- 1Nanotechnology Innovation Centre (NIC), Advanced Materials Division, Mintek, Private Bag X3015, Randburg, South Africa
- 2Department of Physics, University of Free State, Bloemfontein, South Africa
The heightened occurrence of emerging organic pollutants (EOPs) in aquatic bodies has been the subject of global apprehension due to the toxicity they pose to the environment, humans and animals alike. The presence of EOPs has soared due to industrialization and is further exacerbated by human activities like the overuse and poor disposal of dyes, pesticides, pharmaceuticals, surfactants, personal care products and food additives. The complete treatment and removal of EOPs from industrial wastewater and sewage has remained a challenge because of their pseudo-persistence and resistance to degradation. Due to their impressive light absorption properties, high surface-area-to-volume ratio, high porosity, superior mechanical strength, electrospun titanium dioxide (TiO2) and zinc oxide (ZnO) nanofibers have been proposed for the photocatalytic treatment of EOPs. Therefore, this review first highlights the fabrication and modification methods of TiO2 and ZnO nanofibers. A systematic survey of the latest progress in the application of TiO2 and ZnO nanofibers for the degradation of EOPs is then elaborated. Thus, the main goal is to shed light and give insight to researchers on the possibilities surrounding the elimination of EOPs by applying electrospun TiO2 and ZnO semiconductor materials. In addition, the loopholes associated with fabrication and modification processes are discussed with the aim of encouraging innovation for prospective technology advancement and commercialization, as well as to enhance research efforts in wastewater treatment and environmental sustainability.
1 Introduction
Increased industrialization and frequent natural calamities have resulted in the shortage of water, which in turn, poses a threat to the development and livelihoods of the affected communities. The occurrence of emerging organic pollutants (EOPs) such as, industrial products/by-products, pesticides, pharmaceuticals, personal care products and food additives in water streams also contributes to the water crisis (Arman et al., 2021; Paumo et al., 2021; Singh et al., 2021). The overuse and poor disposal of EOPs results in them entering drinking water sources through runoff, artificial recharge, or effluents from wastewater treatment plants (WWTPs) (Mukhopadhyay et al., 2022). Generally, these EOPs have no environmental monitoring or emission standards and have negative ecological effects (He et al., 2022). In addition to jeopardizing environmental sustainability, EOPs can compromise human health when ingested through contaminated water (Guillotin and Delcourt, 2022). They possess endocrine disrupting properties and interfere with the hormonal system (Kasonga et al., 2021; Ng et al., 2021). Moreover, they tend to bio-accumulate in the aquatic environment and exert toxic effects on aquatic animals, which can easily be transferred to humans through the food chain (Impellitteri et al., 2023).
Methods including adsorption, membrane filtration, coagulation-flocculation, solvent extraction and photocatalysis have been explored for the treatment of EOPs (Lhomme et al., 2008; Khoo et al., 2022; Mahmood et al., 2022; Ajala et al., 2023; Tahraoui et al., 2023). With the exception of photocatalysis however, most of these treatment technologies are not efficient at low concentrations of EOPs. They are also not completely destructive and merely transfer the contaminants from one phase to another (He et al., 2022). The use of photocatalysis for the treatment of EOPs has therefore gained interest due to the possibility of achieving high/complete mineralization of the target pollutants. In addition, the process generally does not require high temperatures and pressures, and it has relatively low operating costs (Kumar et al., 2014).
In essence, photocatalysis is based on the light-driven in-situ generation of oxidant species that are highly reactive towards organic and inorganic contaminants (Ramírez-Malule et al., 2020). Due to their impressive light absorption properties and concurrent ability to promote the formation of reactive oxygen species, titanium dioxide (TiO2) and zinc oxide (ZnO) are the most studied semiconductor based photocatalysts (Li et al., 2014; Kumari et al., 2023). They also possess attractive properties including prolonged excited-state lifetimes, enhanced charge transport features, good chemical and optical stability, relatively low cost, abundance, corrosion resistance and non-toxicity (Hernández et al., 2015).
Both TiO2 and ZnO have been synthesized to afford a range of morphologies including nanoflowers, microspheres, nanoribbons, nanoparticles, nanotubes, nanocages and nanofibers (Wang and Lou, 2012; Samanta et al., 2015; Marien et al., 2017; Samadipakchin et al., 2017; Lan et al., 2018; Zhang et al., 2018; Qu et al., 2020). Recently, research interest on nanofibers has soared due to their attractive properties including their high surface-area-to-volume ratio, high porosity, superior mechanical strength, versatile surface functionalization and tunable properties (Prabhu, 2019). Generally, nanofibers can be fabricated using techniques such as sol-gel, hydrothermal, template synthesis, and electrospinning (You et al., 2012; Gupta et al., 2018; Ademola Bode-Aluko et al., 2021; Chen et al., 2021; Vakhrushev and itsova, 2021). The sol-gel and hydrothermal methods suffer from drawbacks including long processing time and high cost of equipment, respectively (Modan and Plaiasu, 2020). The template synthesis method cannot produce nanofibers with a long fiber length because the templates come with preconfigured layouts and designs (Alghoraibi and Alomari, 2018). Electrospinning however has become the most commonly employed nanofiber fabrication technique due to its simplicity, low cost of production and its ability to produce continuous fibers with diameters ranging from tens of nanometers to several micrometers. Electrospinning also allows for easy modification of the nanofibers and is easily reproducible (Ademola Bode-Aluko et al., 2021). Hence, the focus of this review is on electrospun TiO2 and ZnO nanofibers for the treatment of EOPs in wastewater.
Thus, this article is intended to give insight to researchers on the possibilities surrounding the elimination of EOPs by applying electrospun TiO2 and ZnO nanofibers. Recent advances on the fabrication and modification of semiconductor-based nanofibers are discussed and a critical analysis of their efficiencies in the photocatalytic treatment of different EOPs is conducted. Lastly, challenges and possible future prospects in their application in wastewater treatment are identified and deliberated.
2 Electrospinning inorganic TiO2 and ZnO nanofibers
Purely inorganic nanofibers (e.g., the TiO2 and ZnO nanofibers) are generally made out of nano-size grains or crystals wherein the size of the grains influences their specific surface area and thus the resultant properties. The nanofiber fabrication process using electrospinning technique is depicted in Figure 1 (Na et al., 2021a). Briefly, a solution consisting of a polymer, volatile solvent and metal precursor is prepared. Examples of some of the commonly employed metal precursors for the preparation of the TiO2 nanofibers include titanium (IV) isopropoxide, titanium tetraisopropoxide, titanium (IV) n-butoxide and tetra-n-butyl titanate (da Silva et al., 2023; Li and Xia, 2003; Secundino-Sánchez et al., 2022a; Lv et al., 2021), whereas the ZnO nanofibers are commonly synthesized from salts including zinc acetate and zinc nitrate (Mali et al., 2013; Di Mauro et al., 2016). The preparation of both the ZnO and TiO2 nanofibers using electrospinning also requires the use of a sacrificial polymer that is chosen based of its thermal degradation (Zagho and Elzatahry, 2016). Some examples of commonly used sacrificial polymers are polyvinyl pyrrolidone, polyvinyl alcohol and polyacrylonitrile amongst others (Nataraj et al., 2012; Zagho and Elzatahry, 2016). The as-prepared solution is then electrospun wherein upon exposure to high voltage (5–40 kV); nanofibers are formed on a conductive collector (Barhoum et al., 2019). In order to obtain the purely inorganic nanofibers, the obtained inorganic-organic composite nanofibers are subjected to thermal treatment, known as calcination.
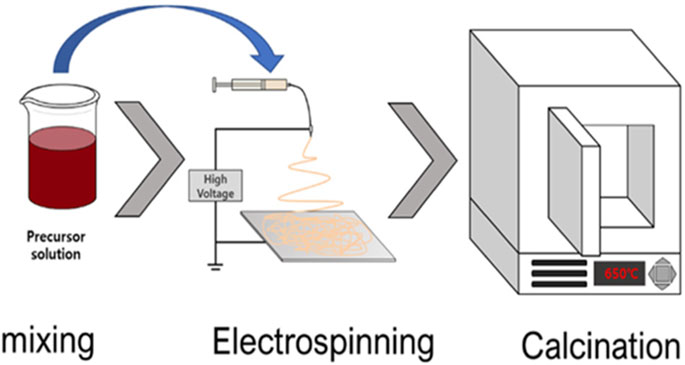
FIGURE 1. Schematic diagram of the inorganic nanofiber fabrication process with electrospinning and calcination. Modified and reprinted with permission (Na et al., 2021a).
During calcination, the temperature and heating rate influence the properties of the resultant nanofibers, including morphology, the phase and chemical composition (Nakonieczny et al., 2021; Vasiljević et al., 2021). This is due to the processes associated with calcination such as removal of residual solvents and water vapour from the nanofibers (Shendokar et al., 2008). In addition, nanofiber shrinkage often takes place as the organic polymer is removed and condensation, as well as structural relaxation proceed (Barhoum et al., 2019). In most cases, no catalytic activity is associated with the polymer, it merely acts as a carrier of the embedded photocatalysts and allows for nanofiber formation. Thus, calcination of the inorganic-organic composite nanofibers not only yields purely crystalline nanofibers, but also removes the polymer and all other organic components thereby resulting in inorganic nanofibers. The resulting inorganic nanofibers exhibit interesting optical and electronic properties as their size approaches the nanoscale. They also possess high crystallinity and flexibility due to their high surface area-to-volume ratio and nanograins (Barhoum et al., 2019; Gugulothu et al., 2019).
Depending on the calcination temperature, TiO2 nanofibers generally exist in different polymorphs, viz. anatase, mixed anatase–rutile or rutile (Nuansing et al., 2006). Both anatase and rutile exhibit tetragonal crystalline geometry, however the angles between the Ti-O bonds of the former are more distorted from 90°. These configurational differences result in a lower thermal stability and larger band gap for anatase (∼3.2 eV) relative to rutile (∼3.0 eV) (Paunović et al., 2021). The ZnO nanofibers on the other hand generally exist as a wurtzite phase (∼3.3 eV) as it is the most stable phase of ZnO relative to the zinc blende, and rock salt phases (Wróbel and Piechota, 2008; Bolarinwa et al., 2017).
3 Modification strategies
Various strategies have been employed to modify TiO2 and ZnO nanofibers to fine-tune and enhance their physical properties and promote versatility in their applications. Modifying the surfaces of the nanofibers also enhances their photocatalytic efficiency as it suppresses charge recombination. Additionally, both TiO2 and ZnO have wide band gaps of
3.1 Doping
Dopants generally work by modulating the active sites of materials, thereby providing a powerful means for creating a large variety of highly efficient catalysts for various reactions (Zhang et al., 2021). Doping with metal cations, non-metal anions or non-metal molecules can strongly enhance the light absorption efficiency of a photocatalyst by influencing its electronic structure (Zhang et al., 2010).
As shown in Figure 2, doping semiconductors (TiO2 used as an example) with non-metals like nitrogen can reduce the band gap of the former by creating an intermediate band for the electrons below the conduction band or above the valence band (Mahy et al., 2018). Moreover, nitrogen has the capability to change the surface-electronic properties TiO2. The comparable atomic size of nitrogen (155 pm) with oxygen (152 pm) allows nitrogen to substitute the oxygen atoms from the TiO2 lattice, causing enhanced photocatalytic efficiencies (Natarajan et al., 2021).
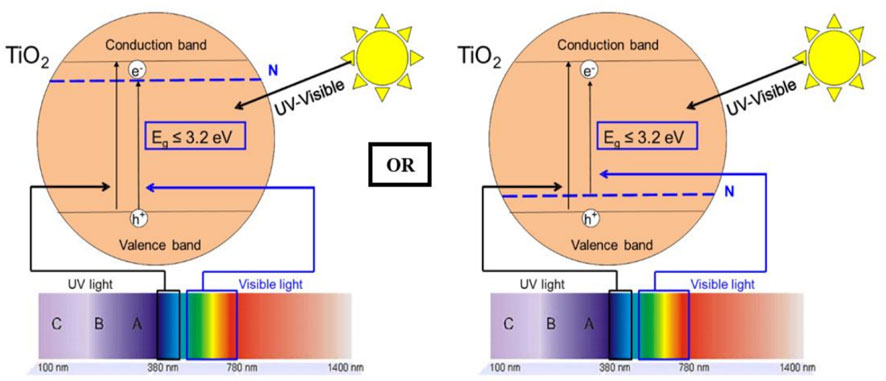
FIGURE 2. Effect of N-doping on TiO2 photocatalysis. Reprinted with permission (Mahy et al., 2018).
Doping is therefore an effective method for fabricating visible light-active photocatalysts, due to the ability of the ions from the dopant to incorporate into the catalyst crystal lattice of the semiconductors and modify its electron properties (Zhang et al., 2010). For instance, transition metals possess several valences and a d-electron structure that is not full and can accept extra electrons, thereby restricting charge recombination in the semiconductors (Estévez Ruiz et al., 2023).
Possible limitations of doping include photocorrosion and promoted charge recombination at the metal sites (Ellappan and Miranda, 2014). Doping can also disrupt the structural integrity and induce distortions on the surface of the nanofibers. Nonetheless, researchers have doped TiO2 and ZnO nanofibers with metals and non-metals, mainly for an enhanced photo-response and thus catalytic efficiency as discussed next.
3.1.1 Doping of TiO2 and ZnO nanofibers
Ma et al. fabricated S-doped TiO2 nanofibers, using thiourea and carbon disulphide, as sulphur precursors. Their results revealed that sulphur atoms were successfully incorporated into the bulk phase of the TiO2 nanofibers. The S-doping could also effectively inhibit the growth of crystalline grain size and increase light absorbance in the visible region (Ma et al., 2014). Similarly, Camillio et al. prepared N-doped TiO2 nanofibers. Doping enhanced the light absorption properties of the TiO2 nanofibers, resulting in visible-light active nanofibers (Di Camillo et al., 2012). Roongraung et al. fabricated Ag-doped TiO2 nanofibers with different Ag contents, resulting in nanofibers with even better properties than the commercial TiO2 (P25) powder (Roongraung et al., 2020).
Song et al. fabricated W-doped TiO2 nanofibers. Before doping, the TiO2 nanofibers composed of anatase and rutile phases. Interestingly, doping the TiO2 nanofibers with tungsten completely transformed their rutile phase to anatase phase (Song et al., 2016). Similarly, Na et al. prepared Fe-doped TiO2 nanofibers wherein the crystal phase of the TiO2 matrix was transitioned from anatase to rutile after doping. This is demonstrated in Figure 3 wherein the selected area electron diffraction results for the nanofibers showed a ring-type diffraction pattern, indicating crystallinity. As shown in Figure 3A, the diffraction pattern of the TiO2 nanofibers had a [101] plane, attributed to the anatase phase, while the Fe-doped TiO2 nanofibers (Figure 3B), were indexed to the rutile phase (Na et al., 2021b). It has been reported that phase transformations do occur when TiO2 nanomaterials are doped, depending on several parameters including the method of doping, dopant type and concentration used, as well as the reaction atmosphere (Ahmad et al., 2007).
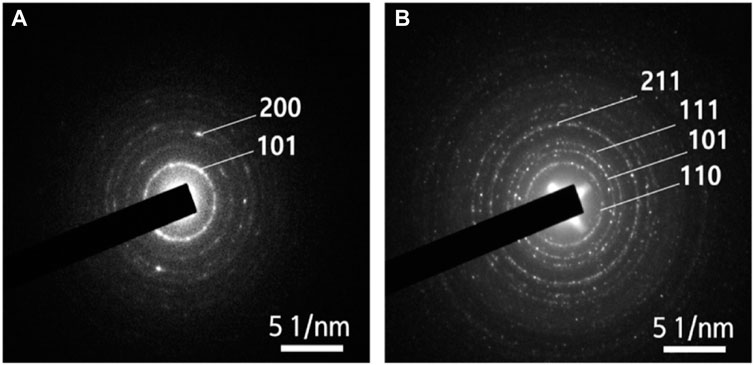
FIGURE 3. Selected area electron diffraction patterns obtained from nanofibers: (A) TiO2 nanofibers and (B) Fe-doped TiO2 nanofibers. Reprinted with permission (Na et al., 2021b).
Moreover, ZnO nanofibers have also been doped to alter and enhance their properties. Wang et al. fabricated Mn2+-doped and N-decorated ZnO nanofibers. The doped ZnO nanofibers exhibited enhanced photocatalytic properties due to their synergetic interaction with both Mn2+ and N (Wang et al., 2016). Sun et al. (2023) prepared Rh-doped ZnO nanofibers wherein the doping introduced large amounts of surface oxygen vacancy defects and enhanced the optical properties of the nanofibers (Sun et al., 2023).
In addition to enhancing the visible light absorption, some dopants induce magnetic properties to the nanofibers, making their magnetic regeneration post-application easy. For instance, Baranowska-Korczyc et al. fabricated Fe-doped ZnO nanofibers exhibiting ferromagnetism at room temperature. This feature was found to either be mediated by the presence of oxygen vacancies or related to the presence of small precipitates of ferromagnetic phases of iron (Baranowska-Korczyc et al., 2012). Chen et al. fabricated Cu-doped ZnO nanofibers using two methods: adding Cu salt in the sol-gel precursor before electrospinning and thermally diffusing Cu atoms into the pure ZnO nanofibers. They showed that the nanofibers obtained by adding Cu salt in the sol-gel precursor before electrospinning were paramagnetic while those obtained by thermal in-diffusion exhibited ferromagnetism at room temperature (Chen et al., 2020).
3.2 Dye photosensitization
Dye photosensitization entails modifying TiO2 and ZnO with either natural or synthetic dye extracts, thereby activating their visible light absorption capacity (Goulart et al., 2020). Most of the commonly used photosensitizing dyes reported in the literature include porphyrins and metallophthalocyanines (Duan et al., 2012; Atta-Eyison et al., 2021; Keşir et al., 2021).
As depicted in Figure 4, upon exposure to visible radiation, the excited dye passes an electron from its highest occupied molecular orbital (HOMO) to its lowest unoccupied molecular orbital (LUMO), and subsequently to the conduction band of the semiconductor (Bayrak et al., 2016; Diaz-Angulo et al., 2019). This results in an enhanced photocatalytic efficiency for the semiconductor and enables its activation using visible light. On the other hand, the dyes that are not adsorbed on the semiconductor and are in the solution can also absorb light, allowing for the electrons in the LUMO to react with dissolved oxygen in order to produce superoxide anion radicals (O2•-) (Diaz-Angulo et al., 2019).
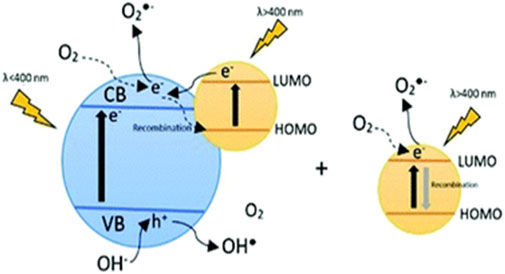
FIGURE 4. Mechanism of the dye sensitization process with the dye adsorbed onto the catalyst surface and dye in the bulk. Reprinted with permission (Diaz-Angulo et al., 2019).
In order for dyes to be considered as efficient photosensitizers, they need to have strong absorption of visible light or part of the near infrared (NIR) region (Abrahamse and Hamblin, 2016). They also need to be photostable and have anchoring groups (e.g., SO3H, –COOH, –H2PO3, etc.) to facilitate the binding of dye molecules onto the surface of the semiconductor (Diaz-Angulo et al., 2019). Moreover, the excited state of the dye photosensitizers should be higher in energy than the conduction band edge of the semiconductor to enable efficient electron transfer between the two (Yun et al., 2017).
Dye photosensitization does however have limitations including lack of long-term stability as sometimes the dye itself suffers degradation by irradiation, thereby changing its structure and properties (Belver et al., 2019). The synthesis of the dyes can also be an issue as the processes often involve organic reactions with several steps and low yields, making them expensive to generate. Nonetheless, a few researchers have reported on the dye photosensitization of TiO2 and ZnO nanofibers.
3.2.1 Dye-photosensitized TiO2 and ZnO nanofibers
Guo et al. modified TiO2 nanofibers with 2,9,16,23-tetra-nitrophthalocyanine iron(II) (Guo et al., 2012). The resulting nanofibers exhibited an enhanced photo-response relative to the unmodified ones. Mkhondwanae et al. modified TiO2 nanofibers using a Zn-5-p-carboxyphenyl-10,15,20-(tris-4-pyridyl)-porphyrin (amongst other things) and found that the nanofibers were highly active in visible light driven photocatalysis (Mkhondwane et al., 2022). Hlabangwane et al. modified TiO2 nanofibers with free base, tin (II) and indium (III) tetramethoxyporphyrins for comparison of photo-response enhancement efficiencies (Hlabangwane et al., 2023). Arifin et al. enhanced the optical properties of their ZnO nanofibers by modifying them using a ruthenium complex dye (Arifin et al., 2020). Lastly, Mapukata et al. modified both TiO2 and ZnO nanofibers with a zinc phthalocyanine, reporting that the modified nanofibers had a higher photo-response and photocatalytic efficiency than the unmodified ones (Mapukata and Nyokong, 2020).
3.3 Composite nanofibers
Some scientists have explored combining TiO2 and ZnO together or with other semiconductors to create composite nanofibers. This is because the synergistic interaction between the individual semiconductor components provides enhanced physical and chemical properties (Tan et al., 2020). The interaction between composite semiconductors is shown in Figure 5. Upon photo-excitation, the individual constituents of the composite are excited and charge transfer occurs (Siwińska-Stefańska et al., 2018). This suppresses individual charge recombination and increases the lifetimes of the charge carriers, thereby enhancing the photocatalytic performance of the composite materials (Siwińska-Stefańska et al., 2018; Bai et al., 2021).
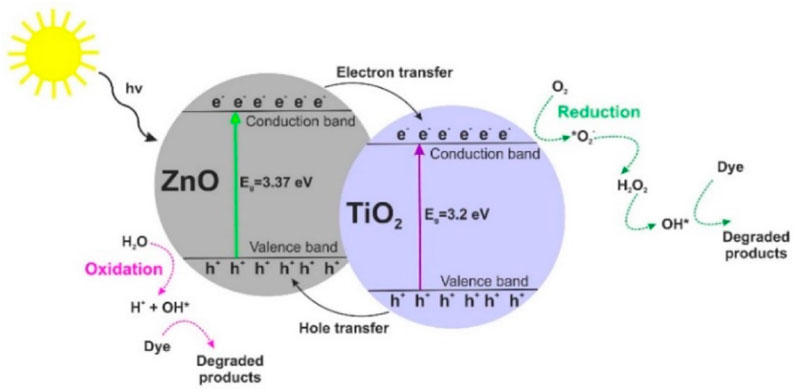
FIGURE 5. Mechanism of photo-activity of TiO2-ZnO composite materials. Reprinted with permission (Siwińska-Stefańska et al., 2018).
Composite nanofibers in particular usually have high strength, corrosion resistance, design flexibility and increased durability relative to their separate constituents (Toriello et al., 2020). However, the fabrication of composite nanofibers tends to require intricate optimisation as they can have entangled patterns with different cross-sectional structures, often with beads.
3.3.1 TiO2 and ZnO based composite nanofibers
Someswararao et al. fabricated TiO2/ZnO composite nanofibers with an improved photocatalytic activity relative to the separate TiO2 and ZnO nanofibers (Someswararao et al., 2021). Chen et al. also fabricated TiO2/ZnO composite nanofibers with varying amounts of zinc acetate (0.50%–2.00%) as shown in the scanning electron microscopy (SEM) images in Figures 6A–E. They reported that the nanofibers were smooth, even with an increase in the zinc acetate content and the overall reticular conformation was maintained. The nanofibers with 1 wt% zinc acetate did however possess more fractured surfaces existing as loose ends (Figure 6C). The elemental composition of nanofibers was confirmed as shown in Figure 6F. The fabricated nanofibers were composed of carbon, titanium, zinc and oxygen elements, demonstrating the coexistence of ZnO and TiO2 in the samples (Chen et al., 2016).
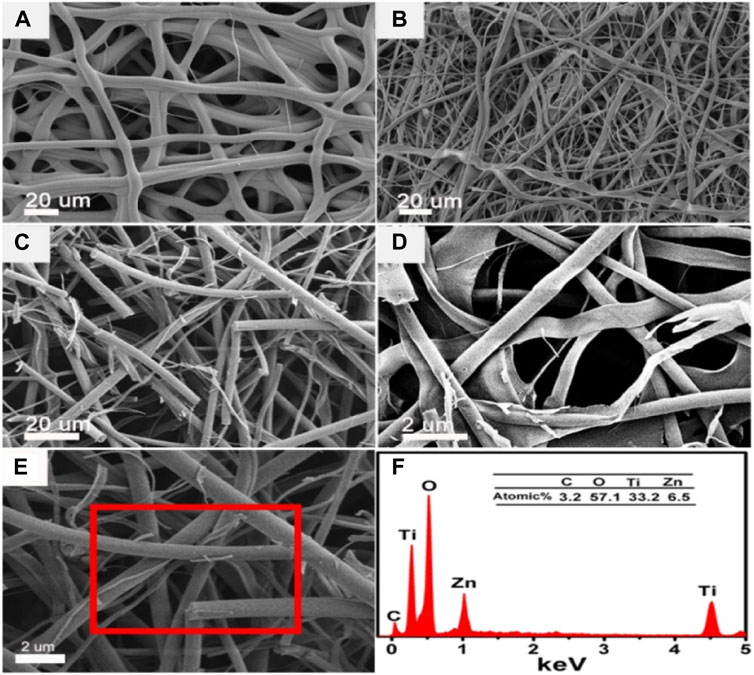
FIGURE 6. SEM pictures of the pure TiO2 and the ZnO/TiO2 nanofibers with different content of Zn(OAc)2 after calcination: (A) bare TiO2, (B) 0.5 wt%, (C) 1 wt%, (D) 2 wt%, (E) SEM image of ZnO/TiO2 (1 wt%) nanofibers, (F) EDX spectra from the selected region in image E. Reprinted with permission (Chen et al., 2016).
Moreover, numerous researchers have also reported on various composite nanofibers of TiO2 with other semiconductors like SnO2 (Shi et al., 2014; Han et al., 2020; Wang and Cheng, 2021). The fabrication of composite nanofibers with ZnO and other semiconductors has also been extensively reported (Yan et al., 2015; Zhao et al., 2015; Naderi et al., 2019). They all reported that the heterogeneous nanofibers had enhanced optical and chemical properties than the individual counterparts.
4 Treatment of emerging organic pollutants
After fabrication, modification and characterization, the nanofibers are ready for application, which in this case is photocatalytic treatment of EOPs. As shown in Figure 7, during the photocatalytic treatment process, the photocatalysts (TiO2 and ZnO nanofibers) absorb light (photon energy higher or equal to the band gap of the photocatalyst), thereby creating holes (h+) in the valence band and electrons (e−) in the conduction band (Al-Nuaim et al., 2023; Navidpour et al., 2023). The photo-generated e–/h+ pairs initiate the photocatalytic degradation process by mediating the formation of species including HO• and O2•- from atmospheric oxygen and moisture (Fotiou et al., 2014; Pavel et al., 2023). These species are well known for having the ability to oxidize and break down organic pollutants (Kondrakov et al., 2016).
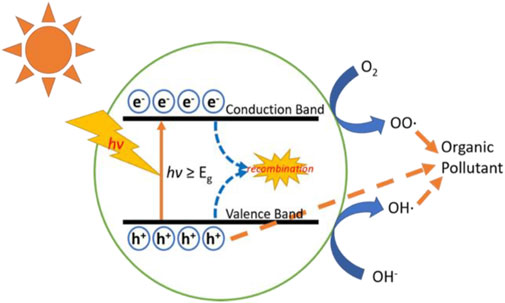
FIGURE 7. Schematic illustration of the photocatalysis process. Reprinted with permission (Gao et al., 2021).
In order to determine the internal mechanism of a photocatalytic reaction, it is necessary to identify which reactive species plays a key role in the photocatalytic process (Choudhary et al., 2021). The main oxidative species in the photocatalytic process can be detected using quenchers such as isopropyl alcohol and 5,5-dimethyl-1-pyrroline N-oxide (DMPO) are scavengers for •OH, while ammonium oxalate is a scavenger for h+. Additionally, diphenylisobenzofuran and p-benzoquinone are commonly used as scavengers for 1O2 and •O2−, respectively (Parrino et al., 2021). Techniques such as electron paramagnetic resonance spectroscopy (EPR) can be used to investigate and quantify the species formed in the presence of scavengers (Schneider et al., 2020). The radicals formed during photocatalysis have a very short lifetime (usually nanoseconds half-lives). As shown in Figure 8, the presence of diamagnetic spin trap reagents (using DMPO as an example) in the reaction medium generates more stable spin adducts that can be qualified and quantified (Al-Madanat et al., 2021). The other spin trap reagents also work in a similar manner to identify the exact reactive species/radicals doing the photocatalytic treatment.
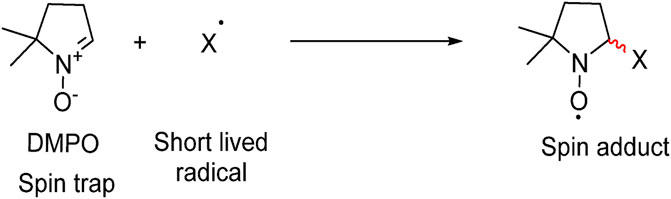
FIGURE 8. Illustration of the spin trapping method. Reprinted with permission (Al-Madanat et al., 2021).
Given its modular nature and variability of precursor chemistry, electrospinning can enable scaled-up production of photocatalytic inorganic micro- and nanofibers at reasonable cost. It therefore represents a promising fabrication method for generating highly functional nanomaterials for a range of applications (Ludwig et al., 2018). Although herein emphasis is made on their photocatalytic efficiencies for the treatment of EOPs, inorganic TiO2 and ZnO nanofibers have also been successfully applied in sensing, energy storage, air quality and antimicrobial studies (Wang et al., 2017; Thakur et al., 2020; Tshabalala et al., 2021; Zhang et al., 2023a). The fabrication of the TiO2 and ZnO nanofibers combines their elevated surface areas with their intrinsic semiconductor properties, thereby opening enormous potential for these materials. A critical evaluation of the literature published in recent years on the use of electrospun TiO2 and ZnO nanofibers for the treatment of EOPs is discussed next. The pollutants of interest are mainly dyes and pharmaceuticals, although others are briefly discussed as well.
4.1 Dyes
Dyes are colored synthetic or natural pigmented substances that chemically bond to various substrates, thereby imparting a color change (Kumar et al., 2021). They are widely utilized in various industries including, textile, tannery, as well as paper and pulp (Gomes et al., 2016; Liu, 2020). The release of dye effluents into the environment and water reservoirs has thus increased, causing drastic aesthetic alterations to water bodies (Colin et al., 2016). Their presence in water also hinders the penetration of sunlight, causing a decline in photosynthesis and water oxygen levels, thereby killing aquatic fauna and flora (Lellis et al., 2019; Al-Tohamy et al., 2022). Moreover, upon ingestion of contaminated water, dyes can pose health complications due to their carcinogenic and mutagenic properties (Singh and Chadha, 2016).
Most dyes are also highly soluble and non-biodegradable, making them more persistent in the environment and difficult to remove by conventional methods (Lellis et al., 2019). This has prompted research interests in fabricating materials and devising efficient technologies for the complete eradication of dyes as well as other EOPs.
4.1.1 Treatment of dyes using electrospun TiO2 and ZnO nanofibers
Numerous researchers have reported on the efficiency of TiO2 nanofibers for the photocatalytic treatment of dyes. For instance, Soo et al. fabricated mesoporous TiO2 nanofibers with an anatase crystalline structure obtained after calcination at 500°C. The prepared nanofibers showed good photodegradation efficiency against methylene blue (MB) and methyl orange (MO). They attributed the efficiency of their TiO2 nanofibers to a combination of high particle crystallinity as well as fiber porosity and surface area (Soo et al., 2018). On the other hand, Jafri et al. reported that having mixed polymorphs (anatase and rutile) in the TiO2 nanofibers enhances their catalytic efficiency when compared to having one phase (anatase or rutile). They pointed out that their hollow TiO2 nanofibers consisting of a mixture of 24.2% anatase and 75.8% rutile exhibited superior photocatalytic degradation of MB when compared to catalysts that consist of the anatase polymorph. They obtained their highest photocatalytic degradation efficiency of 85.50% at a catalyst loading of 0.75 g/L and dye concentration of 10 ppm. As shown in Figure 9, they ascribed the excellent performance of the mixed polymorph nanofibers to: (1) the extended surface area for UV irradiation and pollutant molecule adsorption; (2) accelerated electron-hole separation due to heterojunctions between the anatase and rutile phase; and (3) efficient light utilization due to light scattering effect in hollow nanofibers (Jafri et al., 2021).
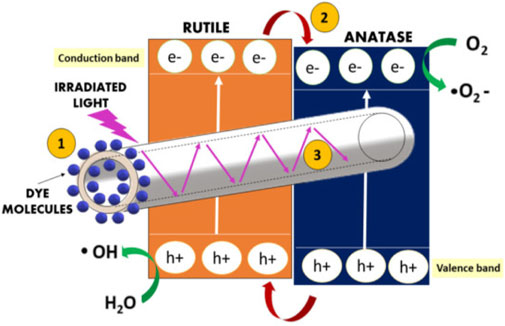
FIGURE 9. Titanium dioxide hollow nanofibers with enhanced photocatalytic properties. Reprinted with permission (Jafri et al., 2021).
In addition to the polymorphs being crucial for the photocatalytic behavior of the resulting inorganic nanofibers, modification frequently further enhances the photocatalytic performance of the final products. In their study, Formo et al. evaluated the effect of modification of anatase TiO2 nanofibers with other nanomaterials on their catalytic performance. They fabricated and calcined the nanofibers in air at 510°C, followed by functionalization with Pt nanoparticles and nanowires. They found that the nanofibers showed excellent catalytic activity for the hydrogenation of azo bonds in methyl red (MR), even more so when modified with Pt nanoparticles (Formo et al., 2008). More examples of studies that have been conducted on the use of TiO2 nanofibers for the photocatalytic degradation of dyes are listed in Table 1.
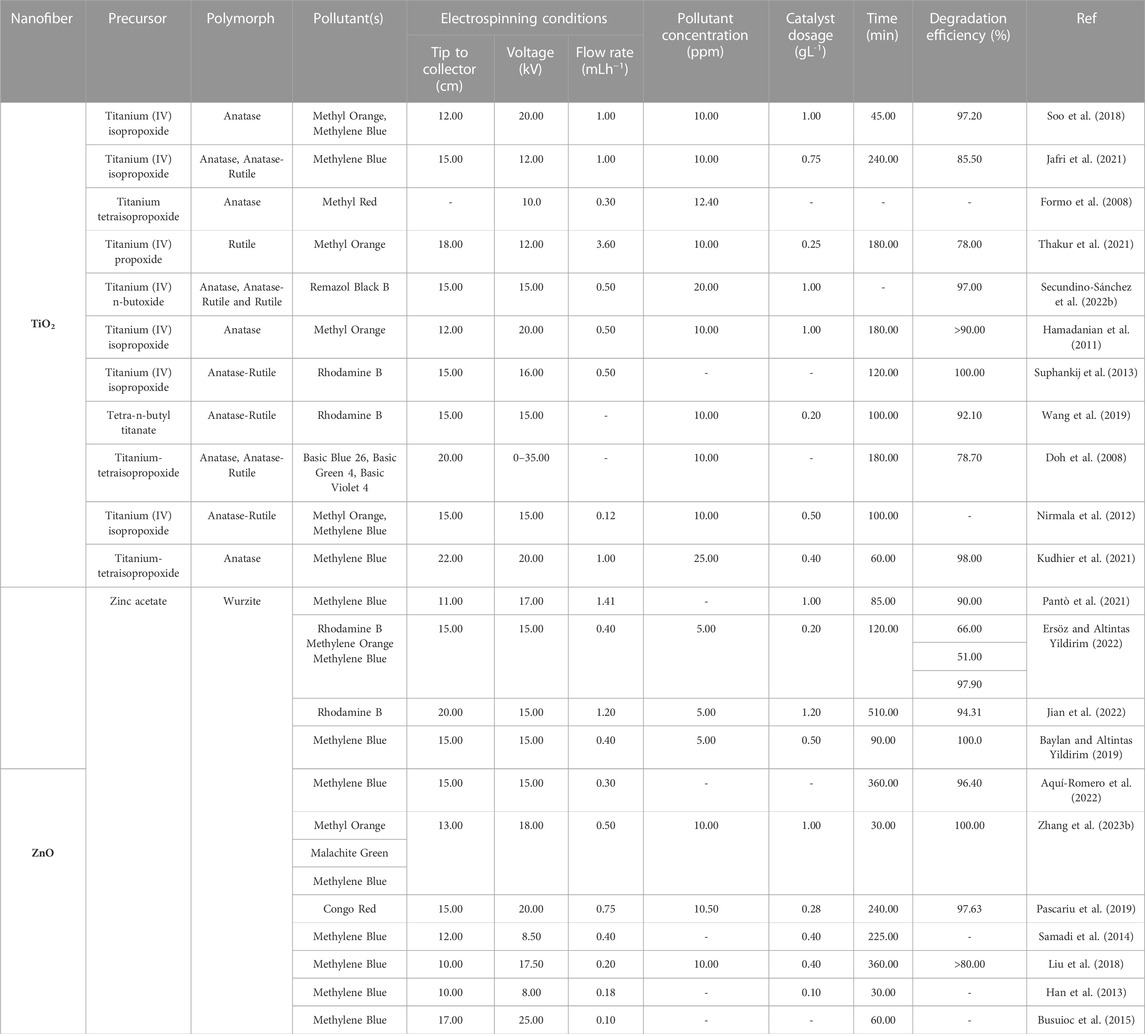
TABLE 1. Recent literature on electrospinning parameters and photocatalytic performances of TiO2 and ZnO nanofibers against dyes.
The use of ZnO-based nanofibers for the photocatalytic treatment of dyes has also been conducted and reported. In their research work, Pantò et al. fabricated ZnO nanofibers consisting of interconnected polycrystalline nanoplatelets with defect-rich hollow nanostructures. The as-prepared ZnO nanofibers were evaluated for the degradation of MB under 350 nm UV and outperformed most state-of-art electrospun pure ZnO photocatalysts (Pantò et al., 2021).
Doping ZnO nanofibers was also reported to further improve their overall photocatalytic performance. Ersöz et al. doped ZnO nanofibers with Ag to evaluate the catalytic effect of the resultant Ag-doped ZnO nanofibers. They used rhodamine B (RhB), MO and MB as model dyes and found that the Ag-doped ZnO nanofibers showed enhanced degradation efficiency relative to the unmodified ZnO nanofibers (Ersöz and Altintas Yildirim, 2022).
Similarly, Jian et al. prepared a series of La-doped ZnO nanofibers. The La-doped ZnO nanofibers were found to have a higher photocatalytic efficiency in the degradation of RhB when illuminated with visible light relative to the undoped ones (Jian et al., 2022). Baylan and Yildirim fabricated Mn-doped ZnO nanofibers wherein substitutional incorporation of Mn2+ and Mn4+ ions in ZnO resulted in the generation of additional energy levels within the band gap of ZnO. The substitutional incorporation of the dopant ions also resulted in slight morphological variations and enhanced the photocatalytic efficiency of the ZnO nanofibers in MB degradation. This was attributed to the formation of a greater number of charge carriers and the corresponding delay in the recombination process relative to the bare ZnO nanofibers (Baylan and Altintas Yildirim, 2019). More examples of studies that have been conducted on the use of ZnO nanofibers for the photocatalytic degradation of dyes are reviewed in Table 1.
Furthermore, comparison of the dye degradation efficiencies of the TiO2 and ZnO nanofibers prepared in similar conditions have been conducted. Mapukata and Nyokong reported that under the same experimental conditions, anatase TiO2 nanofibers showed better photodegradation of MO than the wurzite ZnO nanofibers (Mapukata and Nyokong, 2020). Arshad et al. conducted a similar study wherein they fabricated TiO2 and ZnO nanofibers followed by calcination at 450°C. They reported that under the same fabrication and processing conditions, the TiO2 nanofibers had better photoactivity and thus a higher MO degradation efficiency. They attributed this to the TiO2 nanofibers being more aligned and electron-supportive for conduction as compared to ZnO nanofibers, which were dense and agglomerated at some point. Moreover, their Hall Effect measurements showed that the TiO2 nanofibers had a higher conductivity of 1.38 × 10−04 Ω.cm−1, compared to ZnO nanofibers with 1.08 × 10−04 Ω.cm−1 (Arshad et al., 2023).
Some researchers also studied the photodegradation of dyes using composites of the two nanofibers, i.e., TiO2/ZnO nanofibers. For instance, Pei and Leung evaluated the photocatalytic activities of TiO2/ZnO nanofibers in the degradation of RhB under the 420 nm visible-light irradiation. The nanofibers’ diameters ranging between 80 and 130 nm were obtained by varying the concentrations of zinc acetate in the precursor solutions between 0.15% and 0.6% (Pei and Leung, 2013). 2g L−1 of TiO2/ZnO was found to be the optimal loading for efficient removal of RhB. Similarly, Leet et al. fabricated TiO2/ZnO nanofibers, which showed higher photocatalytic properties when compared to TiO2–based nanofibers in the degradation of MB. The higher activity of the composite nanofibers has been attributed to the transfer of electrons from ZnO to the TiO2 and transfer of holes from the TiO2 to the ZnO. This enhances the generation of HO• and O2•-, thereby increasing the degradation efficiency (Lee et al., 2019).
4.2 Pharmaceuticals
Pharmaceutical products have been found to be present in sewage treatment plants, surface water, ground water and drinking water due to reckless disposal of drugs and direct release from the manufacturing industries (Waleng and Nomngongo, 2022). Additionally, they can only be partly metabolized during therapeutic use, resulting in the excretion and release of residual fractions through human and animal waste (Frascaroli et al., 2021). This accelerates the spread of therapeutic resistance, thus causing a threat to human health and ecological systems (Serwecińska, 2020). Moreover, the occurrence of pharmaceuticals in potable water has become pervasive, presenting life-threatening issues because amongst other things, they are potential endocrine disruptors (Massima Mouele et al., 2021). Complete mineralization of these pharmaceuticals has proven to be a challenge using many conventional methods, thereby prompting research efforts in other fields (Papagiannaki et al., 2022). As a result, numerous researchers have proposed using TiO2 nanofibers as efficient materials for the treatment of various pharmaceuticals as discussed next.
4.2.1 Treatment of pharmaceuticals using TiO2 nanofibers
Javid et al. evaluated the performance of TiO2 nanofibers calcined at 560°C in the oxidation of the antibiotic tetracycline. At optimum conditions (tetracycline concentration: 50 mg/L, pH = 8.3, time = 15 min), they reported a maximum degradation efficiency of around 95% (Javid et al., 2013). Dhal et al. fabricated TiO2 nanofibers with mixed phases (anatase and rutile) which were efficiently applied in the degradation of tetracycline hydrochloride among other contaminants. The excellent photocatalytic activity of the nanofibers was attributed to the heterojunction at anatase–rutile interface and immediate charge transfer due to 1D structure, which inhibit the electron–hole recombination (Dhal et al., 2019). Moreover, Lin et al. supported TiO2 nanofibers onto boron-nitride sheets and applied them in the photocatalytic destruction of ibuprofen; a nonsteroidal anti-inflammatory drug. Relative to the pure TiO2 nanofibers, the supported ones had reduced recombination of charge carriers and higher photocatalytic efficiency under visible light (Lin et al., 2019).
Additionally, Pascariu et al. studied the effect of doping TiO2 nanofibers on their photocatalytic efficiency against pharmaceuticals. They prepared pure TiO2 and La3+-doped TiO2 nanofibers for the treatment of the antibiotic ciprofloxacin amongst others pollutants. They optimised the La3+-dopant content and calcination temperature to 0.1% La3+ and 600°C, respectively to achieve 99.5% degradation efficiency after 300 min irradiation under visible light (Pascariu et al., 2022a). In another study, Pascariu et al. doped the TiO2 nanofibers with Sm3+ and Er3+ at doping levels tuned in the range of 0.05%–1.0% for the mineralisation of ciprofloxacin (Pascariu et al., 2022b). They reported that TiO2:Sm (0.1%) calcined at 600°C showed superior catalytic activity towards ciprofloxacin, exhibiting a removal efficiency of ∼99.6%. Similarly, Liao et al. prepared hierarchical BiOI/TiO2 composite nanofibers for the degradation of tetracycline under visible light irradiation (Liao et al., 2022). They obtained a degradation efficiency of ∼99%, attributing it to the large surface area, interconnected interfaces and readily exposed active sites on the BiOI/TiO2 composite nanofibers. Moreover, a recent study by Ruiz-Ramírez et al. reported on the preparation of bare and Ag-doped SiO2/TiO2 composite nanofibers for the photocatalytic degradation of the antibiotic oxytetracycline. They found that the undoped composite nanofibers had a degradation percentage of 65%, which improved to 90% upon doping with Ag (Ruiz-Ramírez et al., 2023).
The degradation of pharmaceuticals using ZnO nanomaterials has been reported (Sabouni and Gomaa, 2019; Tanveer et al., 2019; Al-Khadhuri et al., 2023). However, to the best of our knowledge, there are no reports on the use of electrospun ZnO nanofibers for the treatment of pharmaceuticals, an area that can be explored in future research endeavors.
4.3 Treatment of other EOPs using TiO2 and ZnO nanofibers
When released into the environment, wastewater containing polycyclic aromatic hydrocarbons (PAHs) can disrupt the ecosystem and degrade the quality of water bodies (Pathak et al., 2023). Exposure to wastewater with PAH like naphthalene can cause headaches, vomiting, diarrhea, abdominal pain, fever and alter mental status (Volney et al., 2018). Mondal et al. proposed the use of TiO2 nanofibers for the photocatalytic treatment of these contaminants. They fabricated partially aligned free-standing mesoporous pure anatase TiO2 nanofiber mats for the photocatalytic degradation of naphthalene. Doping the nanofibers with carbon residue (2.54%) doubled the efficiency of the pristine TiO2 nanofibers in the photodegradation of the PAH, but the effectiveness declined at higher carbon content (6.45% and 10.91%) (Mondal et al., 2014). On the other hand, Sekar et al. also successfully degraded naphthalene using Fe-doped ZnO nanofibers (Sekar et al., 2018).
Wastewater containing phenols can also be very toxic, even at low concentrations (Fseha et al., 2023). Phenolic compounds are widely used in various industries including fertilizers, paints, explosives, rubbers, plastics, cosmetics, and antioxidants (Balasundram et al., 2006; Singh and Yadav, 2022). The ingestion of water contaminated with phenols can lead to severe health effects including ailments of the liver, kidney, gastrointestinal and central nervous system (Chand Meena et al., 2015; Panigrahy et al., 2022). Electrospun TiO2 nanofibers have been proposed as efficient materials for the photocatalytic treatment of these contaminants. Norouzi et al. prepared Ag-doped TiO2 nanofibers and applied them for the photodegadation of phenol under the visible light (Norouzi et al., 2020). They varied the phenol concentration, pH, dopant loading and catalyst dosage to get optimum degradation efficiencies. A maximum degradation of 93% was achieved when the phenol content was 5.62 ppm, the catalyst loading was 2.06 g L−1, and the pH value was 8. Rongan et al. prepared composite Bi2O3/TiO2 nanofibers with an S-scheme heterojunction, which exhibited superior photocatalytic activity for removal of phenol under visible light irradiation in comparison to pristine Bi2O3 and TiO2 nanofibers (Rongan et al., 2020). Similarly, Nada et al. fabricated composite WS2/TiO2 nanofibers, which showed a remarkable performance in phenol degradation (83%) under visible light (Nada et al., 2022).
The release of bisphenol A (BPA) into water bodies; which is a common monomer in a variety of industries, including the manufacturing of food containers, plastic bottles, and electronic equipment can have adverse human and animal health effects. Devastatingly, BPA has estrogen-like and anti-androgen effects causing damages to different tissues and organs, including reproductive system, immune system and neuroendocrine system (Michałowicz, 2014; Ma et al., 2019). To eradicate such a contaminant, Jafri et al. proposed the use of free-standing TiO2 hollow nanofibers to photocatalytically mineralise BPA (Jafri et al., 2022). The process entails the attack of electron-rich C3 in the phenyl group of the BPA by HO• from the excited photocatalyst (see Figure 10). Subsequently, the cleavage of the phenyl groups, forming 4-isopropanolphenol and phenol occurs. Hydroquinone is produced from the oxidation of 4-isopropanolphenol. These compounds are further degraded into short-chain aliphatic compounds through ring-opening reactions by the •OH radicals, eventually forming carbon dioxide and water.
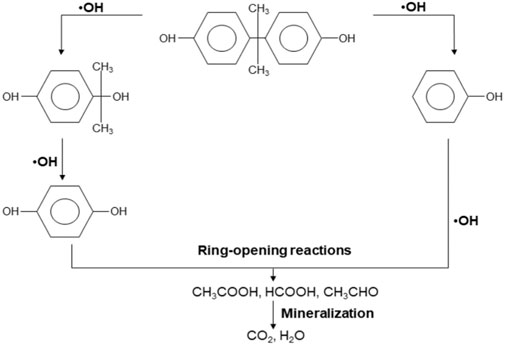
FIGURE 10. Proposed photocatalytic degradation pathway of BPA. Reprinted with permission (Jafri et al., 2022).
Another industry that generates waste with high organic content is the dairy industry. Dairy effluent is rich in organic substances including lactose, whey proteins, minerals, and lipids (Das et al., 2016; Usmani et al., 2022). The complexity of this waste has made it challenging for researchers to treat it efficiently. Electrospun ZnO nanofibers have been proposed as suitable candidates for the photocatalytic treatment of dairy effluent. Kanjwal and coworkers fabricated ZnO nanofibers and composite ZnO/NiO nanofibers amongst other materials and applied them in a range of pollutants including dairy effluents (Kanjwal et al., 2015). The ZnO nanofibers showed a maximum degradation of 75% for the dairy effluents, while the composite ZnO/NiO nanofibers eliminated 80% within 3 h.
Lastly, aside from photocatalytic decomposition of wastewater contaminants, TiO2 nanofibers have also been applied for oil-water separation. This is because oil pollution is an ever present threat in the marine environment with a large numbers of spills, being recorded every year (Asif et al., 2022). Zhang et al. electrospun pine-branch-like TiO2 nanofibrous membranes for oil-water separations. The resultant hierarchical TiO2 membrane displayed superhydrophilicity and underwater superoleophobicity. The hierarchical TiO2 membrane could separate various highly corrosive acidic, basic, and salty oil-in-water emulsions with high separation efficiencies (>99%) and stable flux (Zhang et al., 2017).
5 Conclusion and outlook
In summary, TiO2 and ZnO nanostructures have been used for the elimination of EOPs in wastewater. Their photocatalytic properties coupled with other properties such as good chemical and optical stability, relatively low cost, abundance, corrosion resistance and non-toxicity have publicized their extensive usage in water treatment applications. Their effective use relies on their morphological properties and in this instance, the fiber morphology that offers several advantageous characteristics including high surface-area-to-volume ratio, high porosity, superior mechanical strength, versatile surface functionalization and tuneable properties. Synthesized through electrospinning technique, these characteristics can further be adjusted by varying the techniques parameters to offer fibers with a range of sizes and porous structures. The electrospun nanofibers are also favorable for their easy regeneration and reusability. Modifications to enhance the photocatalytic performance of the TiO2 and ZnO nanofibers have been achieved using different strategies, such as doping, dye photosensitization and through the creation of heterogeneous nanofibers. Extensive research studies on the application of TiO2 and ZnO nanofibers as well as their modified versions have demonstrated the effectiveness of using these materials for the photocatalytic decomposition of wastewater contaminants, such as pesticides, pharmaceuticals, dyes and other pollutants. The need to protect the aquatic environment from these contaminants have seen the growth of research in development of water treatment technologies. Not only are the contaminants posing a threat to human health, but to the aquatic environment and life as well. The use of TiO2 and ZnO nanofibers and their modified versions in water treatment is promising, owing to the advances in research and innovation towards improving their efficiency, thereby ensuring access to safe and clean water. The reported literature shows that the TiO2 nanofibers have a higher photocatalytic activity relative to the commonly used commercial Degussa P-25. Moreover, the properties of the TiO2 nanofibers are highly dependent on the calcination temperatures due to the different polymorphs, wherein the purely anatase nanofibers are predominantly favored. The ZnO nanofibers still need to be explored for photodegradation of emerging pollutants, such as pharmaceuticals. It is however worth mentioning that the fabrication and modification of TiO2 and ZnO nanofibers results in photocatalysts with exceptional properties and potential in wastewater treatment applications.
Author contributions
SM: Conceptualization, Formal Analysis, Writing–original draft, Writing–review and editing. KS: Writing–review and editing. TM: Writing–review and editing.
Funding
The author(s) declare that no financial support was received for the research, authorship, and/or publication of this article.
Acknowledgments
The authors would like to thank Department of Science and Innovation (DSI)/Mintek Nanotechnology Innovation Centre, Department of Science and Innovation-National Research Foundation (DSI-NRF) and National Research Foundation (NRF), South Africa for financial support.
Conflict of interest
The authors declare that the research was conducted in the absence of any commercial or financial relationships that could be construed as a potential conflict of interest.
Publisher’s note
All claims expressed in this article are solely those of the authors and do not necessarily represent those of their affiliated organizations, or those of the publisher, the editors and the reviewers. Any product that may be evaluated in this article, or claim that may be made by its manufacturer, is not guaranteed or endorsed by the publisher.
References
Abrahamse, H., and Hamblin, M. R. (2016). New photosensitizers for photodynamic therapy. Biochem. J. 473, 347–364. doi:10.1042/bj20150942
Ademola Bode-Aluko, C., Pereao, O., Kyaw, H. H., Al-Naamani, L., Al-Abri, M. Z., Tay Zar Myint, M., et al. (2021). Photocatalytic and antifouling properties of electrospun TiO2 polyacrylonitrile composite nanofibers under visible light. Mat. Sci. Eng. B 264, 114913. doi:10.1016/j.mseb.2020.114913
Ahmad, A., Thiel, J., and Shah, S. I. (2007). Structural effects of niobium and silver doping on titanium dioxide nanoparticles. J. Phys. Conf. Ser. 61, 11–15. doi:10.1088/1742-6596/61/1/003
Ajala, O. A., Akinnawo, S. O., Bamisaye, A., Adedipe, D. T., Adesina, M. O., Okon-Akan, O. A., et al. (2023). Adsorptive removal of antibiotic pollutants from wastewater using biomass/biochar-based adsorbents. RSC Adv. 13, 4678–4712. doi:10.1039/d2ra06436g
Alghoraibi, I., and Alomari, S. (2018). “Different methods for nanofiber design and fabrication,” in Handbook of nanofibers (Cham: Springer International Publishing), 1–46.
Al-Khadhuri, A., Al-Sabahi, J., Kyaw, H. H., Myint, M. T. Z., Al-Farsi, B., and Al-Abri, M. (2023). Photocatalytic degradation toward pharmaceutical pollutants using supported zinc oxide nanorods catalyzed visible light system. Int. J. Environ. Sci. Technol. 20, 10021–10030. doi:10.1007/s13762-022-04705-8
Al-Madanat, O., Nunes, B. N., AlSalka, Y., Hakki, A., Curti, M., Patrocinio, A. O. T., et al. (2021). Application of EPR spectroscopy in TiO2 and Nb2O5 photocatalysis. Catalysts 11 (12), 1514. doi:10.3390/catal11121514
Al-Nuaim, M. A., Alwasiti, A. A., and Shnain, Z. Y. (2023). The photocatalytic process in the treatment of polluted water, 77. Springer Science and Business Media Deutschland GmbH, 677–701. Chemical Papers.
Al-Tohamy, R., Ali, S. S., Li, F., Okasha, K. M., Mahmoud, Y. A. G., Elsamahy, T., et al. (2022). A critical review on the treatment of dye-containing wastewater: ecotoxicological and health concerns of textile dyes and possible remediation approaches for environmental safety. Ecotoxicol. Environ. Saf. 231, 113160. doi:10.1016/j.ecoenv.2021.113160
Aquí-Romero, F., Santos-Sauceda, I., and Ramírez-Bon, R. (2022). Electrospun ZnO nanofibers thin films for the methylene blue degradation driven by natural sunlight. Inorg. Chem. Commun. 145, 109962. doi:10.1016/j.inoche.2022.109962
Arifin, Z., Hadi, S., Jati, H. N., and Prasetyo, S. D.Suyitno (2020). “Effect of electrospinning distance to fabricate ZnO nanofiber as photoanode of dye-sensitized solar cells,” in AIP conference proceedings (American Institute of Physics Inc.).
Arman, N. Z., Salmiati, S., Aris, A., Salim, M. R., Nazifa, T. H., Muhamad, M. S., et al. (2021). A review on emerging pollutants in the water environment: existences, health effects and treatment processes. Water 13, 3258. doi:10.3390/w13223258
Arshad, Z., Ali, M., Lee, E. J., Alshareef, M., Alsowayigh, M. M., Shahid, K., et al. (2023). Comparison of electrospun titania and zinc oxide nanofibers for perovskite solar cells and photocatalytic degradation of methyl orange dye. Catalysts 13, 1062. doi:10.3390/catal13071062
Asif, Z., Chen, Z., An, C., and Dong, J. (2022). Environmental impacts and challenges associated with oil spills on shorelines. J. Mar. Sci. Eng. 10, 762. doi:10.3390/jmse10060762
Atta-Eyison, A. A., Anukwah, G. D., and Zugle, R. (2021). Photocatalysis using zinc oxide-zinc phthalocyanine composite for effective mineralization of organic pollutants. Catal. Commun. 160, 106357. doi:10.1016/j.catcom.2021.106357
Bai, N., Liu, X., Li, Z., Ke, X., Zhang, K., and Wu, Q. (2021). High-efficiency TiO2/ZnO nanocomposites photocatalysts by sol–gel and hydrothermal methods. J. Solgel Sci. Technol. 99, 92–100. doi:10.1007/s10971-021-05552-8
Balasundram, N., Sundram, K., and Samman, S. (2006). Phenolic compounds in plants and agri-industrial by-products: antioxidant activity, occurrence, and potential uses. Food Chem. 99, 191–203. doi:10.1016/j.foodchem.2005.07.042
Baranowska-Korczyc, A., Reszka, A., Sobczak, K., Sikora, B., Dziawa, P., Aleszkiewicz, M., et al. (2012). Magnetic Fe doped ZnO nanofibers obtained by electrospinning. J. Solgel Sci. Technol. 61, 494–500. doi:10.1007/s10971-011-2650-1
Barhoum, A., Rasouli, R., Yousefzadeh, M., Rahier, H., and Bechelany, M. (2019). “Nanofiber technologies: history and development,” in Handbook of nanofibers (Springer International Publishing), 3–43.
Baylan, E., and Altintas Yildirim, O. (2019). Highly efficient photocatalytic activity of stable manganese-doped zinc oxide (Mn:ZnO) nanofibers via electrospinning method. Mater Sci. Semicond. Process 103, 104621. doi:10.1016/j.mssp.2019.104621
Bayrak, R., Albay, C., Koç, M., Altın, İ., Değirmencioğlu, İ., and Sökmen, M. (2016). Preparation of phthalocyanine/TiO2 nanocomposites for photocatalytic removal of toxic Cr(VI) ions. Process Saf. Environ. Prot. 102, 294–302. doi:10.1016/j.psep.2016.03.023
Belver, C., Bedia, J., Rodriguez, J. J., Gómez-Avilés, A., and Peñas-Garzón, M. (2019). “Semiconductor photocatalysis for water purification,” in Nanoscale materials in water purification (Elsevier), 581–651.
Bolarinwa, H. S., Onuu, M. U., Fasasi, A. Y., Alayande, S. O., Animasahun, L. O., Abdulsalami, I. O., et al. (2017). Determination of optical parameters of zinc oxide nanofibre deposited by electrospinning technique. J. Taibah Univ. Sci. 11, 1245–1258. doi:10.1016/j.jtusci.2017.01.004
Busuioc, C., Evanghelidis, A., Enculescu, M., and Enculescu, I. (2015). Optical and photocatalytic properties of electrospun ZnO fibers. Dig. J. Nanomater. Biostructures 10, 957–965.
Chand Meena, M., Band, R., and Sharma, G. (2015). Phenol and its toxicity: a case report. Iran. J. Toxicol. 8, 1222–1224.
Chen, J. D., Liao, W. S., Jiang, Y., Yu, D. N., Zou, M. L., Zhu, H., et al. (2016). Facile fabrication of ZnO/TiO2 heterogeneous nanofibres and their photocatalytic behaviour and mechanism towards rhodamine B. Nanomater Nanotechnol. 6, 9. doi:10.5772/62291
Chen, M., Liu, P., He, J. H., Wang, H. L., Zhang, H., Wang, X., et al. (2021). Nanofiber template-induced preparation of ZnO nanocrystal and its application in photocatalysis. Sci. Rep. 11, 21196. doi:10.1038/s41598-021-00303-9
Chen, Y., Xu, X., Li, X., and Zhang, G. (2020). Vacancy induced room temperature ferromagnetism in Cu-doped ZnO nanofibers. Appl. Surf. Sci. 506, 144905. doi:10.1016/j.apsusc.2019.144905
Choudhary, S., Bisht, A., and Mohapatra, S. (2021). Microwave-assisted synthesis of α-Fe2O3/ZnFe2O4/ZnO ternary hybrid nanostructures for photocatalytic applications. Ceram. Int. 47, 3833–3841. doi:10.1016/j.ceramint.2020.09.243
Colin, N., Maceda-Veiga, A., Flor-Arnau, N., Mora, J., Fortuño, P., Vieira, C., et al. (2016). Ecological impact and recovery of a Mediterranean river after receiving the effluent from a textile dyeing industry. Ecotoxicol. Environ. Saf. 132, 295–303. doi:10.1016/j.ecoenv.2016.06.017
Das, B., Sarkar, S., Sarkar, A., Bhattacharjee, S., and Bhattacharjee, C. (2016). Recovery of whey proteins and lactose from dairy waste: a step towards green waste management. PSEP 101, 27–33. doi:10.1016/j.psep.2015.05.006
da Silva, D. R. C., Mapukata, S., Currie, S., Kitos, A. A., Lanterna, A. E., Nyokong, T., et al. (2023). Fibrous TiO2 alternatives for semiconductor-based catalysts for photocatalytic water remediation involving organic contaminants. ACS Omega 8, 21585–21593. doi:10.1021/acsomega.3c00781
Dhal, J. P., Sahoo, S. K., Padhiari, S., Dash, T., and Hota, G. (2019). In-situ synthesis of mixed phase electrospun TiO2 nanofibers: a novel visible light photocatalyst. SN Appl. Sci. 1, 243. doi:10.1007/s42452-019-0261-6
Diaz-Angulo, J., Gomez-Bonilla, I., Jimenez-Tohapanta, C., Mueses, M., Pinzon, M., and Machuca-Martinez, F. (2019). Visible-light activation of TiO2 by dye-sensitization for degradation of pharmaceutical compounds. Photochem Photobiol. Sci. 18, 897–904. doi:10.1039/c8pp00270c
Di Camillo, D., Ruggieri, F., Santucci, S., and Lozzi, L. (2012). N-doped TiO2 nanofibers deposited by electrospinning. J. Phys. Chem. C 116, 18427–18431. doi:10.1021/jp302499n
Di Mauro, A., Zimbone, M., Fragalà, M. E., and Impellizzeri, G. (2016). Synthesis of ZnO nanofibers by the electrospinning process. Mater Sci. Semicond. Process 42, 98–101. doi:10.1016/j.mssp.2015.08.003
Doh, S. J., Kim, C., Lee, S. G., Lee, S. J., and Kim, H. (2008). Development of photocatalytic TiO2 nanofibers by electrospinning and its application to degradation of dye pollutants. J. Hazard Mater 154, 118–127. doi:10.1016/j.jhazmat.2007.09.118
Duan, M. Y., Li, J., Li, M., Zhang, Z. Q., and Wang, C. (2012). Pt(II) porphyrin modified TiO2 composites as photocatalysts for efficient 4-NP degradation. Appl. Surf. Sci. 258, 5499–5504. doi:10.1016/j.apsusc.2012.02.069
Ellappan, P., and Miranda, L. R. (2014). Synthesis and characterization of cerium doped titanium catalyst for the degradation of nitrobenzene using visible light. Intern. J. Photoenergy 2014, 1–9. doi:10.1155/2014/756408
Ersöz, E., and Altintas Yildirim, O. (2022). Green synthesis and characterization of Ag-doped ZnO nanofibers for photodegradation of MB, RhB and MO dye molecules. J. Korean Ceram. Soc. 59, 655–670. doi:10.1007/s43207-022-00202-3
Estévez Ruiz, E. P., Lago, J. L., and Thirumuruganandham, S. P. (2023). Experimental studies on TiO2 NT with metal dopants through Co-precipitation, sol–gel, hydrothermal scheme and corresponding computational molecular evaluations. Materials 16, 3076. doi:10.3390/ma16083076
Etacheri, V., Di Valentin, C., Schneider, J., Bahnemann, D., and Pillai, S. C. (2015). Visible-light activation of TiO2 photocatalysts: advances in theory and experiments. J. Photochem Photobiol. C. Photochem Rev. 25, 1–29. doi:10.1016/j.jphotochemrev.2015.08.003
Formo, E., Lee, E., Campbell, D., and Xia, Y. (2008). Functionalization of electrospun TiO2 nanofibers with Pt nanoparticles and nanowires for catalytic applications. Nano Lett. 8, 668–672. doi:10.1021/nl073163v
Fotiou, T., Triantis, T. M., Kaloudis, T., Papaconstantinou, E., and Hiskia, A. (2014). Photocatalytic degradation of water taste and odour compounds in the presence of polyoxometalates and TiO2: intermediates and degradation pathways. J. Photochem Photobiol. A Chem. 286, 1–9. doi:10.1016/j.jphotochem.2014.04.013
Frascaroli, G., Reid, D., Hunter, C., Roberts, J., Helwig, K., Spencer, J., et al. (2021). Pharmaceuticals in wastewater treatment plants: a systematic review on the substances of greatest concern responsible for the development of antimicrobial resistance. Appl. Sci. 11, 6670. doi:10.3390/app11156670
Fseha, Y. H., Shaheen, J., and Sizirici, B. (2023). Phenol contaminated municipal wastewater treatment using date palm frond biochar: optimization using response surface methodology. Emerg. Contam. 9, 100202. doi:10.1016/j.emcon.2022.100202
Gao, Z., Pan, C., Choi, C. H., and Chang, C. H. (2021). Continuous-flow photocatalytic microfluidic-reactor for the treatment of aqueous contaminants, simplicity, and complexity: a mini-review. Symmetry 13, 1325. doi:10.3390/sym13081325
Gomes, C. S., Piccin, J. S., and Gutterres, M. (2016). Optimizing adsorption parameters in tannery-dye-containing effluent treatment with leather shaving waste. PSEP 99, 98–106. doi:10.1016/j.psep.2015.10.013
Goulart, S., Jaramillo Nieves, L. J., Dal Bó, A. G., and Bernardin, A. M. (2020). Sensitization of TiO2 nanoparticles with natural dyes extracts for photocatalytic activity under visible light. Dyes Pigm 182, 108654. doi:10.1016/j.dyepig.2020.108654
Gugulothu, D., Barhoum, A., Nerella, R., Ajmer, R., and Bechelany, M. (2019). “Fabrication of nanofibers: electrospinning and non-electrospinning techniques,” in Handbook of nanofibers (Springer International Publishing), 45–77.
Guillotin, S., and Delcourt, N. (2022). Studying the impact of persistent organic pollutants exposure on human health by proteomic analysis: a systematic review. Int. J. Mol. Sci. 23, 14271. doi:10.3390/ijms232214271
Guo, Z., Chen, B., Mu, J., Zhang, M., Zhang, P., Zhang, Z., et al. (2012). Iron phthalocyanine/TiO2 nanofiber heterostructures with enhanced visible photocatalytic activity assisted with H2O2. J. Hazard Mater 219–220, 156–163. doi:10.1016/j.jhazmat.2012.03.068
Gupta, V. K., Fakhri, A., Agarwal, S., Bharti, A. K., Naji, M., and Tkachev, A. G. (2018). Preparation and characterization of TiO2 nanofibers by hydrothermal method for removal of Benzodiazepines (Diazepam) from liquids as catalytic ozonation and adsorption processes. J. Mol. Liq. 249, 1033–1038. doi:10.1016/j.molliq.2017.11.144
Hamadanian, M., Akbari, A., and Jabbari, V. (2011). Electrospun titanium dioxide nanofibers: fabrication, properties and its application in photo-oxidative degradation of methyl orange (MO). Fibers Polym. 12, 880–885. doi:10.1007/s12221-011-0880-z
Han, X., Yao, B., Li, K., Zhu, W., and Zhang, X. (2020). Preparation and photocatalytic performances of WO3/TiO2 composite nanofibers. J. Chem. 2020, 1–12. doi:10.1155/2020/2390486
Han, Z., Li, S., Chu, J., and Chen, Y. (2013). Electrospun Pd-doped ZnO nanofibers for enhanced photocatalytic degradation of methylene blue. J. Solgel Sci. Technol. 66, 139–144. doi:10.1007/s10971-013-2978-9
He, Y., Sang, W., Lu, W., Zhang, W., Zhan, C., and Jia, D. (2022). Recent advances of emerging organic pollutants degradation in environment by non-thermal plasma technology: a review. Water 14, 1351. doi:10.3390/w14091351
Hernández, S., Hidalgo, D., Sacco, A., Chiodoni, A., Lamberti, A., Cauda, V., et al. (2015). Comparison of photocatalytic and transport properties of TiO2 and ZnO nanostructures for solar-driven water splitting. Phys. Chem. Chem. Phys. 17, 7775–7786. doi:10.1039/c4cp05857g
Hlabangwane, K., Matshitse, R., Managa, M., and Nyokong, T. (2023). The application of Sn(IV)Cl2 and In(III)Cl porphyrin-dyed TiO2 nanofibers in photodynamic antimicrobial chemotherapy for bacterial inactivation in water. Photodiagnosis Photodyn. Ther. 44, 103795. doi:10.1016/j.pdpdt.2023.103795
Impellitteri, F., Multisanti, C. R., Rusanova, P., Piccione, G., Falco, F., and Faggio, C. (2023). Exploring the impact of contaminants of emerging concern on fish and invertebrates physiology in the mediterranean sea. Biology 12, 767. doi:10.3390/biology12060767
Jafri, N. N. M., Jaafar, J., Alias, N. H., Samitsu, S., Aziz, F., Salleh, W. N. W., et al. (2021). Synthesis and characterization of titanium dioxide hollow nanofiber for photocatalytic degradation of methylene blue dye. Membranes 11, 581. doi:10.3390/membranes11080581
Jafri, N. N. M., Jaafar, J., Aziz, F., Salleh, W. N. W., Yusof, N., Othman, M. H. D., et al. (2022). Development of free-standing titanium dioxide hollow nanofibers photocatalyst with enhanced recyclability. Membranes 12, 342. doi:10.3390/membranes12030342
Javid, A., Nasseri, S., Mesdaghinia, A., Mahvi, A. H., Alimohammadi, M., Aghdam, R. M., et al. (2013). Performance of photocatalytic oxidation of tetracycline in aqueous solution by TiO2 nanofibers. J. Environ. Health Sci. Eng. 11, 24. doi:10.1186/2052-336x-11-24
Jian, S., Tian, Z., Hu, J., Zhang, K., Zhang, L., Duan, G., et al. (2022). Enhanced visible light photocatalytic efficiency of La-doped ZnO nanofibers via electrospinning-calcination technology. Adv. Powder Mater 1, 100004. doi:10.1016/j.apmate.2021.09.004
Kanjwal, M. A., Chronakis, I. S., and Barakat, N. A. M. (2015). Electrospun NiO, ZnO and composite NiO–ZnO nanofibers/photocatalytic degradation of dairy effluent. Ceram. Int. 41, 12229–12236. doi:10.1016/j.ceramint.2015.06.045
Kasonga, T. K., Coetzee, M. A. A., Kamika, I., Ngole-Jeme, V. M., and Benteke Momba, M. N. (2021). Endocrine-disruptive chemicals as contaminants of emerging concern in wastewater and surface water: a review. J. Environ. Manage 277, 111485. doi:10.1016/j.jenvman.2020.111485
Keşir, M. K., Sökmen, M., and Bıyıklıoğlu, Z. (2021). Photocatalytic efficiency of metallo phthalocyanine sensitized TiO2 (MPc/TiO2) nanocomposites for Cr(VI) and antibiotic amoxicillin. Water 13, 2174. doi:10.3390/w13162174
Khoo, Y. S., Goh, P. S., Lau, W. J., Ismail, A. F., Abdullah, M. S., Mohd Ghazali, N. H., et al. (2022). Removal of emerging organic micropollutants via modified-reverse osmosis/nanofiltration membranes: a review. Chemosphere 305, 135151. doi:10.1016/j.chemosphere.2022.135151
Kondrakov, A. O., Ignatev, A. N., Lunin, V. V., Frimmel, F. H., Bräse, S., and Horn, H. (2016). Roles of water and dissolved oxygen in photocatalytic generation of free OH radicals in aqueous TiO2 suspensions: an isotope labeling study. Appl. Catal. B 182, 424–430. doi:10.1016/j.apcatb.2015.09.038
Kudhier, M. A., Alkareem, RASA, and Sabry, R. S. (2021). Enhanced photocatalytic activity of TiO2-CdS composite nanofibers under sunlight irradiation. J. Mech. Behav. Mater 30, 213–219. doi:10.1515/jmbm-2021-0022
Kumar, A., Dixit, U., Singh, K., Prakash Gupta, S., and Jamal Beg M, S. (2021). “Dyes and pigments - novel applications and waste treatment,” in Structure and properties of dyes and pigments. Editor R. Papadakis (London, United Kingdom: IntechOpen).
Kumar, S., Ahlawat, W., Bhanjana, G., Heydarifard, S., Nazhad, M. M., and Dilbaghi, N. (2014). Nanotechnology-based water treatment strategies. J. Nanosci. Nanotechnol. 14, 1838–1858. doi:10.1166/jnn.2014.9050
Kumari, H., Suman, S., Ranga, R., Chahal, S., Devi, S., Sharma, S., et al. (2023). A review on photocatalysis used for wastewater treatment: dye degradation. Water Air Soil Pollut. 234, 349. doi:10.1007/s11270-023-06359-9
Lan, K., Wang, R., Zhang, W., Zhao, Z., Elzatahry, A., Zhang, X., et al. (2018). Mesoporous TiO2 microspheres with precisely controlled crystallites and architectures. Chem 4, 2436–2450. doi:10.1016/j.chempr.2018.08.008
Lee, C. G., Na, K. H., Kim, W. T., Park, D. C., Yang, W. H., and Choi, W. Y. (2019). TiO2/ZnO nanofibers prepared by electrospinning and their photocatalytic degradation of methylene blue compared with TiO2 nanofibers. Appl. Sci. 9, 3404. doi:10.3390/app9163404
Lellis, B., Fávaro-Polonio, C. Z., Pamphile, J. A., and Polonio, J. C. (2019). Effects of textile dyes on health and the environment and bioremediation potential of living organisms. Biotechnol. Res. Innov. 3, 275–290. doi:10.1016/j.biori.2019.09.001
Lhomme, L., Brosillon, S., and Wolbert, D. (2008). Photocatalytic degradation of pesticides in pure water and a commercial agricultural solution on TiO2 coated media. Chemosphere 70, 381–386. doi:10.1016/j.chemosphere.2007.07.004
Li, D., and Xia, Y. (2003). Fabrication of titania nanofibers by electrospinning. Nano Lett. 3, 555–560. doi:10.1021/nl034039o
Li, M., Yin, J. J., Wamer, W. G., and Lo, Y. M. (2014). Mechanistic characterization of titanium dioxide nanoparticle-induced toxicity using electron spin resonance. J. Food Drug Anal. 22, 76–85. doi:10.1016/j.jfda.2014.01.006
Liao, X., Li, T. T., Ren, H. T., Zhang, X., Shen, B., Lin, J. H., et al. (2022). Construction of BiOI/TiO2 flexible and hierarchical S-scheme heterojunction nanofibers membranes for visible-light-driven photocatalytic pollutants degradation. Sci. Total Environ. 806, 150698. doi:10.1016/j.scitotenv.2021.150698
Lin, L., Jiang, W., Nasr, M., Bechelany, M., Miele, P., Wang, H., et al. (2019). Enhanced visible light photocatalysis by TiO2-BN enabled electrospinning of nanofibers for pharmaceutical degradation and wastewater treatment. Photochem Photobiol. Sci. 18, 2921–2930. doi:10.1039/c9pp00304e
Liu, L., Liu, Z., Yang, Y., Geng, M., Zou, Y., Shahzad, M. B., et al. (2018). Photocatalytic properties of Fe-doped ZnO electrospun nanofibers. Ceram. Int. 44, 19998–20005. doi:10.1016/j.ceramint.2018.07.268
Liu, Q. (2020). Pollution and treatment of dye waste-water. IOP Conf. Ser. Earth Environ. Sci. 514, 052001. doi:10.1088/1755-1315/514/5/052001
Ludwig, T., Bohr, C., Queraltó, A., Frohnhoven, R., Fischer, T., and Mathur, S. (2018). Inorganic nanofibers by electrospinning techniques and their application in energy conversion and storage systems. Semicond. Semimetals, 1–70. doi:10.1016/bs.semsem.2018.04.003
Lv, Y., Chen, F., Tang, Y., and Chen, Z. (2021). Synthesis of titania fibers by electrospinning and its photocatalytic degradation properties. Charact. Appl. 4, 94–101. doi:10.24294/can.v4i1.1329
Ma, D., Xin, Y., Gao, M., and Wu, J. (2014). Fabrication and photocatalytic properties of cationic and anionic S-doped TiO2 nanofibers by electrospinning. Appl. Catal. B 147, 49–57. doi:10.1016/j.apcatb.2013.08.004
Ma, Y., Liu, H., Wu, J., Yuan, L., Wang, Y., Du, X., et al. (2019). The adverse health effects of bisphenol A and related toxicity mechanisms. Environ. Res. 176, 108575. doi:10.1016/j.envres.2019.108575
Mahmood, T., Momin, S., Ali, R., Naeem, A., and Khan, A. (2022). “Wastewater treatment,” in Technologies for removal of emerging contaminants from wastewater. Editors M. Ince, and O. Kaplan Ince, 1–20.
Mahy, J., Cerfontaine, V., Poelman, D., Devred, F., Gaigneaux, E., Heinrichs, B., et al. (2018). Highly efficient low-temperature N-doped TiO2 catalysts for visible light photocatalytic applications. Materials 11, 584. doi:10.3390/ma11040584
Mali, S. S., Kim, H., Jang, W. Y., Park, H. S., Patil, P. S., and Hong, C. K. (2013). Novel synthesis and characterization of mesoporous ZnO nanofibers by electrospinning technique. ACS Sustain Chem. Eng. 1, 1207–1213. doi:10.1021/sc400153j
Mapukata, S., and Nyokong, T. (2020). Development of phthalocyanine functionalised TiO2 and ZnO nanofibers for photodegradation of methyl orange. New J. Chem. 44, 16340–16350. doi:10.1039/d0nj03326j
Marien, C. B. D., Marchal, C., Koch, A., Robert, D., and Drogui, P. (2017). Sol-gel synthesis of TiO2 nanoparticles: effect of Pluronic P123 on particle’s morphology and photocatalytic degradation of paraquat. Environ. Sci. Pollut. Res. 24, 12582–12588. doi:10.1007/s11356-016-7681-2
Massima Mouele, E. S., Tijani, J. O., Badmus, K. O., Pereao, O., Babajide, O., Zhang, C., et al. (2021). Removal of pharmaceutical residues from water and wastewater using dielectric barrier discharge methods—a review. Int. J. Environ. Res. Public Health 18, 1683. doi:10.3390/ijerph18041683
Michałowicz, J. (2014). Bisphenol A – sources, toxicity and biotransformation. Environ. Toxicol. Pharmacol. 37, 738–758. doi:10.1016/j.etap.2014.02.003
Mkhondwane, S. T., Matshitse, R., and Nyokong, T. (2022). Porphyrin-graphitic carbon nitride quantum dots decorated on titanium dioxide electrospun nanofibers for photocatalytic degradation of organic pollutants. J. Coord. Chem. 75, 2150–2169. doi:10.1080/00958972.2022.2132153
Modan, E. M., and Plaiasu, A. G. (2020). Advantages and disadvantages of chemical methods in the elaboration of nanomaterials. Ann. “Dunarea de Jos” Univ. Galati Fascicle IX, Metallurgy Mater. Sci. 43, 53–60. doi:10.35219/mms.2020.1.08
Mondal, K., Bhattacharyya, S., and Sharma, A. (2014). Photocatalytic degradation of naphthalene by electrospun mesoporous carbon-doped anatase TiO2 nanofiber mats. Ind. Eng. Chem. Res. 53, 18900–18909. doi:10.1021/ie5025744
Mukhopadhyay, A., Duttagupta, S., and Mukherjee, A. (2022). Emerging organic contaminants in global community drinking water sources and supply: a review of occurrence, processes and remediation. J. Environ. Chem. Eng. 10, 107560. doi:10.1016/j.jece.2022.107560
Na, K. H., Jang, K. P., Kim, S. W., and Choi, W. Y. (2021a). Fabrication of electrospun Ni0.5Zn0.5Fe2O4 nanofibers using polyvinyl pyrrolidone precursors and electromagnetic wave absorption performance improvement. Polymers 13, 4247. doi:10.3390/polym13234247
Na, K. H., Kim, B. S., Yoon, H. S., Song, T. H., Kim, S. W., Cho, C. H., et al. (2021b). Fabrication and photocatalytic properties of electrospun Fe-doped TiO2 nanofibers using polyvinyl pyrrolidone precursors. Polymers 13, 2634. doi:10.3390/polym13162634
Nada, E. A., El-Maghrabi, H. H., Raynaud, P., Ali, H. R., Abd El-Wahab, S., Sabry, D. Y., et al. (2022). Enhanced photocatalytic activity of WS2/TiO2 nanofibers for degradation of phenol under visible light irradiation. Inorganics 10, 54. doi:10.3390/inorganics10040054
Naderi, H., Hajati, S., Ghaedi, M., and Espinos, J. P. (2019). Highly selective few-ppm NO gas-sensing based on necklace-like nanofibers of ZnO/CdO n-n type I heterojunction. Sens. Actuators B Chem. 297, 126774. doi:10.1016/j.snb.2019.126774
Nakonieczny, D. S., Kern, F., Dufner, L., Dubiel, A., Antonowicz, M., and Matus, K. (2021). Effect of calcination temperature on the phase composition, morphology, and thermal properties of ZrO2 and Al2O3 modified with APTES (3-aminopropyltriethoxysilane). Materials 14, 6651. doi:10.3390/ma14216651
Nataraj, S. K., Yang, K. S., and Aminabhavi, T. M. (2012). Polyacrylonitrile-based nanofibers—a state-of-the-art review. Prog. Polym. Sci. 37, 487–513. doi:10.1016/j.progpolymsci.2011.07.001
Natarajan, T. S., Mozhiarasi, V., and Tayade, R. J. (2021). Nitrogen doped titanium dioxide (N-TiO2): synopsis of synthesis methodologies, doping mechanisms, property evaluation and visible light photocatalytic applications. Photochem 1, 371–410. doi:10.3390/photochem1030024
Navidpour, A. H., Abbasi, S., Li, D., Mojiri, A., and Zhou, J. L. (2023). Investigation of advanced oxidation process in the presence of TiO2 semiconductor as photocatalyst: property, principle, kinetic analysis, and photocatalytic activity. Catalysts 13, 232. doi:10.3390/catal13020232
Ng, B., Quinete, N., Maldonado, S., Lugo, K., Purrinos, J., Briceño, H., et al. (2021). Understanding the occurrence and distribution of emerging pollutants and endocrine disruptors in sensitive coastal South Florida Ecosystems. Sci. Total Environ. 757, 143720. doi:10.1016/j.scitotenv.2020.143720
Nirmala, R., Kim, H. Y., Navamathavan, R., Yi, C., Won, J. J., Jeon, K., et al. (2012). Photocatalytic activities of electrospun tin oxide doped titanium dioxide nanofibers. Ceram. Int. 38, 4533–4540. doi:10.1016/j.ceramint.2012.02.030
Norouzi, M., Fazeli, A., and Tavakoli, O. (2020). Phenol contaminated water treatment by photocatalytic degradation on electrospun Ag/TiO2 nanofibers: optimization by the response surface method. J. Water Process Eng. 37, 101489. doi:10.1016/j.jwpe.2020.101489
Nuansing, W., Ninmuang, S., Jarernboon, W., Maensiri, S., and Seraphin, S. (2006). Structural characterization and morphology of electrospun TiO2 nanofibers. Mater Sci. Eng. B 131, 147–155. doi:10.1016/j.mseb.2006.04.030
Panigrahy, N., Priyadarshini, A., Sahoo, M. M., Verma, A. K., Daverey, A., and Sahoo, N. K. (2022). A comprehensive review on eco-toxicity and biodegradation of phenolics: recent progress and future outlook. Environ. Technol. Innov. 27, 102423. doi:10.1016/j.eti.2022.102423
Pantò, F., Dahrouch, Z., Saha, A., Patanè, S., Santangelo, S., and Triolo, C. (2021). Photocatalytic degradation of methylene blue dye by porous zinc oxide nanofibers prepared via electrospinning: when defects become merits. Appl. Surf. Sci. 557, 149830. doi:10.1016/j.apsusc.2021.149830
Papagiannaki, D., Belay, M. H., Gonçalves, N. P. F., Robotti, E., Bianco-Prevot, A., Binetti, R., et al. (2022). From monitoring to treatment, how to improve water quality: the pharmaceuticals case. Chem. Eng. J. Adv. 10, 100245. doi:10.1016/j.ceja.2022.100245
Parrino, F., D’Arienzo, M., Callone, E., Conta, R., Di Credico, B., Mascotto, S., et al. (2021). TiO2 containing hybrid nanocomposites with active–passive oxygen scavenging capability. Chem. Eng. J. 417, 129135. doi:10.1016/j.cej.2021.129135
Pascariu, P., Cojocaru, C., Homocianu, M., and Samoila, P. (2022b). Tuning of Sm3+ and Er3+-doped TiO2 nanofibers for enhancement of the photocatalytic performance: optimization of the photodegradation conditions. J. Environ. Manage 316, 115317. doi:10.1016/j.jenvman.2022.115317
Pascariu, P., Cojocaru, C., Homocianu, M., Samoila, P., Dascalu, A., and Suchea, M. (2022a). New La3+ doped TiO2 nanofibers for photocatalytic degradation of organic pollutants: effects of thermal treatment and doping loadings. Ceram. Int. 48, 4953–4964. doi:10.1016/j.ceramint.2021.11.033
Pascariu, P., Homocianu, M., Cojocaru, C., Samoila, P., Airinei, A., and Suchea, M. (2019). Preparation of La doped ZnO ceramic nanostructures by electrospinning–calcination method: effect of La3+ doping on optical and photocatalytic properties. Appl. Surf. Sci. 476, 16–27. doi:10.1016/j.apsusc.2019.01.077
Pathak, S., Pant, K. K., and Kaushal, P. (2023). Analysis of naphthalene adsorption from wastewater using activated and non-activated biochar produced from bagasse. Biomass Convers. Biorefin. doi:10.1007/s13399-023-04070-7
Paumo, H. K., Dalhatou, S., Katata-Seru, L. M., Kamdem, B. P., Tijani, J. O., Vishwanathan, V., et al. (2021). TiO2 assisted photocatalysts for degradation of emerging organic pollutants in water and wastewater. J. Mol. Liq. 331, 115458. doi:10.1016/j.molliq.2021.115458
Paunović, V., Rellán-Piñeiro, M., López, N., and Pérez-Ramírez, J. (2021). Activity differences of rutile and anatase TiO2 polymorphs in catalytic HBr oxidation. Catal. Today 369, 221–226. doi:10.1016/j.cattod.2020.03.036
Pavel, M., Anastasescu, C., State, R. N., Vasile, A., Papa, F., and Balint, I. (2023). Photocatalytic degradation of organic and inorganic pollutants to harmless end products: assessment of practical application potential for water and air cleaning. Catalysts 13, 380. doi:10.3390/catal13020380
Pei, C. C., and Leung, W. W. F. (2013). Photocatalytic degradation of Rhodamine B by TiO2/ZnO nanofibers under visible-light irradiation. Sep. Purif. Technol. 114, 108–116. doi:10.1016/j.seppur.2013.04.032
Prabhu, P. (2019). “Nanofibers for medical diagnosis and therapy,” in Handbook of nanofibers (Cham: Springer International Publishing), 831–867.
Qu, Y., Huang, R., Qi, W., Shi, M., Su, R., and He, Z. (2020). Controllable synthesis of ZnO nanoflowers with structure-dependent photocatalytic activity. Catal. Today 355, 397–407. doi:10.1016/j.cattod.2019.07.056
Ramírez-Malule, H., Quiñones-Murillo, D. H., and Manotas-Duque, D. (2020). Emerging contaminants as global environmental hazards. A bibliometric analysis. Emerg. Contam. 6, 179–193. doi:10.1016/j.emcon.2020.05.001
Rongan, H., Haijuan, L., Huimin, L., Difa, X., and Liuyang, Z. (2020). S-scheme photocatalyst Bi2O3/TiO2 nanofiber with improved photocatalytic performance. J. Mater Sci. Technol. 52, 145–151. doi:10.1016/j.jmst.2020.03.027
Roongraung, K., Chuangchote, S., Laosiripojana, N., and Sagawa, T. (2020). Electrospun Ag-TiO2 nanofibers for photocatalytic glucose conversion to high-value chemicals. ACS Omega 5, 5862–5872. doi:10.1021/acsomega.9b04076
Ruiz-Ramírez, L. R., Torres-Pérez, J., Medellín-Castillo, N., and Reyes-López, S. Y. (2023). Photocatalytic degradation of oxytetracycline by SiO2–TiO2–Ag electrospun fibers. Solid State Sci. 140, 107188. doi:10.1016/j.solidstatesciences.2023.107188
Sabouni, R., and Gomaa, H. (2019). Photocatalytic degradation of pharmaceutical micro-pollutants using ZnO. Environ. Sci. Pollut. Res. 26, 5372–5380. doi:10.1007/s11356-018-4051-2
Samadi, M., Pourjavadi, A., and Moshfegh, A. Z. (2014). Role of CdO addition on the growth and photocatalytic activity of electrospun ZnO nanofibers: UV vs. visible light. Appl. Surf. Sci. 298, 147–154. doi:10.1016/j.apsusc.2014.01.146
Samadipakchin, P., Mortaheb, H. R., and Zolfaghari, A. (2017). ZnO nanotubes: preparation and photocatalytic performance evaluation. J. Photochem Photobiol. A Chem. 337, 91–99. doi:10.1016/j.jphotochem.2017.01.018
Samanta, P., Bagchi, S., and Mishra, S. (2015). Synthesis and sensing characterization of ZnO nanofibers prepared by electrospinning. Mater Today Proc. 2, 4499–4502. doi:10.1016/j.matpr.2015.10.061
Schneider, J. T., Firak, D. S., Ribeiro, R. R., and Peralta-Zamora, P. (2020). Use of scavenger agents in heterogeneous photocatalysis: truths, half-truths, and misinterpretations. Phys. Chem. Chem. Phys. 22, 15723–15733. doi:10.1039/d0cp02411b
Secundino-Sánchez, O., Díaz-Reyes, J., Sánchez-Ramírez, J. F., Arias-Cerón, J. S., Galván-Arellano, M., and Vázquez-Cuchillo, O. (2022b). Controlled synthesis of electrospun TiO2 nanofibers and their photocatalytic application in the decolouration of Remazol Black B azo dye. Catal. Today 392–393, 13–22. doi:10.1016/j.cattod.2021.10.003
Secundino-Sánchez, O., Mendoza-Álvarez, J. G., Díaz-Reyes, J., Sánchez-Ramírez, J. F., Zaca-Moran, O., and Herrera-Pérez, J. L. (2022a). Structural and optical characterization of electrospun TiO2 nanofibers using titanium tetrabutoxide and titanium isopropoxide as precursors for photocatalytic applications. Colloids Surf. A Physicochem Eng. Asp. 649, 129505. doi:10.1016/j.colsurfa.2022.129505
Sekar, A. D., Muthukumar, H., Chandrasekaran, N. I., and Matheswaran, M. (2018). Photocatalytic degradation of naphthalene using calcined Fe–ZnO/PVA nanofibers. Chemosphere 205, 610–617. doi:10.1016/j.chemosphere.2018.04.131
Serwecińska, L. (2020). Antimicrobials and antibiotic-resistant bacteria: a risk to the environment and to public health. Water 12, 3313. doi:10.3390/w12123313
Shendokar, S., Kelkar, A., Mohan, R., Bolick, R., and Chandekar, G. (2008). “Effect of sintering temperature on mechanical properties of electrospun silica nanofibers,” in Volume 13: nano-manufacturing technology; and micro and nano systems, parts A and B. ASMEDC, Boston, Massachusetts, USA, 1133–1138. doi:10.1115/IMECE2008-68033
Shi, H., Zhou, M., Song, D., Pan, X., Fu, J., Zhou, J., et al. (2014). Highly porous SnO2/TiO2 electrospun nanofibers with high photocatalytic activities. Ceram. Int. 40, 10383–10393. doi:10.1016/j.ceramint.2014.02.124
Singh, J., Gupta, P., and Das, A. (2021). “Dyes from textile industry wastewater as emerging contaminants in agricultural fields,” in Sustainable agriculture reviews. Editors V. Kumar Singh, R. Singh, and E. Lichtfouse (Switzerland: Springer), 109–129.
Singh, N., and Yadav, S. S. (2022). A review on health benefits of phenolics derived from dietary spices. Curr. Res. Food Sci. 5, 1508–1523. doi:10.1016/j.crfs.2022.09.009
Singh, Z., and Chadha, P. (2016). Textile industry and occupational cancer. J. Occup. Med. Toxicol. 11, 39. doi:10.1186/s12995-016-0128-3
Siwińska-Stefańska, K., Kubiak, A., Piasecki, A., Goscianska, J., Nowaczyk, G., Jurga, S., et al. (2018). TiO2-ZnO binary oxide systems: comprehensive characterization and tests of photocatalytic activity. Materials 11, 841. doi:10.3390/ma11050841
Someswararao, M. V., Dubey, R. S., and Subbarao, P. S. V. (2021). Electrospun composite nanofibers prepared by varying concentrations of TiO2/ZnO solutions for photocatalytic applications. J. Photochem Photobiol. 6, 100016. doi:10.1016/j.jpap.2021.100016
Song, Y. S., Cho, N. I., Lee, M. H., Kim, B. Y., and Lee, D. Y. (2016). Photocatalytic activity of W-doped TiO2 nanofibers for methylene blue dye degradation. J. Nanosci. Nanotechnol. 16, 1831–1833. doi:10.1166/jnn.2016.11970
Soo, J. Z., Ang, B. C., and Ong, B. H. (2018). Influence of calcination on the morphology and crystallinity of titanium dioxide nanofibers towards enhancing photocatalytic dye degradation. Mater Res. Express 6, 025039. doi:10.1088/2053-1591/aaf013
Sun, N., Tian, Q., Bian, W., Wang, X., Dou, H., Li, C., et al. (2023). Highly sensitive and lower detection-limit NO2 gas sensor based on Rh-doped ZnO nanofibers prepared by electrospinning. Appl. Surf. Sci. 614, 156213. doi:10.1016/j.apsusc.2022.156213
Suphankij, S., Mekprasart, W., and Pecharapa, W. (2013). Photocatalytic of N-doped TiO2 nanofibers prepared by electrospinning. Energy Procedia 34, 751–756. doi:10.1016/j.egypro.2013.06.810
Tahraoui, H., Belhadj, A. E., Triki, Z., Boudellal, N. R., Seder, S., Amrane, A., et al. (2023). Mixed coagulant-flocculant optimization for pharmaceutical effluent pretreatment using response surface methodology and Gaussian process regression. PSEP 169, 909–927. doi:10.1016/j.psep.2022.11.045
Tan, D., Lee, W., Kim, Y. E., Ko, Y. N., Youn, M. H., Jeon, Y. E., et al. (2020). SnO2/ZnO composite hollow nanofiber electrocatalyst for efficient CO2 reduction to formate. ACS Sustain Chem. Eng. 8, 10639–10645. doi:10.1021/acssuschemeng.0c03481
Tanveer, M., Guyer, G. T., and Abbas, G. (2019). Photocatalytic degradation of ibuprofen in water using TiO2 and ZnO under artificial UV and solar irradiation. Water Environ. Res. 91, 822–829. doi:10.1002/wer.1104
Thakur, N., Thakur, N., Bhullar, V., Sharma, S., Mahajan, A., Kumar, K., et al. (2021). TiO2 nanofibers fabricated by electrospinning technique and degradation of MO dye under UV light. Z Krist. Cryst. Mater 236, 239–250. doi:10.1515/zkri-2021-2025
Thakur, S., Kaur, M., Lim, W. F., and Lal, M. (2020). Fabrication and characterization of electrospun ZnO nanofibers; antimicrobial assessment. Mater Lett. 264, 127279. doi:10.1016/j.matlet.2019.127279
Toriello, M., Afsari, M., Shon, H., and Tijing, L. (2020). Progress on the fabrication and application of electrospun nanofiber composites. Membranes 10, 204. doi:10.3390/membranes10090204
Tshabalala, Z. P., Swart, H. C., and Motaung, D. E. (2021). Fabrication of TiO2 nanofibers based sensors for enhanced CH4 performance induced by notable surface area and acid treatment. Vacuum 187, 110102. doi:10.1016/j.vacuum.2021.110102
Usmani, Z., Sharma, M., Gaffey, J., Sharma, M., Dewhurst, R. J., Moreau, B., et al. (2022). Valorization of dairy waste and by-products through microbial bioprocesses. Bioresour. Technol. 346, 126444. doi:10.1016/j.biortech.2021.126444
Vakhrushev, A. Y., and itsova, T. B. (2021). TiO2 and TiO2/Ag nanofibers: template synthesis, structure, and photocatalytic properties. J. Porous Mater 28, 1023–1030. doi:10.1007/s10934-021-01061-9
Vasiljević, Z. Ž., Dojčinović, M. P., Vujančević, J. D., Spreitzer, M., Kovač, J., Bartolić, D., et al. (2021). Exploring the impact of calcination parameters on the crystal structure, morphology, and optical properties of electrospun Fe2TiO5 nanofibers. RSC Adv. 11, 32358–32368. doi:10.1039/d1ra05748k
Volney, G., Tatusov, M., Yen, A. C., and Karamyan, N. (2018). Naphthalene toxicity: methemoglobinemia and acute intravascular hemolysis. Cureus 10, e3147. doi:10.7759/cureus.3147
Waleng, N. J., and Nomngongo, P. N. (2022). Occurrence of pharmaceuticals in the environmental waters: african and Asian perspectives. Environ. Chem. Ecotoxicol. 4, 50–66. doi:10.1016/j.enceco.2021.11.002
Wang, L., Zhao, Y., and Zhang, J. (2017). Photochemical removal of SO2 over TiO2 -based nanofibers by a dry photocatalytic oxidation process. Energy fuels. 31, 32711. doi:10.1021/acs.energyfuels.7b01514
Wang, T., and Cheng, L. (2021). Hollow hierarchical TiO2-SnO2-TiO2 composite nanofibers with increased active-sites and charge transfer for enhanced acetone sensing performance. Sens. Actuators B Chem. 334, 129644. doi:10.1016/j.snb.2021.129644
Wang, T., Gao, Y., Tang, T., Bian, H., Zhang, Z., Xu, J., et al. (2019). Preparation of ordered TiO2 nanofibers/nanotubes by magnetic field assisted electrospinning and the study of their photocatalytic properties. Ceram. Int. 45, 14404–14410. doi:10.1016/j.ceramint.2019.04.158
Wang, Y., Cheng, J., Yu, S., Alcocer, E. J., Shahid, M., Wang, Z., et al. (2016). Synergistic effect of N-decorated and Mn2+ doped ZnO nanofibers with enhanced photocatalytic activity. Sci. Rep. 6, 32711. doi:10.1038/srep32711
Wang, Z., and Lou, X. W. (2012). TiO2 nanocages: fast synthesis, interior functionalization and improved lithium storage properties. Adv. Mater 24, 4124–4129. doi:10.1002/adma.201104546
Wróbel, J., and Piechota, J. (2008). On the structural stability of ZnO phases. Solid State Commun. 146, 324–329. doi:10.1016/j.ssc.2008.03.001
Yan, S. H., Ma, S. Y., Li, W. Q., Xu, X. L., Cheng, L., Song, H. S., et al. (2015). Synthesis of SnO2-ZnO heterostructured nanofibers for enhanced ethanol gas-sensing performance. Sens. Actuators B Chem. 221, 88–95. doi:10.1016/j.snb.2015.06.104
You, Y., Zhang, S., Wan, L., and Xu, D. (2012). Preparation of continuous TiO2 fibers by sol–gel method and its photocatalytic degradation on formaldehyde. Appl. Surf. Sci. 258, 3469–3474. doi:10.1016/j.apsusc.2011.11.099
Yun, E. T., Yoo, H. Y., Kim, W., Kim, H. E., Kang, G., Lee, H., et al. (2017). Visible-light-induced activation of periodate that mimics dye-sensitization of TiO2: simultaneous decolorization of dyes and production of oxidizing radicals. Appl. Catal. B 203, 475–484. doi:10.1016/j.apcatb.2016.10.029
Zagho, M. M., and Elzatahry, A. (2016). “Recent trends in electrospinning of polymer nanofibers and their applications as templates for metal oxide nanofibers preparation,” in Electrospinning - material, techniques, and biomedical applications (London, United Kingdom: InTech).
Zhang, A., Liang, Y., Zhang, H., Geng, Z., and Zeng, J. (2021). Doping regulation in transition metal compounds for electrocatalysis. Chem. Soc. Rev. 50, 9817–9844. doi:10.1039/d1cs00330e
Zhang, M., Zhao, W., Wu, J., Li, Z., Xue, L., Yang, F., et al. (2023a). Promising rare-earth-doped, electrospun, ZnO nanofiber N-type semiconductor for betavoltaic batteries. ACS Omega 8, 17644–17652. doi:10.1021/acsomega.3c00039
Zhang, S., Xu, S., Hu, D., Zhang, C., Che, J., and Song, Y. (2018). Formation of TiO2 nanoribbons by anodization under high current density. Mater Res. Bull. 103, 205–210. doi:10.1016/j.materresbull.2018.02.014
Zhang, X.-bing, Hu, Y.-ping, Yang, W., and M-bao, F. (2023b). Ag-loaded and Pd-loaded ZnO nanofiber membranes: preparation via electrospinning and application in photocatalytic antibacterial and dye degradation. Appl. Nanosci. 13, 1495–1506. doi:10.1007/s13204-021-02056-3
Zhang, Y., Chen, Y., Hou, L., Guo, F., Liu, J., Qiu, S., et al. (2017). Pine-branch-like TiO2 nanofibrous membrane for high efficiency strong corrosive emulsion separation. J. Mater Chem. A Mater 5, 16134–16138. doi:10.1039/c7ta00833c
Zhang, Z., Shao, C., Zhang, L., Li, X., and Liu, Y. (2010). Electrospun nanofibers of V-doped TiO2 with high photocatalytic activity. J. Colloid Interface Sci. 351, 57–62. doi:10.1016/j.jcis.2010.05.067
Keywords: electrospinning, photocatalysis, TiO2 nanofibers, ZnO nanofibers, wastewater treatment, organic pollutants
Citation: Mapukata S, Shingange K and Mokhena T (2023) Review of the recent advances on the fabrication, modification and application of electrospun TiO2 and ZnO nanofibers for the treatment of organic pollutants in wastewater. Front. Chem. Eng. 5:1304128. doi: 10.3389/fceng.2023.1304128
Received: 28 September 2023; Accepted: 15 November 2023;
Published: 04 December 2023.
Edited by:
Tshimangadzo Munonde, University of South Africa, South AfricaReviewed by:
Lethula Mofokeng, University of Pretoria, South AfricaRudzani Ratshiedana, University of South Africa, South Africa
Copyright © 2023 Mapukata, Shingange and Mokhena. This is an open-access article distributed under the terms of the Creative Commons Attribution License (CC BY). The use, distribution or reproduction in other forums is permitted, provided the original author(s) and the copyright owner(s) are credited and that the original publication in this journal is cited, in accordance with accepted academic practice. No use, distribution or reproduction is permitted which does not comply with these terms.
*Correspondence: Sivuyisiwe Mapukata, c2l2dXlpc2l3ZW1AbWludGVrLmNvLnph