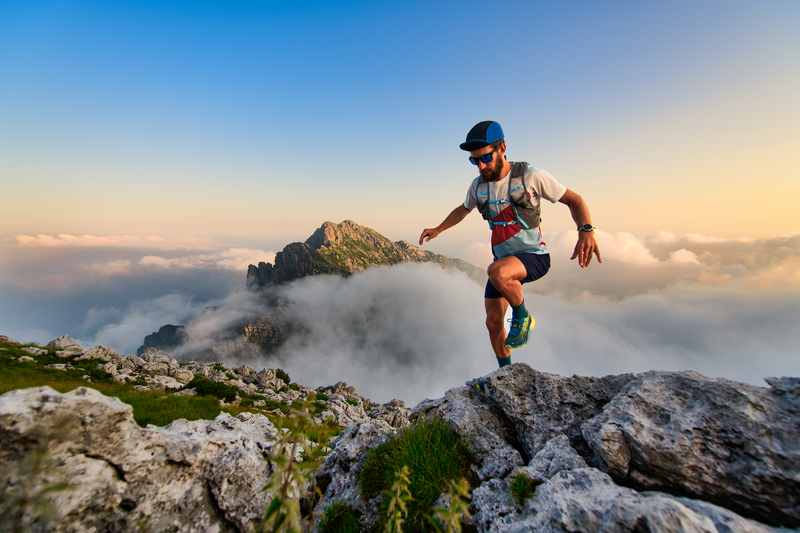
94% of researchers rate our articles as excellent or good
Learn more about the work of our research integrity team to safeguard the quality of each article we publish.
Find out more
REVIEW article
Front. Chem. Eng. , 08 August 2024
Sec. Separation Processes
Volume 6 - 2024 | https://doi.org/10.3389/fceng.2024.1430773
This article is part of the Research Topic Women in Separation Processes 2023 View all articles
Semiconductor photocatalysis, heterogeneous photo-Fenton and heterogeneous photocatalytic persulfate activation are light-driven advanced oxidation processes (AOPs) that have attracted much attention as promising technologies in wastewater treatment. Nevertheless, their large-scale application still faces several challenges, including the need to separate the catalyst from the treated water. In this regard, composite catalysts made up of semiconductor and magnetic materials have been understood as a potential alternative since the resulting magnetic photocatalysts can be easily separated from the medium by applying an external magnetic field. Interestingly, apart from facilitating the photocatalyst retrieval, the magnetic components could also be involved in the photocatalytic process. However, while the magnetic separation ability has been widely highlighted, other functions of the magnetic constituents remain obscure. This work, which covers the last 6 years of research, provides a comprehensive review on the magnetically assisted photocatalytic degradation of organic pollutants from water. Specifically, the magnetic photocatalysts that are commonly employed for that purpose are presented and the different roles of their magnetic constituents (roughly grouped as retrieval assistance, active catalyst, conduction medium or activator) are discussed. Furthermore, the importance of designing magnetic separators for attaining the photocatalyst retrieval is also emphasized. Collectively, this study could supply an avenue for developing magnetic photocatalysts that take advantage of both roles of magnetic materials, which can contribute to accomplish a more efficient pollutant degradation.
Global development and a better lifestyle come at the price of water pollution, which has emerged as a serious environmental issue. Particularly, the industrialization, rapid population growth and several anthropogenic activities (e.g., agriculture) have led to the discharge of pollutants in aquatic ecosystems, thus degrading the quality of water resources (Ahmadpour et al., 2020; Arman et al., 2021; Khosravani Goshtasb et al., 2022; Surana et al., 2022; Vale et al., 2022). A group of great interest is constituted by the so-called Contaminants of Emerging Concern (CECs), which comprise an extensive variety of micropollutants (including pharmaceuticals, personal care products, biocides, pesticides, herbicides, etc.); they have been detected in surface, ground and drinking water (Chávez et al., 2020; Tsaridou and Karabelas, 2021; Adeola et al., 2022). The adverse impacts that CECs pose on the environment and human health cause that their presence in water bodies raises concern worldwide. Hence, there is an urgent need to develop strategies to remove these pollutants from water (Cheng et al., 2021; Adeola et al., 2022; Morin-Crini et al., 2022).
Light-driven advanced oxidation processes (AOPs) have gained great recognition for that purpose; they constitute a family of technologies that are based on the production of highly reactive oxygen species, including hydroxyl radicals (•OH) and sulfate radicals (
In light of the concise overview of several AOPs previously mentioned, it is evident that most of the research on the catalytic degradation of pollutants has been carried out working with solid semiconductors suspended in the aqueous medium; therefore, after treatment, the catalysts must be efficiently separated from the liquid (He et al., 2019; Bielan et al., 2021). Nevertheless, the retrieval and recycling of the photocatalyst after the degradation process, which are of paramount importance for making the process cost-effective, represent an ongoing challenge that needs to be further explored (Iglesias et al., 2016; Abdel-Wahed et al., 2020; Bielan et al., 2021). Filtration and centrifugation have been used for the retrieval of the photocatalyst. However, the high cost and energy consumption of these additional operations call for the development of more efficient strategies (Wu et al., 2019; Lendzion-Bielun et al., 2020). If the photocatalytic material contains constituents of magnetic character, implementation of magnetic field-based separation provides a convenient avenue for accomplishing its successful retrieval (Bielan et al., 2021). Hence, compositing semiconductors with magnetic materials enables the successful retrieval of the resulting magnetic photocatalyst by applying external magnetic fields. Besides the advantageous separation and recovery of the magnetic photocatalyst, it has been reported that the magnetic component could participate in the photocatalytic degradation of pollutants, thus enhancing the photocatalyst’s performance (Lendzion-Bielun et al., 2020; Moradi et al., 2022). Given that the magnetic constituent could fulfill several roles (roughly grouped as retrieval assistance and photocatalytic), recognizing these functions is of utmost importance for designing and synthesizing more efficient photocatalysts. In a previous work of our research group (Gómez-Pastora et al., 2017a), the use of magnetic photocatalysts for water treatment was outlined and their promising possibilities for that application were highlighted. However, such study was mainly focused on the photocatalytic properties of these composites and their synthesis methods, thus the photocatalytic function of the magnetic materials was not investigated and the magnetic recovery of the photocatalyst was slightly addressed.
Motivated by this lack of knowledge, the present work aims at providing a comprehensive overview about the magnetically assisted photocatalytic degradation of organic pollutants from water. We specially focus on two key features of photocatalysis involving magnetic photocatalysts, namely, unveiling the possible roles of photocatalyst’s magnetic constituents (coarsely categorized as retrieval assistance and photocatalytic), and progressing in the efficient recovery of the photocatalyst after use. Firstly, photocatalysts whose magnetic component exclusively contributes to facilitate their retrieval are introduced. The following section provides insights into the photocatalytic functions that the magnetic materials could accomplish. Additionally, an overall picture of the magnetic recovery of the photocatalysts is given, with a special attention to the promising magnetic separators that could be used for that purpose. Finally, we close this work highlighting several key challenges and prospects of the performance and separation of magnetic photocatalysts. Overall, the present study could pave the way for the rational design of magnetic photocatalysts that apart from being easily collected after use demonstrate enhanced photocatalytic ability by benefiting from the photocatalytic roles of the magnetic materials.
To give an updated picture of the application of magnetic photocatalysts in water treatment, literature in the field of magnetic photocatalysts for CECs degradation was surveyed for the last 6 years (2019–2024). Particularly, studies that use magnetic photocatalysts for dyes or microplastics degradation, inactivation of bacteria, or sewage disinfection have not been considered; on the other hand, works focused on the removal of phenolic compounds have been included, since they are typically used as model pollutants. Additionally, only studies that offer valuable insight about magnetic photocatalysts have been analyzed. The selected studies following these criteria have been categorized in Tables 1–5 according to the different roles of the magnetic constituent of the photocatalysts, which have been schematized in Figure 1. From these Tables, it can be noted that iron oxides (typically magnetite, Fe3O4) and ferrites (whose general formula is AFe2O4, where A is a divalent metal cation) are the prime candidates for being used as magnetic materials in magnetic photocatalysts (Singh et al., 2019; Belessiotis et al., 2022; Grzegórska et al., 2023). Thereby, magnetite and ferrites make up the photocatalysts that are employed in 44% and 48% of the studies surveyed in this work, respectively, which may substantiate the wide applicability of these magnetic materials in the synthesis of magnetic photocatalysts. In the upcoming subsections, the most commonly used magnetic materials and their possible roles are presented and discussed. Particularly, the function of the photocatalyst’s magnetic components in the retrieval assistance is outlined in Section 2.1, whereas Section 2.2 elaborates on the different photocatalytic roles that these constituents could perform.
Table 1. Studies focused on the retrieval assistance role of the magnetic constituents of photocatalysts.
Figure 1. Schematic representation of the possible roles of the magnetic constituents of photocatalysts.
The primary focus of compositing photocatalysts with magnetic materials lies in facilitating their recovery after use. Table 1 presents the surveyed studies that solely exploit the retrieval assistance function of the photocatalyst’s magnetic constituents. Particularly, it encompasses studies that either do not demonstrate an enhancement in the pollutant removal with the inclusion of magnetic materials in the photocatalyst, or do not point out any photocatalytic role of the magnetic components.
According to Table 1, Fe3O4 is the predominant magnetic material used for photocatalyst retrieval in the vast majority of the research works. More specifically, about 67% of the selected studies employed Fe3O4 as magnetic source, while ∼22% of works have reported compositing photocatalysts with ferrites. Additionally, it can be noticed that TiO2 has been extensively utilized for degrading a wide variety of organic pollutants. This fact may derive from the appealing features of this photocatalysts, such as high photocatalytic activity and chemical stability, environmental friendliness, low-cost, corrosion resistance, and non-toxicity (Dharma et al., 2022; Sepahvand et al., 2022). When TiO2 serves as the photocatalytic component, the composite typically has a core-shell structure as deduced from Table 1, where the magnetic material constitutes the core and is encapsulated in a TiO2 shell. In most of these studies, a silicon dioxide (SiO2) interlayer is introduced between the magnetic core and TiO2 to prevent their interaction and assist the stability of the magnetic core (Khan et al., 2019a; Kumar et al., 2019; Sepahvand et al., 2022). An example of the core-interlayer-shell structure is shown in Figure 2A, where the coating of the magnetic core by a SiO2/TiO2 shell can be distinguished. Alternatively, the magnetic constituent can be loaded on the material surface. Figure 2B depicts this configuration, showing Fe3O4 nanoparticles growing on the surface of bentonite.
Figure 2. Images of photocatalysts where the magnetic constituent is located: (A) in the core (transmission electron microscopy, TEM); reprinted with permission from Dudziak et al. (2021), J. Environ. Chem. Eng (https://creativecommons.org/licenses/by/4.0/). or (B) deposited on the photocatalyst’s surface (scanning electron microscope, SEM); reprinted with permission from Kamali et al. (2022), Molecules, (Creative Commons Attribution, CC BY, license https://creativecommons.org/licenses/by/4.0/).
It is worth highlighting that for core-shell structures the thickness of the photocatalysts layer (shell) strongly impacts the efficiency of the photocatalytic and magnetic recovery stages. Specifically, the thickness of the photocatalyst layer should alter the diffusion layer, thus modifying the photocatalytic activity; moreover, the thicker the photocatalyst layer the worse magnetization of the material (Abdel-Wahed et al., 2020). In this regard, it is interesting to note that although the magnetic photocatalysts can be magnetically retrieved, they exhibit lower saturation magnetization than pure magnetite or ferrite, as evidenced in Table 1. Such magnetism reduction of the composite is caused by the presence of non-magnetic materials (Xu et al., 2021; Yilmaz et al., 2022). Hence, it is observed regardless of the type of photocatalytic semiconductor or the location of the magnetic material in the composite, that is in its core (for core-shell structures) or on the surface (when magnetic components are deposited on the material). For instance, Xu et al. (2021) found that the saturation magnetization (Ms) was drastically decreased when Fe3O4 (Ms = 85.9 emu/g) was composited with non-magnetic CdS and BiVO4 in the Fe3O4/BiVO4/CdS photocatalyst (Ms = 10.3 emu/g). Similarly, Kumar et al. (2019) reported that the coating of N-TiO2 and SiO2 on Fe3O4 resulted in the considerable reduction of the saturation magnetization of N-TiO2@SiO2@Fe3O4 (Ms = 8.69 emu/g) in comparison to pure Fe3O4 (Ms = 43.94 emu/g). Despite the evidenced magnetism reduction, Xu et al. (2021) demonstrated the successful retrieval of the photocatalyst by applying an external magnetic field. Thereby, although the reduction in the saturation magnetization does not hinder the magnetic separation of the photocatalyst, it involves an increase in the time required to fulfill such retrieval (Fuziki et al., 2021). Regarding the time to accomplish the magnetic recovery of the catalysts, Kumar et al. (2019) experimentally demonstrated that after 25 min the separation efficiency under a magnetic field was 97%, thus supporting the attractive possibilities of the magnetically facilitated recovery of photocatalysts.
Apart from having magnetic responsiveness, some of the photocatalysts gathered in Table 1 are also superparamagnetic. Particularly, Fung et al., 2019 and Kumar et al. (2019) highlighted the superparamagnetic behavior of their BiOBr0.9I0.1/Fe3O4@SiO2 and N-TiO2@SiO2@Fe3O4 photocatalysts, respectively. The superparamagnetic nature is revealed by their S-shaped magnetization curves, which are distinctive of superparamagnetic materials (Zimmermann, G. et al., 2003; Abdel-Wahed et al., 2020). Specifically, this type of materials exhibits low retentivity, and thus residual magnetic forces do not exist after removing the external magnetic field, which enables the quick redispersion of the photocatalyst once the magnetic field is ceased (Moniriyan et al., 2021; Xie et al., 2021). Superparamagnetism occurs in ferromagnetic and ferrimagnetic nanoparticles when their diameter falls below a critical threshold, which ranges from 3 to 50 nm depending on the material (Marghussian, 2015; Gómez-Pastora et al., 2017b). Superparamagnetic materials are attractive for water treatment applications due to the reversibility of their magnetic interactions. As a result, in absence of magnetic fields these materials can be redispersed in the aqueous media. Thereby, the agglomeration of the photocatalysts, which causes the reduction of its surface area, is prevented, thus demonstrating the possibility of being reused (Khan et al., 2019b; Abdel-Wahed et al., 2020; Zhou et al., 2020).
The features of magnetic photocatalysts outlined throughout this subsection support the promising prospects of integrating semiconductors with magnetic materials, since the resulting composite can be efficiently recovered by magnetic means. However, when designing magnetic photocatalysts it is crucial to take into account not only their easy recovery after use but also their efficiency to degrade the target pollutants. The photocatalyst’s magnetic constituents could have different effects on the contaminant removal. Thereby, they could either enhance the efficiency of photodegradation, which will be comprehensively discussed in the next section, or negatively affect it. In this regard, Smułek et al. (2021) and Lendzion-Bielun et al. (2020) conducted comparative studies on the ability of Fe3O4@SiO2-TiO2 and Fe3O4-TiO2, respectively, to degrade target contaminants against TiO2-P25. It is noteworthy that Smułek et al. (2021) and Lendzion-Bielun et al. (2020) synthesized the TiO2 of their composites, which could compromise the direct comparison with TiO2-P25 since they are different materials. Furthermore, the differences in the performance of the composite material could be also attributed to a lower catalyst concentration than that used for pristine catalyst. Despite the challenges for this comparison, Smułek et al. (2021) reported that while TiO2-P25 was able to almost completely remove nitrofurazone in 60 min, only 44% of the pollutant was degraded during the same time period using Fe3O4@SiO2-TiO2. On the other hand, Lendzion-Bielun et al. (2020) observed that 100% phenol conversion could be achieved using both TiO2-P25 and Fe3O4-TiO2. However, the former required 200 min and the later 300 min for achieving the same degradation. Although TiO2-P25 demonstrated an efficient performance, Smułek et al. (2021) and Lendzion-Bielun et al. (2020) concluded that the easy separation of magnetic photocatalysts from the treated media by applying magnetic fields supports the interest in their use for pollutant degradation. Hence, although pristine TiO2 could yield a higher conversion of the contaminant, its challenging recovery after use ultimately hinders its practical application.
Overall, the main interest in integrating semiconductors with magnetic materials lies in enhancing the recovery of the resulting composite after use. In this context, the attractive potential of the magnetically assisted photocatalysts recovery overcomes the possible reduction in degradation efficiency of magnetic photocatalysts. According to the studies herein surveyed, Fe3O4 stands out as the predominantly used magnetic material when the purpose of incorporating magnetic materials into the photocatalysts is solely to enhance their retrieval. In this regard, efficient magnetic separation has been demonstrated despite the lower saturation magnetization of the composite photocatalyst compared to the pure magnetic materials. Additionally, magnetic photocatalysts exhibiting a superparamagnetic behavior have been synthesized, which is especially attractive for the successful re-use of the photocatalysts after their magnetic retrieval.
As it has been widely highlighted in the literature, the introduction of magnetic components to the photocatalysts make it possible their easy separation from the treated medium. However, the magnetic constituents not only play a pivotal role in facilitating the recovery of the photocatalyst, but they could also be involved in the photodegradation of contaminants. Recognizing the different photocatalytic-based roles the magnetic materials that constitute the composite can play in the process may be of paramount interest for enhancing the efficiency of the degradation of pollutants. Therefore, in this section, the roughly classified as photocatalytic functions that the magnetic constituents can fulfill, which include (i) active catalyst, (ii) conduction medium, (iii) component of Fenton-like processes and (iv) persulfate (PS)/PMS activator, are presented and discussed. A general representation of these functions is depicted in Figure 3. It can be noted that for all roles the magnetic photocatalyst comprises at least one material of non-magnetic character (i.e., the semiconductor) and a magnetic material. The magnetic constituent can be involved in the formation of radicals through two different strategies. On the one hand, it can generate •OH or
Figure 3. Schematics of the photocatalytic roles of the magnetic constituents of photocatalysts: (A) active catalyst, (B) conduction medium, (C) component of Fenton-like processes, and (D) PS/PMS activator.
When the magnetic components that constitute the photocatalyst can be photoexcited to generate e− and h+ in their conduction (CB) and valence (VB) bands, respectively, and as a result the degradation efficiency is enhanced, they act as photocatalysts (Table 2). For instance, Wang et al. (2021), Zhao et al. (2022) and Ahmadpour et al. (2020) reported that ZnFe2O4 was able to yield degradation efficiencies exceeding 50% of the target pollutants; similarly, Sayadi et al., 2021 stated that CuFe2O4 was able to degrade 47% of gemfibrozil. According to Table 2, which includes the studies where the magnetic component acts as photocatalyst, ferrites are extensively used as magnetic materials. This observation may be in line with the exploitation of ferrite photocatalysts for the removal of organic pollutants that has been reported in the literature (Gupta et al., 2020). Particularly, ferrites have garnered interest as photocatalysts due to their narrow band gap, enabling them to harness visible light. Additionally, ferrites have a tunable band gap since depending on the ferrite (e.g., Zn-, Mn-, Co-ferrite, etc.) they exhibit a different band gap (<2.5 eV) and band positions (Ma and Liu, 2021; Cheng et al., 2023; Zheng et al., 2024).
Table 2. Studies focused on the active photocatalyst role of the magnetic constituents of photocatalysts.
Additionally, ferrites can be composited with semiconductor materials, which results in an improved photocatalytic performance (Casbeer et al., 2012; Kefeni and Mamba, 2020; Wu and Song, 2023). In this context, the magnetic materials generally reduce the band gap and/or inhibit the recombination of the photogenerated e−/h+ pairs mainly due to the formation of Z- or S-scheme heterojunctions with the semiconductors. Hence, the photocatalytic activity typically enhances when magnetic materials are introduced to the composite and work as photocatalysts. For instance, Wang et al. (2021) reported that the efficiency to degrade tetracycline hydrochloride upon visible light irradiation of the ZnFe2O4/Bi0-Bi2MoO6 composite they prepared was around 87%, which is considerably higher than that for pure Bi0-Bi2MoO6 (52%). They explained that the introduction of ZnFe2O4 decreases the band gap (from 2.32 eV for Bi0-Bi2MoO6 to 1.97 eV ZnFe2O4/Bi0-Bi2MoO6), broadens the spectral absorption range of the photocatalyst, and results in the formation of a Z-scheme heterojunction to facilitate the charge separation. Particularly, they suggested that both Bi2MoO6 and ZnFe2O4 where excited under visible light irradiation to produce e− and h+. The photogenerated e− of Bi2MoO6 could migrate to the nano-Bi0 and ZnFe2O4. Hence, nano-Bi0 and ZnFe2O4 can reduce O2 to
The magnetic components can also work as a conduction medium for the photogenerated e− so that they are effectively transferred between the semiconductors that constitute the photocatalytic composite. As noticed from Table 3, only Zhou et al. (2020) and Preeyanghaa et al. (2022) have raised this role of the photocatalyst’s magnetic constituent. More specifically, Zhou et al. (2020) and Preeyanghaa et al. (2022) reported that Fe3O4 can serve as an electron mediator to foster the building of Z-scheme heterojunctions. This role stems from the fact that Fe3+ and Fe2+ in Fe3O4 can act as recombination centers to capture photogenerated e− and h+ (Zhou et al., 2020). Particularly, Zhou et al. (2020) indicated that the following reactions could be involved when Fe3O4 acts as conduction medium:
Table 3. Studies focused on the conduction medium role of the magnetic constituents of photocatalysts.
In this context, Preeyanghaa et al. (2022) proposed a photocatalytic reaction mechanism for g-C3N4/BiOBr/Fe3O4 under simulated solar light irradiation that highlights the role of Fe3O4 as the conduction medium to transfer the CB e− of BiOBr to the heterojunction interface; subsequently, these e− are recombined with the VB h+ of g-C3N4. This way, the recombination of the photogenerated e−/h+ pairs in BiOBr and g-C3N4 is avoided, thus leading to the accumulation of h+ in the VB of BiOBr and e− in the CB of g-C3N4. These accumulated h+ and e− are involved in the production of •OH and
The Fe ions that constitute the magnetic materials can be also involved in Fenton reactions, leading to the synergistic degradation of contaminants due to photocatalytic and Fenton oxidation. Combining Fenton reaction with light irradiation results in photo-Fenton processes, which exhibit higher degradation rate compared to classical Fenton processes (Jiang Y. et al., 2022; Machado et al., 2023; Bule Možar et al., 2024). Particularly, light irradiation enhances the process performance by increasing the generation of •OH radicals through the decomposition of H2O2 and promoting the regeneration of Fe2+ ions (O’Dowd and Pillai, 2020; Ganiyu et al., 2022; Machado et al., 2023; Wu et al., 2023). Thereby, the photo-Fenton process involves the following reactions:
Table 4 collects studies that investigate the degradation of pollutants by coupling semiconductor photocatalysis with the photo-Fenton process involving the magnetic constituent of the photocatalysts. It can be noticed that while visible light is typically used in these systems, UVA irradiation has also been employed as the light source. Moreover, Fe3O4 is a magnetic material added in the composite when the Fenton-like process contributes to the pollutants degradation, together with photocatalysis. Although Fe3O4 is a traditional Fenton counterpart, photocatalysts containing ferrites, such as CoFe2O4, can also be involved in this process for generating ⋅OH radicals as it has been suggested (Preeyanghaa et al., 2022; Sepahvand et al., 2022). The use of magnetic photocatalyst in the photo-Fenton removal of contaminants was reported by Banic et al. (2019), who investigated the efficiency for degrading thiacloprid of WO3/Fe3O4 under simulated solar radiation in the presence and absence of H2O2. They found that the pollutant removal increased from 5% (WO3/Fe3O4) to 65% (WO3/Fe3O4/H2O2) when H2O2 was present. They reasoned that besides the influence of the coupling effect of WO3 and Fe3O4, the heterogeneous photo-Fenton process also affects the degradation efficiency. On a similar note, Sepahvand et al. (2022) proposed that the magnetic constituent of their CoFe2O4/TiO2/SiO2 photocatalyst could also take part in the degradation of 2,4-dinitrotoluene. Particularly, they proposed a mechanism, where apart from the photogenerated e− and h+ in TiO2, CoFe2O4 could be also involved in the generation of •OH radicals to degrade the pollutant by the reaction of their Fe2+ ions with H2O2. However, exploiting the Fenton-implication role of CoFe2O4 in the photocatalyst prepared by Sepahvand et al. (2022) may prove challenging due to its core-interlayer-shell configuration. More specifically, CoFe2O4 is coated by TiO2@SiO2 and constitutes the photocatalyst’s core. Hence, the photocatalyst’s magnetic constituent in the study of Sepahvand et al. (2022) is not in contact with H2O2. This fact contrasts with the work by Banic et al. (2019) where H2O2 was decomposed on the surface of the iron oxide. On the other hand, it should be emphasized that some studies presented in Table 4 examine how the solution pH impacts the degradation efficiency of pollutants. This investigation may derive from the fact that Fenton’s reactions are reported to be affected by the pH of the media, and thus, identifying the conditions that yield an optimum system performance is of utmost importance (Jung et al., 2009; Chen et al., 2023). For instance, Xue et al. (2024) explored the degradation of tetracycline using the Biochar/FeOOH photocatalysts at several pH values in the range from 3 to 11. They found that increasing the pH resulted in an enhancement of tetracycline degradation (92% for pH = 9 and 11, and ∼82% for pH = 3). Particularly, Xue et al. (2024) ascribed the diminished degradation efficiency under acidic conditions to the inhibition of the Fe2+/Fe3+ cycle by H+. Another key insight from the study by Xue et al. (2024) is ability of the magnetic component (FeOOH) to degrade the pollutant under light exposure when it is not composited with the biochar. Specifically, Xue et al. (2024) reported degradation efficiencies for FeOOH of 48% and 72% in absence and presence of H2O2, respectively. From Table 4, it can be noted that this ability for removing tetracycline is comparable to that reported by Kumar et al. (2022), who obtained 75% removal of tetracycline in a photo-Fenton process that uses in situ generated H2O2 and Ag/s-(Co3O4/NiFe2O4) as photocatalyst. The similar photodegradation ability of FeOOH compared to other photocatalysts evidences the potential of FeOOH to be used alone for the removal of target contaminants.
Table 4. Studies focused on the synergy between photocatalysis and Fenton-like oxidation due to the magnetic constituents of photocatalysts.
The above-mentioned photocatalytic-based roles of the magnetic materials are related to traditional AOPs relaying on •OH radicals. However, AOPs based on
On the other hand, PMS activation through Fe could undergo reactions 10 and 11, which are analogous for other transition metals such as Mn and Co (Kohantorabi et al., 2021; Grzegórska et al., 2023). It is worth pointing out that although these reactions do not directly involve light they have been here included since transition metals constitute the photocatalyst, and thus the reactions can occur in the photocatalytic system.
In this context, the activation of PMS or PS coupled to semiconductor photocatalysis has been reported (Table 5). For instance Grzegórska et al. (2023), Moradi et al. (2022), and Kohantorabi et al. (2021) proposed a mechanism for the photocatalytic degradation of a different contaminant over TiO2/Ti3C2/MnFe2O4/PMS, SCF/g-C3N4/PS/UVC, Fe3O4/CeO2/BiOI/UVA/PMS processes, respectively. Particularly, Grzegórska et al. (2023) posed that PMS could be activated by Mn and Fe in their system and determined the rate-limiting steps in the PMS activation process. On the other hand, Moradi et al. (2022), and Kohantorabi et al. (2021) suggested that apart from the Fe ions, the photogenerated e− can also activate PS and PMS, respectively, to generate
Collectively, the incorporation of magnetic materials to the photocatalyst not only benefits the retrieval of the composite from the treated medium but could also enhance the photocatalytic degradation of contaminants. Specifically, the magnetic materials can play different roles, namely, active catalyst, PS/PMS activator, conduction medium and activation of Fenton-like oxidation, apart from assisting the magnetic recovery of the photocatalyst as it has been discussed throughout this section. It is worth mentioning that the magnetic constituent can exclusively act as photocatalyst, as proposed, for instance in the works of Huang et al. (2023), Jiang X. et al. (2022), Wang et al. (2021), and Karbasi et al. (2019). Alternatively, it can combine this function with its role as PMS activator or component of the Fenton-like oxidation as suggested by Tian et al. (2023), Moradi et al. (2022), and Banic et al. (2019), to name a few.
Throughout the previous sections the attractive prospects of using magnetic photocatalysts for degrading organic pollutants have been comprehensively outlined. It is noteworthy that although integrating semiconductors and magnetic components could enhance the photocatalytic performance, the primary focus of compositing these materials lies in facilitating their recovery after use. Nevertheless, from the numerous works surveyed herein, only a limiting few have assessed the ability of magnetic photocatalysts for being magnetically recovered. This preliminary test is typically based on placing a permanent magnet next to a vial containing a sample of the suspended magnetic photocatalysts; after a certain time period, a clear solution, with the particles retained in the vial wall close to the magnet is obtained (Fung et al., 2019; Wang et al., 2021; Grzegórska et al., 2023). While this methodology enables ascertaining whether the photocatalyst can be magnetically retrieved, the time required for recovering the magnetic photocatalyst from the entire volume of the medium, which is crucial for determining the efficiency of the magnetic recovery stage, is not estimated. On a similar note, the design of magnetic separators for addressing the recovery of magnetic photocatalysts remains an area that has received limited attention. Particularly, only Kumar et al. (2019) and Fernández et al. (2019) have addressed both the recovery of the composites from the total volume of the medium and the use of a specific magnetic separation system to perform such retrieval. Specifically, Kumar et al. (2019) employed an electromagnetic separation unit, where the retrieval of the composites due to the action of the applied electromagnetic field (∼200 mT) was accomplished. Additionally, they also determined the efficiency of the electromagnetic separation unit for retrieving the composite as a function of time by measuring the turbidity of supernatant samples taken at different times. Fernández et al. (2019) utilized a magnetic photocatalytic reactor to carry out the photodegradation of the pollutants followed by the magnetic retrieval of the photocatalysts. The magnetic separation unit features an alternate polarity magnetic bar, where the magnetic photocatalyst was trapped after use. Thereby, the research conducted by Kumar et al. (2019) and Fernández et al. (2019) provide a more realistic picture about the use of magnetic photocatalysts by integrating the photocatalytic degradation step and the subsequent magnetic recovery.
Although magnetic separators have not been typically used in the studies covered by the present work, the efficiency of several systems for retrieving magnetic materials has been evidenced in existing literature. For instance, high gradient magnetic separators (HGMSs) have been applied in several fields for the separation of magnetic solids, including wastewater treatment (Baik et al., 2012; Gómez-Pastora et al., 2017a, Gómez-Pastora et al., 2017b; Kheshti et al., 2019). Briefly, HGMSs comprise batch filters filled with ferromagnetic filaments (Figure 4A) and can use different sources for generating the magnetic field. When an external magnetic field is applied, these filters trap the magnetic materials, thus obtaining a clean solution; subsequently, the magnetic materials can be recovered from the HGMSs by switching off the magnetic field. Despite the high efficiency provided by HGMSs, they pose several drawbacks, such as the undesired entrapment of non-magnetic solids or the possible particle aggregation, which could limit their practical application (Leong et al., 2016; Gómez-Pastora et al., 2017b). Another alternative for addressing the retrieval of magnetic materials consists of the use of open gradient magnetic separators (OGMSs), which are not based on ferromagnetic matrices. In OGMSs, magnetic fields are generated by magnets conveniently arranged around the separator walls. OGMSs provide high separation efficacy; however, their lower magnetic gradients compared to HGMSs represent an important drawback. In this regard, magnetic gradients may be considerably increased by using powerful magnets in quadrupole configuration, which is one of the most common magnet arrangements. Particularly, in the continuous OGMSs with the quadrupole magnet configuration, magnetic materials and the clean solution are obtained at different outlets of the system under a magnetic field as shown in Figure 4B (Gómez-Pastora et al., 2017a; Gómez-Pastora et al., 2017b).
Figure 4. Schematic diagram of (A) HGMS, and (B) OGMS with the quadrupole magnetic configuration (Adapted from González-Fernández et al. (2021), Ind. Eng. Chem. Res. https://creativecommons.org/licenses/by/4.0/). (C) Example of velocity fields predicted by CFD simulations in a serpentine channel; Reprinted from Saif et al. (2024), Desalination (https://creativecommons.org/licenses/by-nc-nd/4.0/).
The design of magnetic separators requires understanding the physics underlying photocatalysts retrieval. Particularly, magnetic separation is driven by the magnetic force exerted on magnetic photocatalysts (Fm), which is described by the following expression (Furlani, 2006; Furlani, 2010):
where, µ0 denotes the permeability of free space, Vp and Mp stand for the particle volume and magnetization, and Ha represents the applied magnetic field intensity (Furlani, 2006, Furlani, 2010).
According to the Eq. 12, Fm is proportional to the volume and saturation magnetization of the photocatalytic particle. Hence, magnetic recovery is favored for large particles. However, increasing the particle size causes the reduction of its surface to volume ratio, thus negatively affecting the efficacy of the photocatalyst. On the other hand, the higher the particle saturation magnetization the greater the Fm that promotes its retrieval from the treated solution. Nevertheless, the saturation magnetization decreases when the magnetic constituent is compound with semiconductors, as it has been previously discussed (Gómez-Pastora et al., 2017b; Torrejon et al., 2020). In this context, it becomes evident that a trade-off between the factors influencing photocatalytic degradation and magnetic separation should be achieved so that both of them could be optimized. Additionally, desired magnetic separators will ensure complete retrieval of photocatalyst while providing high-volumetric throughput. To design systems that fulfill these requirements, simulation software could be employed, since they can provide insight into the magnetic and hydrodynamic conditions in the separators (Figure 4C). In this regard, computational fluid dynamics (CFD) techniques enable modeling the separation process prior to the system fabrication, and their potential for contributing to the design of magnetic separators have been already demonstrated. While CFD offers numerous benefits, it is important to recognize that the computational demand of the simulations could limit the complexity of the separators to be simulated (Gómez-Pastora et al., 2017b; González Fernández et al., 2020; González-Fernández et al., 2021).
Magnetic photocatalysts have gained recognition as potential agents to assist the photodegradation of organic pollutants. Thereby, the integration of semiconductors with magnetic materials enables surmounting a critical challenge in photocatalysis, namely the post-use recovery of the photocatalyst. Additionally, magnetic photocatalysts have been acknowledged for their possibilities to enhance the photocatalytic performance through different functions. However, despite the promising prospects of magnetic photocatalysts, there are still open questions that need to be addressed so that these composites could be applied in large scale water treatment process. These issues pertain to the two main steps of photocatalytic processes, which include the efficient degradation of target pollutants and the subsequent retrieval of the photocatalyst.
Regarding the pollutants removal step, it is crucial to enhance to a great extent the photocatalyst’s performance so that the complete degradation of the target contaminants is efficiently accomplished. In this context, thorough research is essential to confirm and provide evidence for the possible functions of the photocatalyst’s magnetic constituents, since these photocatalytic roles derive from the photocatalytic mechanism proposed in the surveyed studies. Additionally, to deepen the understanding of how magnetic materials contribute to enhance photodegradation, it is necessary to evaluate the photocatalyst’s effectiveness both with and without the magnetic components, and compare the performance of photocatalysts comprising comparable concentrations of magnetic constituents. The absence of such comparative analysis in many studies listed in Tables 1–5 limits elucidating the impact of the magnetic component. The aforementioned insights could have the potential to significantly improve the photodegradation of pollutants by exploiting the photocatalytic roles of the magnetic components. In addition to better understanding the role of the magnetic component in photocatalysis, improving photocatalytic efficiency also requires assessing photocatalytic performance under conditions that closely resemble real environmental scenarios. In this regard, the lack of correlation of the photocatalysts efficiency in well-controlled lab conditions and in real-world applications has been reported to greatly contribute to the scarce use of photocatalysis in the latter (Bortolotto et al., 2022). Exploring the photocatalyst’s efficiency in a medium constituted of a mixture of pollutants, rather than a single one could contribute to bridge the gap between the experimental and real-world conditions encountered in photocatalysis. Finally, advancing in the design of magnetic photocatalysts also requires assessing their performance for pollutant mineralization and monitoring the potential metal leaching during their use. Leaching measurements in several studies revealed a release of metal ions typically lower than 4.6 mg/L, suggesting that there are no significant safety hazards to the aquatic environment. However, total organic carbon (TOC) analysis showed that target contaminants were not fully mineralized into CO2, but TOC yield of removal lower than 70% are normally obtained. Despite the importance of conducting leaching and TOC analyses, they have only been carried out in some works included in Tables 1–5. Hence, future research should incorporate these analyses to enhance the performance of novel magnetic photocatalysts.
Regarding the magnetic retrieval of the photocatalysts, the practical use of magnetic photocatalysts also requires the implementation of the magnetic separation stage, as well as its integration with the previous photocatalytic process. From this perspective, it is vitally important to demonstrate efficient recovery of magnetic photocatalysts that extends beyond the small-scale proof-of-concept assays reported in most studies. Hence, research efforts should be devoted to the design and optimization of magnetic separators for addressing the efficient recovery of the magnetic photocatalysts after use. More specifically, there is an urgent need for developing magnetic separators that ensure complete photocatalyst retrieval while providing high-volume throughput. CFD techniques can guide the enhancement of the systems performance through the strategic refinement of different features, including the separator geometry or the applied magnetic fields and gradients (Gómez-Pastora et al., 2017b; González Fernández et al., 2020; González-Fernández et al., 2021).
Overall, this study offers a comprehensive overview of the potential of magnetic photocatalysts for the oxidation of organic pollutants from aquatic environments. Particularly, we have reviewed articles that cover the last 6 years of research to underscore the dual role of magnetic photocatalysts. Thereby, in addition to emphasizing the ease retrieval of magnetic photocatalysts by applying magnetic fields, a challenge that has received considerable attention, this study also focuses on the less examined photocatalytic roles of the photocatalyst’s magnetic constituents. Additionally, we have highlighted the importance of further research to advance the use of magnetic photocatalysts as outstanding materials in real applications. Collectively, this review envisages great hopes for the exploitation of the magnetically assisted photocatalysis for degradation of organic pollutants, which may stimulate further progress on this field and its exploitation for a broader range of photocatalytic processes beyond the removal of contaminants.
CG-F: Conceptualization, Formal Analysis, Investigation, Writing–original draft. EB: Funding acquisition, Methodology, Visualization, Writing–review and editing. MR: Conceptualization, Funding acquisition, Supervision, Writing–review and editing. IO: Funding acquisition, Project administration, Resources, Supervision, Writing–review and editing.
The author(s) declare that financial support was received for the research, authorship, and/or publication of this article. Financial support from the project PID2021-122563OB-I00 funded by MICIU/AEI/10.13039/501100011033 and by ERDF/EU and Grant PLEC2021-007718 funded by MICIU/AEI/10.13039/501100011033 and by the European Union NextGenerationEU/PRTR are gratefully acknowledged. CG-F also thanks the Spanish Ministry of Universities for the Margarita Salas postdoctoral fellowship (grants for the requalification of the Spanish university system for 2021–2023, University of Cantabria), funded by the European Union-NextGenerationEU.
The authors declare that the research was conducted in the absence of any commercial or financial relationships that could be construed as a potential conflict of interest.
All claims expressed in this article are solely those of the authors and do not necessarily represent those of their affiliated organizations, or those of the publisher, the editors and the reviewers. Any product that may be evaluated in this article, or claim that may be made by its manufacturer, is not guaranteed or endorsed by the publisher.
Abdel-Wahed, M. S., El-Kalliny, A. S., Badawy, M. I., Attia, M. S., and Gad-Allah, T. A. (2020). Core double-shell MnFe2O4@rGO@TiO2 superparamagnetic photocatalyst for wastewater treatment under solar light. Chem. Eng. J. 382, 122936. doi:10.1016/j.cej.2019.122936
Adeola, A. O., Abiodun, B. A., Adenuga, D. O., and Nomngongo, P. N. (2022). Adsorptive and photocatalytic remediation of hazardous organic chemical pollutants in aqueous medium: a review. J. Contam. Hydrol. 248, 104019. doi:10.1016/j.jconhyd.2022.104019
Ahmadpour, N., Sayadi, M. H., Sobhani, S., and Hajiani, M. (2020). Photocatalytic degradation of model pharmaceutical pollutant by novel magnetic TiO2@ZnFe2O4/Pd nanocomposite with enhanced photocatalytic activity and stability under solar light irradiation. J. Environ. Manage. 271, 110964. doi:10.1016/j.jenvman.2020.110964
Alvarez-Aguiñaga, E. A., Elizalde-González, M. P., García-Díaz, E., and Sabinas-Hernández, S. A. (2022). UV-light-driven conversion of gadoterate meglumine: insight into the photocatalyst’s influence on conversion pathway, transformation products, and release of toxic ionic gadolinium. Catal. Commun. 172, 106544. doi:10.1016/j.catcom.2022.106544
Arman, N. Z., Salmiati, S., Aris, A., Salim, M. R., Nazifa, T. H., Muhamad, M. S., et al. (2021). A review on emerging pollutants in the water environment: existences, health effects and treatment processes. Water 13, 3258. doi:10.3390/w13223258
Bahmani, M., Dashtian, K., Mowla, D., Esmaeilzadeh, F., and Ghaedi, M. (2020). UiO-66(Ti)-Fe3O4-WO3 photocatalyst for efficient ammonia degradation from wastewater into continuous flow-loop thin film slurry flat-plate photoreactor. J. Hazard. Mater. 393, 122360. doi:10.1016/j.jhazmat.2020.122360
Baik, S. K., Ha, D. W., Ko, R. K., and Kwon, J. M. (2012). Magnetic field analysis of high gradient magnetic separator via finite element analysis. Phys. C Supercond. its Appl. 480, 111–117. doi:10.1016/j.physc.2012.04.036
Banic, N. D., Abramovic, B. F., Krstic, J. B., Šojiic Merkulova, D. V., Fincur, N. L., and Mitric, M. N. (2019). Novel WO3/Fe3O4 magnetic photocatalysts: preparation, characterization and thiacloprid photodegradation. J. Ind. Eng. Chem. 70, 264–275. doi:10.1016/j.jiec.2018.10.025
Behnajady, M. A., Hajiahmadi, M., and Modirshahla, N. (2012). Enhancement of removal rate of an organic pollutant in the presence of immobilized TiO2 nanoparticles with inorganic anions combination: optimization using Taguchi approach. Ind. Eng. Chem. Res. 51, 15324–15330. doi:10.1021/ie301521z
Belessiotis, G. V., Falara, P. P., Ibrahim, I., and Kontos, A. G. (2022). Magnetic metal oxide-based photocatalysts with integrated silver for water treatment. Materials 15, 4629. doi:10.3390/ma15134629
Bhatkhande, D. S., Pangarkar, V. G., and Beenackers, A. A. C. M. (2002). Photocatalytic degradation for environmental applications - a review. J. Chem. Technol. Biotechnol. 77, 102–116. doi:10.1002/jctb.532
Bielan, Z., Kowalska, E., Dudziak, S., Wang, K., Ohtani, B., and Zielińska-Jurek, A. (2021). Mono- and bimetallic (Pt/Cu) titanium(IV) oxide core–shell photocatalysts with UV/Vis light activity and magnetic separability. Catal. Today 361, 198–209. doi:10.1016/j.cattod.2020.05.034
Bielan, Z., Sulowska, A., Dudziak, S., Siuzdak, K., Ryl, J., and Zielińska-Jurek, A. (2020). Defective TiO2 core-shell magnetic photocatalyst modified with plasmonic nanoparticles for visible light-induced photocatalytic activity. Catalysts 10, 672. doi:10.3390/catal10060672
Bortolotto, V., Djellabi, R., Giordana, A., Cerrato, G., Di Michele, A., and Bianchi, C. L. (2022). Photocatalytic behaviour of Ag3PO4, Fe3O4 and Ag3PO4/Fe3O4 heterojunction towards the removal of organic pollutants and Cr (VI) from water: efficiency and light-corrosion deactivation. Inorg. Chem. Commun. 141, 109516. doi:10.1016/j.inoche.2022.109516
Bule Možar, K., Miloloža, M., Martinjak, V., Radovanović-Perić, F., Bafti, A., Ujević Bošnjak, M., et al. (2024). Evaluation of Fenton, photo-fenton and fenton-like processes in degradation of PE, PP, and PVC microplastics. WaterSwitzerl. 16, 673. doi:10.3390/w16050673
Casbeer, E., Sharma, V. K., and Li, X. Z. (2012). Synthesis and photocatalytic activity of ferrites under visible light: a review. Sep. Purif. Technol. 87, 1–14. doi:10.1016/j.seppur.2011.11.034
Chávez, A. M., Quiñones, D. H., Rey, A., Beltrán, F. J., and Álvarez, P. M. (2020). Simulated solar photocatalytic ozonation of contaminants of emerging concern and effluent organic matter in secondary effluents by a reusable magnetic catalyst. Chem. Eng. J. 398, 125642. doi:10.1016/j.cej.2020.125642
Chen, Z., Yan, Y., Lu, C., Lin, X., Fu, Z., Shi, W., et al. (2023). Photocatalytic self-fenton system of g-C3N4-based for degradation of emerging contaminants: a review of advances and prospects. Molecules 28, 5916. doi:10.3390/molecules28155916
Chen, Z. Y., Lai, W. W. P., Lin, H. H. H., Tan, J. X., Wu, K. C. W., and Lin, A. Y. C. (2022). Photocatalytic degradation of ketamine using a reusable TiO2/SiO2@Fe3O4 magnetic photocatalyst under simulated solar light. J. Environ. Chem. Eng. 10, 108637. doi:10.1016/j.jece.2022.108637
Cheng, N., Wang, B., Wu, P., Lee, X., Xing, Y., Chen, M., et al. (2021). Adsorption of emerging contaminants from water and wastewater by modified biochar: a review. Environ. Pollut. 273, 116448. doi:10.1016/j.envpol.2021.116448
Cheng, Y., Zhang, S., Wang, Z., Wang, B., You, J., Guo, R., et al. (2023). Review on spinel ferrites-based materials (MFe2O4) as photo-Fenton catalysts for degradation of organic pollutants. Sep. Purif. Technol. 318, 123971. doi:10.1016/j.seppur.2023.123971
Dewil, R., Mantzavinos, D., Poulios, I., and Rodrigo, M. A. (2017). New perspectives for advanced oxidation processes. J. Environ. Manage. 195, 93–99. doi:10.1016/j.jenvman.2017.04.010
Dharma, H. N. C., Jaafar, J., Widiastuti, N., Matsuyama, H., Rajabsadeh, S., Othman, M. H. D., et al. (2022). A review of titanium dioxide (TiO2)-based photocatalyst for oilfield-produced water treatment. Membranes 12, 345. doi:10.3390/membranes12030345
do Carmo Batista, W. V. F., da Cunha, R., dos Santos, A. C., dos Reis, P. M., Furtado, C. A., Silva, M. C., et al. (2022). Synthesis of a reusable magnetic photocatalyst based on carbon xerogel/TiO2 composites and its application on acetaminophen degradation. Ceram. Int. 48, 34395–34404. doi:10.1016/j.ceramint.2022.08.018
Dudziak, S., Bielan, Z., Kubica, P., and Zielinska-Jurek, A. (2021). Optimization of carbamazepine photodegradation on defective TiO2-based magnetic photocatalyst. J. Environ. Chem. Eng. 9, 105782. doi:10.1016/j.jece.2021.105782
Feng, S., Xie, T., Wang, J., Yang, J., Kong, D., Liu, C., et al. (2023). Photocatalytic activation of PMS over magnetic heterojunction photocatalyst SrTiO3/BaFe12O19 for tetracycline ultrafast degradation. Chem. Eng. J. 470, 143900. doi:10.1016/j.cej.2023.143900
Fernández, L., Gamallo, M., González-Gómez, M. A., Vázquez-Vázquez, C., Rivas, J., Pintado, M., et al. (2019). Insight into antibiotics removal: exploring the photocatalytic performance of a Fe3O4/ZnO nanocomposite in a novel magnetic sequential batch reactor. J. Environ. Manage. 237, 595–608. doi:10.1016/j.jenvman.2019.02.089
Fung, C. S. L., Khan, M., Kumar, A., and Lo, I. M. C. (2019). Visible-light-driven photocatalytic removal of PPCPs using magnetically separable bismuth oxybromo-iodide solid solutions: mechanisms, pathways, and reusability in real sewage. Sep. Purif. Technol. 216, 102–114. doi:10.1016/j.seppur.2019.01.077
Furlani, E. P. (2006). Analysis of particle transport in a magnetophoretic microsystem. J. Appl. Phys. 99. doi:10.1063/1.2164531
Furlani, E. P. (2010). Magnetic biotransport: analysis and applications. Materials 3, 2412–2446. doi:10.3390/ma3042412
Fuziki, M. E. K., Brackmann, R., Dias, D. T., Tusset, A. M., Specchia, S., and Lenzi, G. G. (2021). Effects of synthesis parameters on the properties and photocatalytic activity of the magnetic catalyst TiO2/CoFe2O4 applied to selenium photoreduction. J. Water Process Eng. 42, 102163. doi:10.1016/j.jwpe.2021.102163
Ganiyu, S. O., Nidheesh, P. V., and Oturan, M. A. (2022). “Synthesis and application of nanostructured iron oxides heterogeneous catalysts for environmental applications,” in Advanced materials for sustainable environmental remediation: terrestrial and aquatic environments, 583–608.
Ghobadifard, M., Mohebbi, S., and Radovanovic, P. V. (2020). Selective oxidation of alcohols by using CoFe2O4/Ag2MoO4 as a visible-light-driven heterogeneous photocatalyst. New J. Chem. 44, 2858–2867. doi:10.1039/c9nj05633e
Gómez-Pastora, J., Dominguez, S., Bringas, E., Rivero, M. J., Ortiz, I., and Dionysiou, D. D. (2017a). Review and perspectives on the use of magnetic nanophotocatalysts (MNPCs) in water treatment. Chem. Eng. J. 310, 407–427. doi:10.1016/j.cej.2016.04.140
Gómez-Pastora, J., Xue, X., Karampelas, I. H., Bringas, E., Furlani, E. P., and Ortiz, I. (2017b). Analysis of separators for magnetic beads recovery: from large systems to multifunctional microdevices. Sep. Purif. Technol. 172, 16–31. doi:10.1016/j.seppur.2016.07.050
González Fernández, C., Gómez Pastora, J., Basauri, A., Fallanza, M., Bringas, E., Chalmers, J. J., et al. (2020). Continuous-flow separation of magnetic particles from biofluids: how does the microdevice geometry determine the separation performance? Sensors 20, 3030. doi:10.3390/s20113030
González-Fernández, C., Gómez-Pastora, J., Bringas, E., Zborowski, M., Chalmers, J. J., and Ortiz, I. (2021). Recovery of magnetic catalysts: advanced design for process intensification. Ind. Eng. Chem. Res. 60, 16780–16790. doi:10.1021/acs.iecr.1c03474
González-Rodríguez, J., Conde, J. J., Vargas-Osorio, Z., Vázquez-Vázquez, C., Piñeiro, Y., Rivas, J., et al. (2024). LED-driven photo-Fenton process for micropollutant removal by nanostructured magnetite anchored in mesoporous silica. J. Environ. Manage. 349, 119461. doi:10.1016/j.jenvman.2023.119461
Grzegórska, A., Ofoegbu, J. C., Cervera-Gabalda, L., Gómez-Polo, C., Sannino, D., and Zielińska-Jurek, A. (2023). Magnetically recyclable TiO2/MXene/MnFe2O4 photocatalyst for enhanced peroxymonosulphate-assisted photocatalytic degradation of carbamazepine and ibuprofen under simulated solar light. J. Environ. Chem. Eng. 11, 110660. doi:10.1016/j.jece.2023.110660
Guo, R., Xi, B., Guo, C., Liu, W., Lv, N., and Xu, J. (2022). Comprehensive insight into heterogeneous persulfate activation for environmental pollutants degradation: approaches and mechanism. Environ. Funct. Mater. 1, 239–252. doi:10.1016/j.efmat.2022.12.001
Gupta, N. K., Ghaffari, Y., Kim, S., Bae, J., Kim, K. S., and Saifuddin, M. (2020). Photocatalytic degradation of organic pollutants over MFe2O4 (M = Co, Ni, Cu, Zn) nanoparticles at neutral pH. Sci. Rep. 10, 4942. doi:10.1038/s41598-020-61930-2
Han, Y., Wang, S., Yu, X., Li, M., Yi, Z., Tang, J., et al. (2023). Ba0.5Sr0.5TiO3/BaFe12O19 magnetic separation photocatalysts: structural, optical, microstructure, photocatalytic activity, degradation pathway and mechanism. Ceram. Int. 49, 37967–37982. doi:10.1016/j.ceramint.2023.09.126
Hao, Y., Yang, H., Jiang, S., Komarneni, S., and Ma, J. (2024). MOF-derived CoFe2O4/FeS2 Z-type heterojunctions for efficient degradation of tetracycline by visible-light activation of persulfate. Ceram. Int. 50, 25365–25378. doi:10.1016/j.ceramint.2024.04.268
He, J., Zeng, X., Lan, S., and Lo, I. M. C. (2019). Reusable magnetic Ag/Fe, N-TiO2/Fe3O4@SiO2 composite for simultaneous photocatalytic disinfection of E. coli and degradation of bisphenol A in sewage under visible light. Chemosphere 217, 869–878. doi:10.1016/j.chemosphere.2018.11.072
Huang, H., Tao, X., Niu, Z., Qin, X., Ren, J., Shan, B., et al. (2023). Construction of magnetically recoverable MnZnFe2O4@Ag3PO4 Z-scheme photocatalyst for rapid visible-light-driven phenol degradation. Environ. Sci. Pollut. Res. 30, 32095–32107. doi:10.1007/s11356-022-24479-3
Iglesias, O., Rivero, M. J., Urtiaga, A. M., and Ortiz, I. (2016). Membrane-based photocatalytic systems for process intensification. Chem. Eng. J. 305, 136–148. doi:10.1016/j.cej.2016.01.047
Jabbar, Z. H., Graimed, B. H., Alsunbuli, M. M., and Sabit, D. A. (2023). Developing a magnetic bismuth-based quaternary semiconductor boosted by plasmonic action for photocatalytic detoxification of Cr(VI) and norfloxacin antibiotic under simulated solar irradiation: synergistic work and radical mechanism. J. Alloys Compd. 958, 170521. doi:10.1016/j.jallcom.2023.170521
Jiang, X., Wang, M., Luo, B., Yang, Z., Li, W., Zhang, D., et al. (2022). Magnetically recoverable flower-like Sn3O4/SnFe2O4 as a type-II heterojunction photocatalyst for efficient degradation of ciprofloxacin. J. Alloys Compd. 926, 166878. doi:10.1016/j.jallcom.2022.166878
Jiang, Y., Ran, J., Mao, K., Yang, X., Zhong, L., Yang, C., et al. (2022). Recent progress in Fenton/Fenton-like reactions for the removal of antibiotics in aqueous environments. Ecotoxicol. Environ. Saf. 236, 113464. doi:10.1016/j.ecoenv.2022.113464
Jung, Y. S., Lim, W. T., Park, J. Y., and Kim, Y. H. (2009). Effect of pH on Fenton and fenton-like oxidation. Environ. Technol. 30, 183–190. doi:10.1080/09593330802468848
Kamali, M., Xue, Y., Khalaj, M., Laats, B., Teunckens, R., Verbist, M., et al. (2022). ZnO/γ-Fe2O3/Bentonite: an efficient solar-light active magnetic photocatalyst for the degradation of pharmaceutical active compounds. Molecules 27, 3050. doi:10.3390/molecules27103050
Karbasi, M., Karimzadeh, F., Kiwi, J., Raeissi, K., Pulgarin, C., and Rtimi, S. (2019). Flower-like magnetized photocatalysts accelerating an emerging pollutant removal under indoor visible light and related phenomena. J. Photochem. Photobiol. A Chem. 378, 105–113. doi:10.1016/j.jphotochem.2019.04.017
Kefeni, K. K., and Mamba, B. B. (2020). Photocatalytic application of spinel ferrite nanoparticles and nanocomposites in wastewater treatment: review. Sustain. Mater. Technol. 23, e00140. doi:10.1016/j.susmat.2019.e00140
Khan, M., Fung, C. S. L., Kumar, A., He, J., and Lo, I. M. C. (2019a). Unravelling mechanistic reasons for differences in performance of different Ti- and Bi-based magnetic photocatalysts in photocatalytic degradation of PPCPs. Sci. Total Environ. 686, 878–887. doi:10.1016/j.scitotenv.2019.05.340
Khan, M., Fung, C. S. L., Kumar, A., and Lo, I. M. C. (2019b). Magnetically separable BiOBr/Fe3O4@SiO2 for visible-light-driven photocatalytic degradation of ibuprofen: mechanistic investigation and prototype development. J. Hazard. Mater. 365, 733–743. doi:10.1016/j.jhazmat.2018.11.053
Kheshti, Z., Hassanajili, S., and Ghajar, K. A. (2019). Study and optimization of a high-gradient magnetic separator using flat and lattice plates. IEEE Trans. Magn. 55, 1–8. doi:10.1109/tmag.2018.2883624
Khosravani Goshtasb, Z., Mehdi Sabzehmeidani, M., Ghaedi, M., Madadi Avargani, V., and Moradi, Z. (2022). Magnetic Ag3PO4/Ag2CrO4/Fe/Fe3O4 quaternary composite for improved solar-driven photocatalytic degradation of cationic dyes under natural solar radiation. J. Photochem. Photobiol. A Chem. 428, 113856. doi:10.1016/j.jphotochem.2022.113856
Kisała, J., Vasile, B. S., Ficai, A., Ficai, D., Wojnarowska-Nowak, R., and Szreder, T. (2023). Reductive photodegradation of 4,4′-Isopropylidenebis(2,6-dibromophenol) on Fe3O4 surface. Materials 16, 4380. doi:10.3390/ma16124380
Klu, P. K., Nasir Khan, M. A., Wang, C., Qi, J., Sun, X., and Li, J. (2022). Mechanism of peroxymonosulfate activation and the utilization efficiency using hollow (Co, Mn)3O4 nanoreactor as an efficient catalyst for degradation of organic pollutants. Environ. Res. 207, 112148. doi:10.1016/j.envres.2021.112148
Kohantorabi, M., Moussavi, G., Oulego, P., and Giannakis, S. (2021). Radical-based degradation of sulfamethoxazole via UVA/PMS-assisted photocatalysis, driven by magnetically separable Fe3O4@CeO2@BiOI nanospheres. Sep. Purif. Technol. 267, 118665. doi:10.1016/j.seppur.2021.118665
Kumar, A., Khan, M., Fang, L., and Lo, I. M. C. (2019). Visible-light-driven N-TiO 2 @SiO 2 @Fe 3 O 4 magnetic nanophotocatalysts: synthesis, characterization, and photocatalytic degradation of PPCPs. J. Hazard. Mater. 370, 108–116. doi:10.1016/j.jhazmat.2017.07.048
Kumar, U., Kuntail, J., Kumar, A., Prakash, R., Pai, M. R., and Sinha, I. (2022). In-situ H2O2 production for tetracycline degradation on Ag/s-(Co3O4/NiFe2O4) visible light magnetically recyclable photocatalyst. Appl. Surf. Sci. 589, 153013. doi:10.1016/j.apsusc.2022.153013
Kunarti, E. S., Roto, R., Sutarno, S., Budi, I. S., and Masdiansyah, M. (2021). Effective photocatalytic degradation of nitrobenzene by magnetite modified titania composite. Asian J. Chem. 33, 1319–1324. doi:10.14233/ajchem.2021.23137
Lendzion-Bielun, Z., Wojciechowska, A., Grzechulska-Damszel, J., Narkiewicz, U., Sniadecki, Z., and Idzikowski, B. (2020). Effective processes of phenol degradation on Fe3O4–TiO2 nanostructured magnetic photocatalyst. J. Phys. Chem. Solids 136, 109178. doi:10.1016/j.jpcs.2019.109178
Lenzi, G. G., Lopes, M. F., Andrade, D. I., Napoli, J. S., Parolin, A., Fávaro, Y. B., et al. (2021). Functioned catalysts with magnetic core applied in ibuprofen degradation. Water Sci. Technol. 84, 2158–2179. doi:10.2166/wst.2021.409
Leong, S. S., Yeap, S. P., and Lim, J. K. (2016). Working principle and application of magnetic separation for biomedical diagnostic at high- and low-field gradients. Interface Focus 6, 20160048. doi:10.1098/rsfs.2016.0048
Li, S., Wang, Z., Zhao, X., Yang, X., Liang, G., and Xie, X. (2019). Insight into enhanced carbamazepine photodegradation over biochar-based magnetic photocatalyst Fe3O4/BiOBr/BC under visible LED light irradiation. Chem. Eng. J. 360, 600–611. doi:10.1016/j.cej.2018.12.002
Li, Y., Zhai, Y., Zhang, P., Wang, X., Cui, H., Li, J., et al. (2019). Synthesis of titania coated magnetic activated carbon for effective photodegradation of tannic acid in aqueous solution. Colloids Surfaces A Physicochem. Eng. Asp. 563, 141–147. doi:10.1016/j.colsurfa.2018.11.025
Li, Z., Sun, Y., Liu, D., Yi, M., Chang, F., Li, H., et al. (2022). A review of sulfate radical-based and singlet oxygen-based advanced oxidation technologies: recent advances and prospects. Catalysts 12, 1092. doi:10.3390/catal12101092
Liu, M., Zhao, M., Tan, C., Ni, Y., Fu, Q., Li, H., et al. (2024). Effect of structural optimization of magnetic MnFe2O4@Bi24O31Br10/Bi5O7I with core-shell structure on its enhanced photocatalytic activity to remove pharmaceuticals. Colloids Surfaces A Physicochem. Eng. Asp. 681, 132763. doi:10.1016/j.colsurfa.2023.132763
Liu, Z., Wang, G., Li, Y., Li, H., and Deng, N. (2024). Carboxymethyl-β-cyclodextrin functionalized TiO2@Fe3O4@RGO magnetic photocatalyst for efficient photocatalytic degradation of tetracycline under visible light irradiation. J. Environ. Chem. Eng. 12, 113303. doi:10.1016/j.jece.2024.113303
Ma, H., and Liu, C. (2021). A mini-review of ferrites-based photocatalyst on application of hydrogen production. Front. Energy 15, 621–630. doi:10.1007/s11708-021-0761-0
Machado, F., Teixeira, A. C. S. C., and Ruotolo, L. A. M. (2023). Critical review of Fenton and photo-Fenton wastewater treatment processes over the last two decades. Int. J. Environ. Sci. Technol. 20, 13995–14032. doi:10.1007/s13762-023-05015-3
Mahato, M., Mukherjee, S., and Mishra, T. (2019). Development of magnetically separable mesoporous N doped TiO2-SiO2 coated Fe3O4 nanomaterial as solar photocatalyst for environmental application. Mater. Res. Express 6, 105544. doi:10.1088/2053-1591/ab432b
Mahmoudi, K., Farzadkia, M., Rezaei Kalantary, R., Sobhi, H. R., Yeganeh, M., and Esrafili, A. (2024). Efficient removal of oxytetracycline antibiotic from aqueous media using UV/g-C3N4/Fe3O4 photocatalytic process. Heliyon 10, e30604. doi:10.1016/j.heliyon.2024.e30604
Malinowska, I., Kubica, P., Madajski, P., Ostrowski, A., Gómez Polo, C., Carvera, L., et al. (2023). Synthesis, characterization, and application of 2D/2D TiO2-GO-ZnFe2O4 obtained by the fluorine-free lyophilization method for solar light-driven photocatalytic degradation of ibuprofen. Environ. Sci. Pollut. Res. 30, 35929–35944. doi:10.1007/s11356-022-24587-0
Marghussian, V. (2015). “Magnetic properties of nano-glass ceramics,” in Nano-glass ceramics (Elsevier), 181–223. doi:10.1016/b978-0-323-35386-1.00004-9
Moniriyan, F., Sabounchei, S. J., Yousefi, A., and Akhavan, O. (2021). Synthesis, morpho-structural properties, and catalytic performances of Pt-APA@Fe3O4/GO nanocomposite based on magnetical graphene in C–C coupling reactions and photoinactivation of E. coli. J. Nanoparticle Res. 23, 192. doi:10.1007/s11051-021-05278-2
Moradi, M., Kakavandi, B., Bahadoran, A., Giannakis, S., and Dehghanifard, E. (2022). Intensification of persulfate-mediated elimination of bisphenol A by a spinel cobalt ferrite-anchored g-C3N4 S-scheme photocatalyst: catalytic synergies and mechanistic interpretation. Sep. Purif. Technol. 285, 120313. doi:10.1016/j.seppur.2021.120313
Morin-Crini, N., Lichtfouse, E., Fourmentin, M., Ribeiro, A. R. L., Noutsopoulos, C., Mapelli, F., et al. (2022). Removal of emerging contaminants from wastewater using advanced treatments. A review. Environ. Chem. Lett. 20, 1333–1375. doi:10.1007/s10311-021-01379-5
Muhammad, W., Ali, W., Khan, M. A., Ali, F., Zada, A., Ansar, M. Z., et al. (2024). Construction of visible-light-driven 2D/2D NiFe2O4/g-C3N4 Z-scheme heterojunction photocatalyst for effective degradation of organic pollutants and CO2 reduction. J. Environ. Chem. Eng. 12, 113409. doi:10.1016/j.jece.2024.113409
Niu, Z., Tao, X., Huang, H., Qin, X., Ren, C., Wang, Y., et al. (2022). Green synthesis of magnetically recyclable Mn0.6Zn0.4Fe2O4@Zn1-xMnxS composites from spent batteries for visible light photocatalytic degradation of phenol. Chemosphere 287, 132238. doi:10.1016/j.chemosphere.2021.132238
O’Dowd, K., and Pillai, S. C. (2020). Photo-Fenton disinfection at near neutral pH: process, parameter optimization and recent advances. J. Environ. Chem. Eng. 8, 104063. doi:10.1016/j.jece.2020.104063
Osanloo, M., Khorasheh, F., and Larimi, A. (2024). Fabrication of nano-dandelion magnetic TiO2/CuFe2O4 doped with silver as a highly visible-light-responsive photocatalyst for degradation of Naproxen and Rhodamine B. J. Mol. Liq. 407, 125242. doi:10.1016/j.molliq.2024.125242
Oyekunle, D. T., Gendy, E. A., Ifthikar, J., and Chen, Z. (2022). Heterogeneous activation of persulfate by metal and non-metal catalyst for the degradation of sulfamethoxazole: a review. Chem. Eng. J. 437, 135277. doi:10.1016/j.cej.2022.135277
Preeyanghaa, M., Dhileepan, M. D., Madhavan, J., and Neppolian, B. (2022). Revealing the charge transfer mechanism in magnetically recyclable ternary g-C3N4/BiOBr/Fe3O4 nanocomposite for efficient photocatalytic degradation of tetracycline antibiotics. Chemosphere 303, 135070. doi:10.1016/j.chemosphere.2022.135070
Priyadarshini, M., Ahmad, A., Yadav, S., and Ghangrekar, M. M. (2024). Activation of persulphate by a waste-extracted metal–organic framework-derived iron oxide as a photocatalyst for effective degradation of salicylic acid. J. Hazard. Toxic. Radioact. Waste 28, 04024008. doi:10.1061/jhtrbp.hzeng-1308
Qi, C., Liu, X., Ma, J., Lin, C., Li, X., and Zhang, H. (2016). Activation of peroxymonosulfate by base: implications for the degradation of organic pollutants. Chemosphere 151, 280–288. doi:10.1016/j.chemosphere.2016.02.089
Radji, G., Bettahar, N., Bahmani, A., Boukhetache, I., and Contreras, S. (2022). Heterogeneous Fenton-like degradation of organic pollutants in petroleum refinery wastewater by copper-type layered double hydroxides. J. Water Process Eng. 50, 103305. doi:10.1016/j.jwpe.2022.103305
Rangaraj, V. M., Devaraju, S., Reddy, T. G., Zafar, H., Anjum, D. H., and Mittal, V. (2024). MOF-derived Co3O4@P-doped carbon nitride/α-Fe2O3 nanocomposite as a dual Z-scheme photocatalyst for persulfate mediated Bisphenol-A degradation. Mater. Res. Bull. 179, 112925. doi:10.1016/j.materresbull.2024.112925
Saif, H. M., Gebregeorgis, T. H., Crespo, J. G., and Pawlowski, S. (2024). The influence of flow electrode channel design on flow capacitive deionization performance: experimental and CFD modelling insights. Desalination 578, 117452. doi:10.1016/j.desal.2024.117452
Sangeetha, M., Ambika, S., Madhan, D., and Vadivel, S. (2024). Photocatalytic activity of Ag doped CuFe2O4 nanoparticles supported on reduced graphene oxide for the degradation of organic dyes under visible light irradiation. J. Mater. Sci. Mater. Electron. 35, 368. doi:10.1007/s10854-024-12076-8
Sayadi, M. H., Ahmadpour, N., and Homaeigohar, S. (2021). Photocatalytic and antibacterial properties of Ag-CuFe2O4@WO3 magnetic nanocomposite. Nanomaterials 11, 298. doi:10.3390/nano11020298
Sepahvand, S., Bahrami, M., and Fallah, N. (2022). Photocatalytic degradation of 2,4-DNT in simulated wastewater by magnetic CoFe2O4/SiO2/TiO2 nanoparticles. Environ. Sci. Pollut. Res. 29, 6479–6490. doi:10.1007/s11356-021-13690-3
Shaheen, S., Xu, S., Bian, J., Zada, A., Zhang, Z. Q., Qu, Y., et al. (2024). Facile synthesis of hierarchical NiO/NiFe2O4 microsphere composite as efficient visible photocatalyst for 2,4-DCP degradation. Rare Met. 43, 1580–1588. doi:10.1007/s12598-023-02585-6
Sharma, S. K., Kumar, A., Sharma, G., Naushad, M., Ubaidullah, M., and García-Peñas, A. (2022). Developing a g-C3N4/NiFe2O4 S-scheme hetero-assembly for efficient photocatalytic degradation of cephalexin. Colloids Surfaces A Physicochem. Eng. Asp. 654, 129968. doi:10.1016/j.colsurfa.2022.129968
Singh, P., Sharma, K., Hasija, V., Sharma, V., Sharma, S., Raizada, P., et al. (2019). Systematic review on applicability of magnetic iron oxides–integrated photocatalysts for degradation of organic pollutants in water. Mater. Today Chem. 14, 100186. doi:10.1016/j.mtchem.2019.08.005
Smułek, W., Bielan, Z., Pacholak, A., Zdarta, A., Zgoła-Grześkowiak, A., Zielińska-Jurek, A., et al. (2021). Nitrofurazone removal from water enhanced by coupling photocatalysis and biodegradation. Int. J. Mol. Sci. 22, 2186. doi:10.3390/ijms22042186
Song, D., Zheng, Z., Wang, Z., Zhao, M., Ding, L., Zhang, Q., et al. (2024). Catalytic PMS oxidation universality of CuFe2O4/MnO2 heterojunctions at multiple application scenarios. Environ. Res. 243, 117828. doi:10.1016/j.envres.2023.117828
Sonu, , Dutta, V., Sharma, S., Raizada, P., Hosseini-Bandegharaei, A., Kumar Gupta, V., et al. (2019). Review on augmentation in photocatalytic activity of CoFe2O4 via heterojunction formation for photocatalysis of organic pollutants in water. J. Saudi Chem. Soc. 23, 1119–1136. doi:10.1016/j.jscs.2019.07.003
Sun, H., Wang, L., Wang, X., Dong, Y., and Pei, T. (2024). A magnetically recyclable Fe3O4/ZnIn2S4 type-II heterojunction to boost photocatalytic degradation of gemifloxacin. Appl. Surf. Sci. 656, 159674. doi:10.1016/j.apsusc.2024.159674
Surana, D., Gupta, J., Sharma, S., Kumar, S., and Ghosh, P. (2022). A review on advances in removal of endocrine disrupting compounds from aquatic matrices: future perspectives on utilization of agri-waste based adsorbents. Sci. Total Environ. 826, 154129. doi:10.1016/j.scitotenv.2022.154129
Tao, Y., Fan, G., Li, X., Cao, X., Du, B., Li, H., et al. (2024). Recyclable magnetic AgBr/BiOBr/Fe3O4 photocatalytic activation peroxymonosulfate for carbamazepine degradation: synergistic effect and mechanism. Sep. Purif. Technol. 330, 125392. doi:10.1016/j.seppur.2023.125392
Thomas, N., Dionysiou, D. D., and Pillai, S. C. (2021). Heterogeneous Fenton catalysts: a review of recent advances. J. Hazard. Mater. 404, 124082. doi:10.1016/j.jhazmat.2020.124082
Tian, N., Giannakis, S., Akbarzadeh, L., Hasanvandian, F., Dehghanifard, E., and Kakavandi, B. (2023). Improved catalytic performance of ZnO via coupling with CoFe2O4 and carbon nanotubes: a new, photocatalysis-mediated peroxymonosulfate activation system, applied towards Cefixime degradation. J. Environ. Manage. 329, 117022. doi:10.1016/j.jenvman.2022.117022
Torrejon, J., Raposo, V., Martinez, E., del Real, R. P., and Hayashi, M. (2020). Current-induced dynamics of chiral domain walls in magnetic heterostructures. 2nd Edn. Elsevier Ltd. doi:10.1016/B978-0-08-102832-2.00010-4
Tsaridou, C., and Karabelas, A. J. (2021). Drinking water standards and their implementation— a critical assessment. WaterSwitzerl. 13, 2918. doi:10.3390/w13202918
Vale, F., Sousa, C. A., Sousa, H., Santos, L., and Simões, M. (2022). Parabens as emerging contaminants: environmental persistence, current practices and treatment processes. J. Clean. Prod. 347, 131244. doi:10.1016/j.jclepro.2022.131244
Wang, H., Liu, H., Chu, Z., Sun, F., Zou, X., Wang, Q., et al. (2024a). Fe3O4 derived from the decomposition of siderite as a heterogeneous photocatalyst to degrade 2,4-dichlorophenol via activating PMS. J. Water Process Eng. 57, 104538. doi:10.1016/j.jwpe.2023.104538
Wang, R., Zhu, P., Liu, M., Xu, J., Duan, M., and Luo, D. (2021). Synthesis and characterization of magnetic ZnFe2O4/Bi0-Bi2MoO6 with Z-scheme heterojunction for antibiotics degradation under visible light. Sep. Purif. Technol. 277, 119339. doi:10.1016/j.seppur.2021.119339
Wang, Y., Zhao, L., Cai, X., Chen, Y., Xu, J., Zhang, L., et al. (2024b). Construction of site-specific magnetic Z-scheme CdS/Fe3O4@N-doped graphene aerogel microtube/N-doped TiO2 with porous structure: enhanced catalytic performance in photo-Fenton reaction. Environ. Sci. Pollut. Res. 31, 15091–15104. doi:10.1007/s11356-024-32190-8
Wu, Q., Siddique, M. S., Wang, H., Cui, L., Wang, H., Pan, M., et al. (2023). Visible-light-driven iron-based heterogeneous photo-Fenton catalysts for wastewater decontamination: a review of recent advances. Chemosphere 313, 137509. doi:10.1016/j.chemosphere.2022.137509
Wu, Q., and Song, Y. (2023). Recent advances in spinel ferrite-based magnetic photocatalysts for efficient degradation of organic pollutants. Water Sci. Technol. 87, 1465–1495. doi:10.2166/wst.2023.077
Wu, Y., Fang, Z., Shi, Y., Chen, H., Liu, Y., Wang, Y., et al. (2019). Activation of peroxymonosulfate by BiOCl@Fe3O4 catalyst for the degradation of atenolol: kinetics, parameters, products and mechanism. Chemosphere 216, 248–257. doi:10.1016/j.chemosphere.2018.10.012
Xie, L., Ren, Z., Zhu, P., Xu, J., Luo, D., and Lin, J. (2021). A novel CeO2–TiO2/PANI/NiFe2O4 magnetic photocatalyst: preparation, characterization and photodegradation of tetracycline hydrochloride under visible light. J. Solid State Chem. 300, 122208. doi:10.1016/j.jssc.2021.122208
Xu, G., Du, M., Li, T., Guan, Y., and Guo, C. (2021). Facile synthesis of magnetically retrievable Fe3O4/BiVO4/CdS heterojunction composite for enhanced photocatalytic degradation of tetracycline under visible light. Sep. Purif. Technol. 275, 119157. doi:10.1016/j.seppur.2021.119157
Xue, Q., Song, B., Feng, Q., Yu, Z., Hu, K., Yang, Y., et al. (2024). Efficiently removal of tetracycline via synergistic photocatalysis with Fenton reaction with biochar/FeOOH. Appl. Surf. Sci. 645, 158869. doi:10.1016/j.apsusc.2023.158869
Yilmaz, M., Mengelizadeh, N., Saloot, M. K., Shahbaksh, S., and Balarak, D. (2022). Facile synthesis of Fe3O4/ZnO/GO photocatalysts for decolorization of acid blue 113 under solar, visible and UV lights. Mater. Sci. Semicond. process. 144, 106593. doi:10.1016/j.mssp.2022.106593
Younis, S. A., and Kim, K. H. (2020). Heterogeneous photocatalysis scalability for environmental remediation: opportunities and challenges. Catalysts 10, 1109. doi:10.3390/catal10101109
Yu, X., Sun, J., Li, G., Huang, Y., Li, Y., Xia, D., et al. (2020). Integration of •SO4−-based AOP mediated by reusable iron particles and a sulfidogenic process to degrade and detoxify Orange II. Water Res. 174, 115622. doi:10.1016/j.watres.2020.115622
Zahedifar, M., and Seyedi, N. (2022). Bare 3D-TiO2/magnetic biochar dots (3D-TiO2/BCDs MNPs): highly efficient recyclable photocatalyst for diazinon degradation under sunlight irradiation. Phys. E Low-dimensional Syst. Nanostructures 139, 115151. doi:10.1016/j.physe.2022.115151
Zhao, M., Wang, Y., Liu, M., Chen, W., Lin, Y., Li, C., et al. (2022). Photocatalytic treatment for antibacterials wastewater with high-concentration using ZnFe2O4/Bi7O9I3 magnetic composite with optimized morphology and structure. Colloids Surfaces A Physicochem. Eng. Asp. 649, 129375. doi:10.1016/j.colsurfa.2022.129375
Zheng, Z., He, J., Zhang, Z., Kumar, A., Khan, M., Lung, C. W., et al. (2024). Magnetically recyclable nanophotocatalysts in photocatalysis-involving processes for organic pollutant removal from wastewater: current status and perspectives. Environ. Sci. Nano 11, 1784–1816. doi:10.1039/d3en00906h
Zheng, Z., Min, J., Wang, X., Lung, C. W., Shih, K., and Lo, I. M. C. (2023). Directional separation of highly reductive electrons to the reactive center in a magnetic S-scheme ZnFe2O4/A-MoS2 heterojunction for enhanced peroxymonosulfate activation toward pharmaceuticals and personal care product removal. Environ. Sci. Technol. 57, 8414–8425. doi:10.1021/acs.est.2c09122
Zhou, A., Liao, L., Wu, X., Yang, K., Li, C., Chen, W., et al. (2020). Fabrication of a Z-scheme nanocomposite photocatalyst for enhanced photocatalytic degradation of ibuprofen under visible light irradiation. Sep. Purif. Technol. 250, 117241. doi:10.1016/j.seppur.2020.117241
Zhu, M., Li, J., Chen, M., Li, Y., Zhang, Q., Tang, Y., et al. (2024). Magnetically separable Cd/CdS-ZnFe2O4/α-Fe2O3 Z-scheme heterostructure for boosting the photo-Fenton removal of antibiotic. J. Alloys Compd. 988, 174319. doi:10.1016/j.jallcom.2024.174319
Zimmermann, G., Pridoehl, M., Knipping, J., and Roth, P. (2003). Superparamagnetic nanocomposite materials. Magnetohydrodynamics 39, 71–76. doi:10.1016/b978-0-323-44923-6.00005-4
Keywords: magnetic photocatalyst, magnetic separation, photocatalysis, wastewater, organic pollutants
Citation: González-Fernández C, Bringas E, Rivero MJ and Ortiz I (2024) Revealing the role of magnetic materials in light-driven advanced oxidation processes: enhanced degradation of contaminants and facilitated magnetic recovery. Front. Chem. Eng. 6:1430773. doi: 10.3389/fceng.2024.1430773
Received: 10 May 2024; Accepted: 22 July 2024;
Published: 08 August 2024.
Edited by:
Patricia Luis, Université Catholique de Louvain, BelgiumReviewed by:
Diana Sannino, University of Salerno, ItalyCopyright © 2024 González-Fernández, Bringas, Rivero and Ortiz. This is an open-access article distributed under the terms of the Creative Commons Attribution License (CC BY). The use, distribution or reproduction in other forums is permitted, provided the original author(s) and the copyright owner(s) are credited and that the original publication in this journal is cited, in accordance with accepted academic practice. No use, distribution or reproduction is permitted which does not comply with these terms.
*Correspondence: Inmaculada Ortiz, b3J0aXppQHVuaWNhbi5lcw==
Disclaimer: All claims expressed in this article are solely those of the authors and do not necessarily represent those of their affiliated organizations, or those of the publisher, the editors and the reviewers. Any product that may be evaluated in this article or claim that may be made by its manufacturer is not guaranteed or endorsed by the publisher.
Research integrity at Frontiers
Learn more about the work of our research integrity team to safeguard the quality of each article we publish.