
Kim, M., Sell, A., and Sinton, D. (2013). Aquifer-on-a-chip: understanding pore-scale salt precipitation dynamics during co2 sequestration. Lab a Chip 13, 2508–2518. doi:10.1039/C3LC00031A
Kolar, E., Catthoor, R. P. R., Kriel, F. H., Sedev, R., Middlemas, S., Klier, E., et al. (2016). Microfluidic solvent extraction of rare earth elements from a mixed oxide concentrate leach solution using Cyanex® 572. Chem. Eng. Sci. 148, 212–218. doi:10.1016/j.ces.2016.04.009Available at: https://www.sciencedirect.com/science/article/pii/S0009250916301658.
Kotova, S., Follink, B., Del Castillo, L., and Priest, C. (2017). Leaching gold by reactive flow of ammonium thiosulfate solution in high aspect ratio channels: rate, passivation, and profile. Hydrometallurgy 169, 207–212. doi:10.1016/j.hydromet.2017.01.015Available at: http://www.sciencedirect.com/science/article/pii/S0304386X1630771X.
Kriel, F. H., Holzner, G., Grant, R. A., Woollam, S., Ralston, J., and Priest, C. (2015). Microfluidic solvent extraction, stripping, and phase disengagement for high-value platinum chloride solutions. Chem. Eng. Sci. 138, 827–833. doi:10.1016/j.ces.2015.08.055Available at: https://www.sciencedirect.com/science/article/pii/S0009250915006302.
Le, T. N. Q., Tran, Q. D., Tran, N. N., Priest, C., Skinner, W., Goodsite, M., et al. (2022). Critical elements: opportunities for microfluidic processing and potential for esg-powered mining investments. Green Chem. 24: 8879–8898. doi:10.1039/D2GC02214A
Lee, S. G., Lee, H., Gupta, A., Chang, S., and Doyle, P. S. (2016). Site-selective in situ grown calcium carbonate micromodels with tunable geometry, porosity, and wettability. Adv. Funct. Mater. 26, 4896–4905. doi:10.1002/adfm.201600573
Lei, W., Liu, T., Xie, C., Yang, H., Wu, T., and Wang, M. (2020). Enhanced oil recovery mechanism and recovery performance of micro-gel particle suspensions by microfluidic experiments. Energy Sci. & Eng. 8, 986–998. doi:10.1002/ese3.563
Li, C., Jiang, F., Ju, S., Peng, J., Wei, Y., and Zhang, L. (2015). Separation of in3+ and fe3+ from sulfate solutions using d2ehpa in a laminar microreactor. Can. Metall. Q. 54, 432–438. doi:10.1179/1879139515Y.0000000005
Li, C., Jiang, F., Ju, S., Peng, J., and Zhang, L. (2016). “Recovery and purification of in3+ from zinc hydrometallurgical process in a t-junction microchannel,” in Rare metal technology 2016 (Cham: Springer International Publishing), 183–195.
Lifton, V. A. (2016). Microfluidics: an enabling screening technology for enhanced oil recovery (eor). Lab a Chip 16, 1777–1796. doi:10.1039/C6LC00318D
Lim, Y. C., Kouzani, A. Z., and Duan, W. (2010). Lab-on-a-chip: a component view. Microsyst. Technol. 16, 1995–2015. doi:10.1007/s00542-010-1141-6
Mariet, C., Vansteene, A., Losno, M., Pellé, J., Jasmin, J.-P., Bruchet, A., et al. (2019). Microfluidics devices applied to radionuclides separation in acidic media for the nuclear fuel cycle. Micro Nano Eng. 3, 7–14. doi:10.1016/j.mne.2019.02.006Available at: https://www.sciencedirect.com/science/article/pii/S2590007219300115.
Morais, S., Cario, A., Liu, N., Bernard, D., Lecoutre, C., Garrabos, Y., et al. (2020). Studying key processes related to co2 underground storage at the pore scale using high pressure micromodels. React. Chem. & Eng. 5, 1156–1185. doi:10.1039/D0RE00023J
Oh, Y. S., Jo, H. Y., Ryu, J. H., and Kim, G. Y. (2017). A microfluidic approach to water-rock interactions using thin rock sections: Pb and u sorption onto thin shale and granite sections. J. Hazard Mater 324, 373–381. doi:10.1016/j.jhazmat.2016.10.071
Osselin, F., Kondratiuk, P., Budek, A., Cybulski, O., Garstecki, P., and Szymczak, P. (2016). Microfluidic observation of the onset of reactive-infitration instability in an analog fracture. Geophys. Res. Lett. 43, 6907–6915. doi:10.1002/2016GL069261Available at: https://agupubs.onlinelibrary.wiley.com/doi/abs/10.1002/2016GL069261.
Park, S., Anggraini, T. M., Chung, J., Kang, P. K., and Lee, S. (2021). Microfluidic pore model study of precipitates induced by the pore-scale mixing of an iron sulfate solution with simulated groundwater. Chemosphere 271, 129857. doi:10.1016/j.chemosphere.2021.129857
Peng, X., Wang, X., Zhou, X., Lin, Z., Zeng, F., and Huang, X. (2021). Lab-on-a-chip systems in imbibition processes: a review and applications/issues for studying tight formations. Fuel 306, 121603. doi:10.1016/j.fuel.2021.121603Available at: https://www.sciencedirect.com/science/article/pii/S0016236121014848.
Porter, M. L., Jiménez-Martínez, J., Martinez, R., McCulloch, Q., Carey, J. W., and Viswanathan, H. S. (2015). Geo-material microfluidics at reservoir conditions for subsurface energy resource applications. Lab a Chip 15, 4044–4053. doi:10.1039/C5LC00704F
Priest, C., Zhou, J., Sedev, R., Ralston, J., Aota, A., Mawatari, K., et al. (2011). Microfluidic extraction of copper from particle-laden solutions. Int. J. Mineral Process. 98, 168–173. doi:10.1016/j.minpro.2010.11.005Available at:. https://www.sciencedirect.com/science/article/pii/S0301751610001523.
Saldaña, M., Neira, P., Gallegos, S., Salinas-Rodríguez, E., Pérez-Rey, I., and Toro, N. (2022). Mineral leaching modeling through machine learning algorithms − a review. Front. Earth Sci. 10. doi:10.3389/feart.2022.816751Available at: https://www.frontiersin.org/journals/earth-science/articles/10.3389/feart.2022.816751.
Schenk, R., Hessel, V., Hofmann, C., Löwe, H., and Schönfeld, F. (2003). Novel liquid-flow splitting unit specifically made for numbering-up of liquid/liquid chemical microprocessing. Chem. Eng. & Technol. 26, 1271–1280. doi:10.1002/ceat.200301867
Schulte, T. H., Bardell, R. L., and Weigl, B. H. (2002). Microfluidic technologies in clinical diagnostics. Clin. Chim. Acta 321, 1–10. doi:10.1016/S0009-8981(02)00093-1Available at: https://www.sciencedirect.com/science/article/pii/S0009898102000931.
Shaik, I. K., Aichele, C. P., and Bikkina, P. K. (2022). Microfluidics-based low salinity wettability alteration study of naphthenic-acid-adsorbed calcite surfaces. Energy & Fuels 36, 1842–1853. doi:10.1021/acs.energyfuels.1c03837
Shaik, I. K., Zhang, L., Pradhan, S., Kalkan, A. K., Aichele, C. P., and Bikkina, P. K. (2021). A parametric study of layer-by-layer deposition of caco3 on glass surfaces towards fabricating carbonate reservoirs on microfluidic chips. J. Petroleum Sci. Eng. 198, 108231. doi:10.1016/j.petrol.2020.108231Available at: https://www.sciencedirect.com/science/article/pii/S0920410520312857.
Shallan, A. I., Tavares, Y., Navvab Kashani, M., Breadmore, M. C., and Priest, C. (2021). An open microfluidic chip for continuous sampling of solute from a turbulent particle suspension. Angew. Chem. 133, 2686–2689. doi:10.1002/ange.202012410
Singh, R., Sivaguru, M., Fried, G. A., Fouke, B. W., Sanford, R. A., Carrera, M., et al. (2017). Real rock-microfluidic flow cell: a test bed for real-time in situ analysis of flow, transport, and reaction in a subsurface reactive transport environment. J. Contam. Hydrology 204, 28–39. doi:10.1016/j.jconhyd.2017.08.001Available at: https://www.sciencedirect.com/science/article/pii/S0169772217300931.
Song, W., de Haas, T. W., Fadaei, H., and Sinton, D. (2014). Chip-off-the-old-rock: the study of reservoir-relevant geological processes with real-rock micromodels. Lab a Chip 14, 4382–4390. doi:10.1039/C4LC00608A
Song, W., and Kovscek, A. R. (2015). Functionalization of micromodels with kaolinite for investigation of low salinity oil-recovery processes. Lab a Chip 15, 3314–3325. doi:10.1039/C5LC00544B
Song, W., Ogunbanwo, F., Steinsbø, M., Fernø, M. A., and Kovscek, A. R. (2018). Mechanisms of multiphase reactive flow using biogenically calcite-functionalized micromodels. Lab a Chip 18, 3881–3891. doi:10.1039/C8LC00793D
Stee, J. v., Depotter, M., Binnemans, K., and Gerven, T. V. (2022). Numbering-up liquid-liquid systems in microfluidic reactors: a parametric study. Chem. Eng. Res. Des. 184, 127–136. doi:10.1016/j.cherd.2022.05.028Available at: https://www.sciencedirect.com/science/article/pii/S0263876222002544.
Tang, Y., Valocchi, A. J., Werth, C. J., and Liu, H. (2013). An improved pore-scale biofilm model and comparison with a microfluidic flow cell experiment. Water Resour. Res. 49, 8370–8382. doi:10.1002/2013WR013843Available at: https://agupubs.onlinelibrary.wiley.com/doi/abs/10.1002/2013WR013843.
Vural Gürsel, I., Kurt, S. K., Aalders, J., Wang, Q., Noël, T., Nigam, K. D. P., et al. (2016). Utilization of milli-scale coiled flow inverter in combination with phase separator for continuous flow liquid–liquid extraction processes. Chem. Eng. J. 283, 855–868. doi:10.1016/j.cej.2015.08.028Available at: https://www.sciencedirect.com/science/article/pii/S1385894715010980.
Wang, L., and Li, P. C. H. (2011). Microfluidic DNA microarray analysis: a review. Anal. Chim. Acta 687, 12–27. doi:10.1016/j.aca.2010.11.056Available at: https://www.sciencedirect.com/science/article/pii/S0003267010014716.
Wang, W., Chang, S., and Gizzatov, A. (2017). Toward reservoir-on-a-chip: fabricating reservoir micromodels by in situ growing calcium carbonate nanocrystals in microfluidic channels. ACS Appl. Mater. & Interfaces 9, 29380–29386. doi:10.1021/acsami.7b10746
Wu, M., Xiao, F., Johnson-Paben, R. M., Retterer, S. T., Yin, X., and Neeves, K. B. (2012). Single- and two-phase flow in microfluidic porous media analogs based on voronoi tessellation. Lab a Chip 12, 253–261. doi:10.1039/C1LC20838A
Xu, W., Ok, J. T., Xiao, F., Neeves, K. B., and Yin, X. (2014). Effect of pore geometry and interfacial tension on water-oil displacement efficiency in oil-wet microfluidic porous media analogs. Phys. Fluids 26, 093102. doi:10.1063/1.4894071Available at: https://aip.scitation.org/doi/abs/10.1063/1.4894071.
Yang, D., Fan, R., Greet, C., and Priest, C. (2020). Microfluidic screening to study acid mine drainage. Environ. Sci. & Technol. 54, 14000–14006. doi:10.1021/acs.est.0c02901
Yang, D., Kirke, M., Fan, R., and Priest, C. (2019). Investigation of chalcopyrite leaching using an ore-on-a-chip. Anal. Chem. 91, 1557–1562. doi:10.1021/acs.analchem.8b04802
Yang, D., Navvab Kashani, M., and Priest, C. (2022). Pilot-scale microfluidic solvent extraction of high-value metals. Miner. Eng. 182, 107536. doi:10.1016/j.mineng.2022.107536Available at: https://www.sciencedirect.com/science/article/pii/S0892687522001467.
Yang, D., and Priest, C. (2018). Microfluidic platform for high-throughput screening of leach chemistry. Anal. Chem. 90, 8517–8522. doi:10.1021/acs.analchem.8b01413
Yang, D., Shallan, A. I., Breadmore, M. C., Greet, C., and Priest, C. (2023). Continuous monitoring of edta extractable iron from mineral slurries using a microfluidic chip. Can. J. Chem. Eng. 101, 944–952. doi:10.1002/cjce.24343
Yang, W., Lu, J., Wei, B., Yu, H., and Liang, T. (2021). Micromodel studies of surfactant flooding for enhanced oil recovery: a review. ACS Omega 6, 6064–6069. doi:10.1021/acsomega.0c05750
Yew, M., Ren, Y., Koh, K. S., Sun, C., and Snape, C. (2019). A review of state-of-the-art microfluidic technologies for environmental applications: detection and remediation. Glob. Challenges 3, 1800060. doi:10.1002/gch2.201800060
Yi, C., Li, C.-W., Ji, S., and Yang, M. (2006). Microfluidics technology for manipulation and analysis of biological cells. Anal. Chim. Acta 560, 1–23. doi:10.1016/j.aca.2005.12.037Available at: https://www.sciencedirect.com/science/article/pii/S0003267005020945.
Yin, C.-Y., Nikoloski, A. N., and Wang, M. (2013). Microfluidic solvent extraction of platinum and palladium from a chloride leach solution using alamine 336. Miner. Eng. 45, 18–21. doi:10.1016/j.mineng.2013.01.013Available at: https://www.sciencedirect.com/science/article/pii/S0892687513000150.
Yin, S., Chen, K., Srinivasakannan, C., Li, S., Zhou, J., Peng, J., et al. (2018). Microfluidic solvent extraction of ce (iii) and pr (iii) from a chloride solution using ehehpa (p507) in a serpentine microreactor. Hydrometallurgy 175, 266–272. doi:10.1016/j.hydromet.2017.12.009Available at:. https://www.sciencedirect.com/science/article/pii/S0304386X1730885X.
Yiotis, A., Karadimitriou, N. K., Zarikos, I., and Steeb, H. (2021). Pore-scale effects during the transition from capillary-to viscosity-dominated flow dynamics within microfluidic porous-like domains. Sci. Rep. 11, 3891. doi:10.1038/s41598-021-83065-8
Yoon, H., Valocchi, A. J., Werth, C. J., and Dewers, T. (2012). Pore-scale simulation of mixing-induced calcium carbonate precipitation and dissolution in a microfluidic pore network. Water Resour. Res. 48. doi:10.1029/2011WR011192Available at: https://agupubs.onlinelibrary.wiley.com/doi/abs/10.1029/2011WR011192.
Yun, W., Chang, S., Cogswell, D. A., Eichmann, S. L., Gizzatov, A., Thomas, G., et al. (2020). Toward reservoir-on-a-chip: rapid performance evaluation of enhanced oil recovery surfactants for carbonate reservoirs using a calcite-coated micromodel. Sci. Rep. 10, 782. doi:10.1038/s41598-020-57485-x
Zhang, C., Kang, Q., Wang, X., Zilles, J. L., Müller, R. H., and Werth, C. J. (2010). Effects of pore-scale heterogeneity and transverse mixing on bacterial growth in porous media. Environ. Sci. & Technol. 44, 3085–3092. doi:10.1021/es903396h
Zhang, D., Li, C., Ji, D., and Wang, Y. (2021). Paper-based microfluidic sensors for onsite environmental detection: a critical review. Crit. Rev. Anal. Chem. 52, 1432–1449. doi:10.1080/10408347.2021.1886900
Zhang, L.-h., Peng, J.-h., Ju, S.-h., Zhang, L.-b., Dai, L.-q., and Liu, N.-s. (2014). Microfluidic solvent extraction and separation of cobalt and nickel. RSC Adv. 4, 16081–16086. doi:10.1039/C4RA00458B
Keywords: microfluidics, lab-on-a-chip, process intensification, high throughput screening, mineral processing, extraction, leaching
Citation: Yang D and Priest C (2024) Industry relevant microfluidic platforms for mineral leaching experiments. Front. Chem. Eng. 6:1445900. doi: 10.3389/fceng.2024.1445900
Received: 08 June 2024; Accepted: 26 August 2024;
Published: 09 September 2024.
Edited by:
Volker Hessel, University of Adelaide, AustraliaReviewed by:
Evgeny Rebrov, University of Warwick, United KingdomLiangliang Lin, Jiangnan University, China
Copyright © 2024 Yang and Priest. This is an open-access article distributed under the terms of the Creative Commons Attribution License (CC BY). The use, distribution or reproduction in other forums is permitted, provided the original author(s) and the copyright owner(s) are credited and that the original publication in this journal is cited, in accordance with accepted academic practice. No use, distribution or reproduction is permitted which does not comply with these terms.
*Correspondence: Craig Priest, Y3JhaWcucHJpZXN0QHVuaXNhLmVkdS5hdQ==