- RFF-CMCC European Institute on Economics and the Environment (EIEE), Centro Euro-Mediterraneo Sui Cambiamenti Climatici, Milano, Italy
Energy policies aim at securing energy supply through domestic production or imports have significant consequences for climate change and its long-term impacts on the economy. Recent European energy crisis as a result of extensive reliance on imported Russian natural gas has highlighted the European Union (EU) energy vulnerability and has challenged its climate change commitments. While switching to alternative domestic fossil fuel sources such as coal in some member states has put the EU climate ambitions in jeopardy, it has also provided new opportunities for up-scaling renewable technologies as well as climate stability measures such as direct air capture (DAC). This paper examines the interaction between energy policy and climate stability by considering imported natural gas, domestic coal production, and possible DAC deployment in the EU under two scenarios of full cooperation and full competition among the EU member states. The results suggest that while cooperation induces higher reliance on imported energy, it also provides a strong incentive for DAC uptake. Competition on the other hand, may result in more reliance on domestic coal production and worse climate change outcomes despite the availability of DAC. Therefore, as the EU is striving for a more perfect union, it should consider better alignment of its short-term energy security policies with long-term climate stability ambitions.
1 Introduction
The widespread adoption of innovative technologies often can be contributed to a convolution of multiple social, behavioral, political, and economic factors (Urmee and Md, 2016). Similarly, the uptake of climate change solutions such as carbon removal (CDR) technologies depends on not only their technological readiness but also on an array of socioeconomic factors facilitating or obstructing their development and deployment (Buck, 2016). In the last few months and after the Russian invasion of Ukraine, growing energy security concerns in Europe (Żuk and Żuk, 2022), combined with unequivocal commitments for decarbonization (Buschle and Westphal, 2019), have provided a unique opportunity for large scale deployment of innovative climate solutions that can play a key role in shaping the future of European energy-climate ecosystem.
In the latest assessment report by intergovernmental panel on climate change (IPCC), CDR has been acknowledged as a “necessary element” in keeping global warming well- 2°C and even more essential in staying below 1.5°C (IPCC, 2022). Although there is no consensus among the researchers, policymakers, climate activist, and the general public on the best approach to removing existing carbon dioxide emissions from the atmosphere (Cox et al., 2020), Direct Air Capture (DAC) in particular, has increasingly been considered as a viable solution for achieving net-zero and net-negative climate objectives (Bednar et al., 2021).
There are several technical and economic reasons for the growing interest in commercialization and early adoption of DAC technologies (McQueen et al., 2021)1. First, DAC technology is flexible in the sense that unlike carbon capture and storage (CCS) that applies to stationary sources of highly concentrated emissions such as fossil fuel power plants, DAC process is not limited to an specific location as long as it is in close proximity to reliable energy sources and CO2 storage sites (Erans et al., 2022). Furthermore, it can use either solid sorbents or liquid solvents (McQueen et al., 2021). Second, the capture units are modular and their annual capacity can be scaled up from a few tons to megatons of captured carbon (Hanna et al., 2021). Third, the DAC operation is an industrial process with controllable and verifiable input and output quantities which can potentially alleviate concerns about verification and certification of the DAC plants (Sovacool et al., 2023). Finally, and compared to other CDR approaches, DAC has a relatively small physical footprint and it requires far less land and water resources for its operation while there is no direct threat to existing food or biodiversity systems (Beuttler et al., 2019). Nevertheless, there are still large uncertainties about optimization of the DAC processes at large scale, their integration into renewable energy systems, and the permanent sequestration of the captured carbon which requires further research and investigation
In the past few years, multiple studies have assessed the techno-economic potentials of DAC technologies (Fasihi et al., 2019; National Academies of Sciences Engineering and Medicine, 2019), their limitations (Smith et al., 2016; Erans et al., 2022), and their future prospect (Nemet et al., 2018; Shayegh et al., 2021). However, the integration of DAC into energy-climate system models is still limited and the results are largely dependent on critical assumptions about the cost and the deployment rate of DAC technologies (Chatterjee and Huang, 2020).
This paper, however, takes a different approach by framing the question of DAC deployment against a broader backdrop of energy transition and energy security from an European perspective. It develops an analytical model for energy policy making mechanism in a representative EU member state economy who has a significant dependence on an insecure foreign energy source (e.g., Russian natural gas), and a controversial reliance on domestic fossil fuel production (e.g., coal industry). Furthermore, the European Union (EU), in line with its member states, has set out an ambitious program (i.e., the European Green Deal) to make the EU climate neutral in 2050 (Commission and for Communication, 2021). As a result, a complex array of such climate ambitions and energy security incentives, in addition to Energy Union considerations (Pérez et al., 2019), has created national policies within the EU member states, which are largely misaligned and are hard to navigate through (Maris and Flouros, 2021; Osička and Černoch, 2022).
On the other hand, delayed deployment of CDR initiatives including DAC, would be costly for the EU and would substantially undermine the removal potential to meet the 2100 targets (Galán-Martín et al., 2021). Therefore, there is an urgent need for the alignment of national energy policies with carbon removal up-scaling strategies in a way that both provide energy security and achieve the long-term climate stability objectives. This paper attempts to revisit energy security considerations and priorities in light of climate change commitments by developing and analysing a simple economic model of a major importer and exporter of natural gas (i.e., the EU member states and Russia, respectively) with differentiated climate change preferences under two scenarios: cooperation between the importing entities and competition among them. The results highlight the interconnection of the imported energy price and the incentive to develop and deploy a reliable carbon removal option such as DAC to offset the negative impacts of domestic and imported fossil fuel consumption.
2 Modeling energy security and climate stability
We investigate the interaction between energy security and climate stability efforts through the development of an importer-exporter two-actor model. In this simple model, a group of countries (e.g., the EU member states) represents the importer actor as they are the main importer of the Russian natural gas. On the other hand, Russia whose economy is heavily dependent on the export of fossil fuel energy commodities (and in particular natural gas to Europe), represents the exporter actor. In addition, we distinguish between two types of fossil fuel as primary sources of energy: coal and natural gas. The importer countries like Germany and Poland produce coal domestically but import natural gas from Russia. Consuming either types of fossil fuel generates an economic value but causes climate change damages due to the CO2 emissions resulting from their combustion in energy generation during economic activities. Russia on the other hand, has an abundance of natural gas which is not only sufficient for its domestic consumption but can sufficiently meet the demand of the importer actors. It is important to note that the equations used in the following sections, are highly simplified and only used to demonstrate the high-level associations among different parameters and variables. Therefore, the scope of this study is limited to analyzing the relationship among the key variables and not presenting any quantitative results. Table 1 provides the list of variables and parameters used in this model along with a brief description of each item.
2.1 Sequence of actions
We model the European energy security and climate stability as a sequential game in two stages: In the first stage, the exporter country (i.e., Russia) sets the price of natural gas and then, in the second stage, the importer countries (i.e., the EU member states) decide on the share of domestic production of coal (as a complement to imported natural gas), and the level of DAC deployment, simultaneously. Besides economic value associated with energy consumption, both the exporter and the importer countries suffer from climate damages linked to rising temperatures as a result of greenhouse gas emissions from fossil fuel consumption. Such damages are however, not equal and among other factors, depend on the geographic location and socioeconomic structure of each country’s economy. As a result, the advanced economies of the importer countries have an incentive to opt for the deployment of DAC as a reliable carbon dioxide removal option to alleviate the negative consequence of global warming. Therefore, in the second stage, the importer countries face two choices: 1) the portion of their energy demand to be supplied domestically (i.e., the energy security indicator), and 2) the level of DAC deployment (i.e., the climate stability indicator). Figure 1 shows the dynamics of this game considering energy security and climate stability. For simplicity, we assume the importer actor comprises N identical entities (e.g., the 27 member states of the EU). Further we assume that information about the energy price and quantities, in addition to the level of DAC deployments are publicly available and shared among all actors. This allows us to use the backward induction technique (Aliprantis, 1999) to solve this sequential game with perfect information.
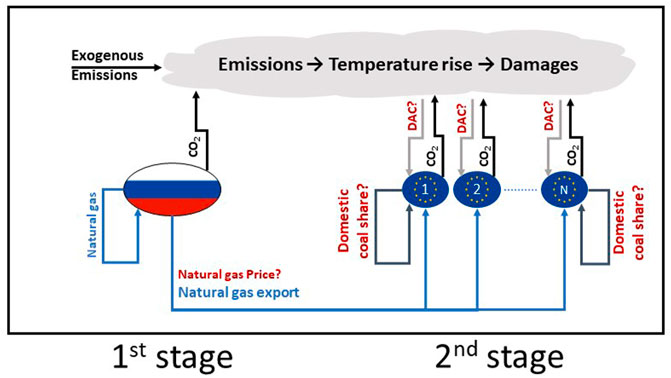
FIGURE 1. Energy security and climate stability dynamics. In the first stage, Russia decides about the price of exported natural gas. In the second stage, the EU countries decide about the share of domestic coal to secure their energy demand, and the level of direct air capture (DAC) to stabilize the climate.
2.1.1 Importer’s problem
In this paper we develop and use a conceptual model of energy economics which assumes that countries benefit from energy consumption but they incur energy expenditures and climate change damage costs. In other words, energy consumption increases their utility while energy acquisition and climate change decrease it. The aim of each country is to maximise their utility by setting the optimal share of energy import and DAC deployment. We start from the second stage, assuming that the price of natural gas (ηg) is known to the importer actor. The utility of each importer entity can be expressed as
with the change in global mean temperature (ΔT) calculated as
where mi is the share of coal in total energy demand (Ei) of an importing entity, and Ri is the carbon removal level through DAC deployment. Parameters ηc, ηg, and ηr reflect the price of coal, natural gas, and DAC respectively. The quadratic DAC cost function is used to account for the required up-scaling investments in carbon removal technologies. Furthermore, the climate damage cost is linked to global mean temperature change and follows a conventional quadratic form (Richard, 1995; Nordhaus, 2017) with parameter δi. In the temperature Eq. 2, parameters θc and θg are used to translate emissions from energy consumption to temperature change for coal and natural gas, respectively. Parameter θr represents the effect of carbon removal on reducing carbon concentration and global mean temperature, subsequently. Finally, Ti represents the contribution of each entity i to global mean temperature change while Tx is the exogenous temperature change due to emissions and other factors beyond the scope of the energy trade between the two actors in this model. To maximize the utility of the importer’s energy consumption, we can derive the optimal values of mi and Ri from the first-order conditions of Eq. 1.
Where Δη = ηg − ηc (i.e., the price difference between imported natural gas and domestic coal) and Δθ = θc − θg (i.e., the warming potential difference between domestic coal emissions and imported natural gas emissions). Solving these two equations simultaneously will provide the optimal values:
If the DAC technology proved to be inefficient (i.e., θr → 0), there will be no incentive to deploy it (i.e.,
2.1.2 Exporter’s problem
In the first stage, the exporter benefits from domestic consumption of natural gas and its export’s revenue while incurring the extraction and climate change damage costs. Therefore, the exporter’s utility can be expressed as
where Ee is the domestic energy demand in the exporting country and ηe is the extraction unit cost. We use a linear climate damage function in the exporter’s utility to reflect the potential benefits of rising temperatures for Russia which moderates the climate change damages.
Taking the first-order condition, the optimal price of natural gas can be obtained from plugging back the results of the second stage into this equation:
This result shows that minimum price of exported natural gas is the average of its extraction cost and the price of coal in the importing countries. In order to gain a better understanding of the market mechanism and its impact on energy security and climate stability efforts, we consider two cases of cooperation and competition among the N importing players. We then compare the optimal share of domestic coal production and DAC deployment between these two cases.
2.2 Cooperation
In the case of full cooperation, the N importing entities act as a block (e.g., the EU negotiates the gas import from Russia on behalf of all its member states). We formulate the utility optimization problem of the importing actor (i.e., the block of N importing entities) as follows:
where the change in global mean temperature (ΔT) is calculated as
The optimization of Eq. 10 with respect to decision variables mi (the share of domestic coal) and Ri (the carbon removal through DAC) provides the following two firs-order conditions:
Solving these equations simultaneously will provide the optimal levels of mi and Ri in this case.
In this case, the price of natural gas in the first stage will be calculated as
Next, we investigate the case when the N importing countries act independently in a competitive market.
2.3 Competition
In this case, every importing entity decides on the level of domestic coal production and DAC deployment independently, bearing in mind that other importing entities are doing the same. We formulate the utility optimization problem of each importing actor as follows:
where the change in global mean temperature (ΔT) is calculated as
The optimization of Eq. 17 with respect to decision variables mi (the share of domestic coal) and Ri (the carbon removal through DAC) provides firs-order conditions similar to those shown in Eqs 3, 4. However, since all N importing entities are similar, the solution should be the same for everyone and therefore, we have Ti = Tj for all i, j ∈ {1, …, N}.
As a result the optimal levels of mi and Ri in this case will be.
In this case, the price of natural gas in the first stage will be calculated as
3 Results
The derivation of optimal natural gas price in the first stage, and energy security (m) and climate stability measures (R) in the second stage for a given number of importing entities (N) allows us to infer important comparisons between the two extreme cases of full cooperation and full competition. To do so, we first consider a case where the natural gas price is exogenously determined by the exporter, and then we investigate the full endogenous case where the price is set in the first stage foreseeing the market conditions in the second stage.
3.1 Exogenous natural gas prices
We evaluate the performance of each case along the energy security dimension by comparing the share of domestic coal in final energy consumption in both cases under the assumption that the all prices are exogenous and therefore, we only focus on the implications of a fixed price of natural gas on the performance of both markets. Proposition 1 unveils the important implication of the well-know free-riding phenomenon in the competition case. While competition disincentives lowering emissions and therefore, encourages the consumption of the dirtier domestic coal, cooperation induces higher imports of (relatively cleaner) natural gas to lower emissions. In other words, cooperation, unintentionally, leads to higher reliance on foreign energy which undermines energy security within the EU.
Proposition 1. For a given N > 1 and an exogenous natural gas price higher than coal price (ηg > ηc), the share of imported natural gas is always (strictly) higher in the full cooperation case compared to the full competition case regardless of the availability of any climate stability measure (e.g., DAC).
Proof. To prove this proposition, we compare the share of domestic coal in both cases using Eq. 21 and Eq. 14. If we can show that the difference between the two shares for any given N and ηm is strictly positive, it means that the EU imports less natural gas in the competition case and therefore, the theorem is proved. Let’s define
Since all of its components are positive,
3.2 Endogenous natural gas prices
So far we have analysed energy security and climate stability implications of exogenous imported natural gas rices in cooperative and competitive markets. Now, we allow for the natural gas price to be determined endogenously through Eq. 16 and Eq. 23 for any given number of importing entities (N). Proposition below asserts that in the first stage, the natural gas exporter foreseeing the market conditions in the second stage, always sets the natural gas price higher in the cooperation case compared to the competition case.
Proposition 2. For a given N > 1, the price of natural gas in the full cooperation case is (strictly) higher than the full competition case regardless of the availability of any climate stability measure such as DAC.
Proof. To prove this proposition, we compare the natural gas price in both cases using Eq. 16 and Eq. 23. If we can show that for a given N, the difference between the two prices is strictly positive, the theorem is proved. Let’s define Δg = ηg−coop − ηg−comp. We want to show that Δg > 0 for all N:
Since all the components are positive, Δg is positive. In the special case when θr = 0 (i.e., DAC is ineffective or more generally, in the no-DAC case) the difference will be simplified to:
which again is positive and therefore, the theorem holds regardless of the availability of any climate stability measure such as DAC.The comparison between the optimal share of domestic coal in the cooperative case and competitive case is less straightforward since it both depends on the number of importers and the price of natural gas as shown in equation below.
We know from Eq. 25 that
4 Numerical example
Figure 2 shows the result of a hypothetical model with the following parameter values: Ei = 150, Ee = 80, ηc = 0.25, ηr = 0.001, ηe = 0.4, θc = 0.003, θg = 0.00012, θr = 0.001, δi = 400, δe = 75, and Tx = 0.15.
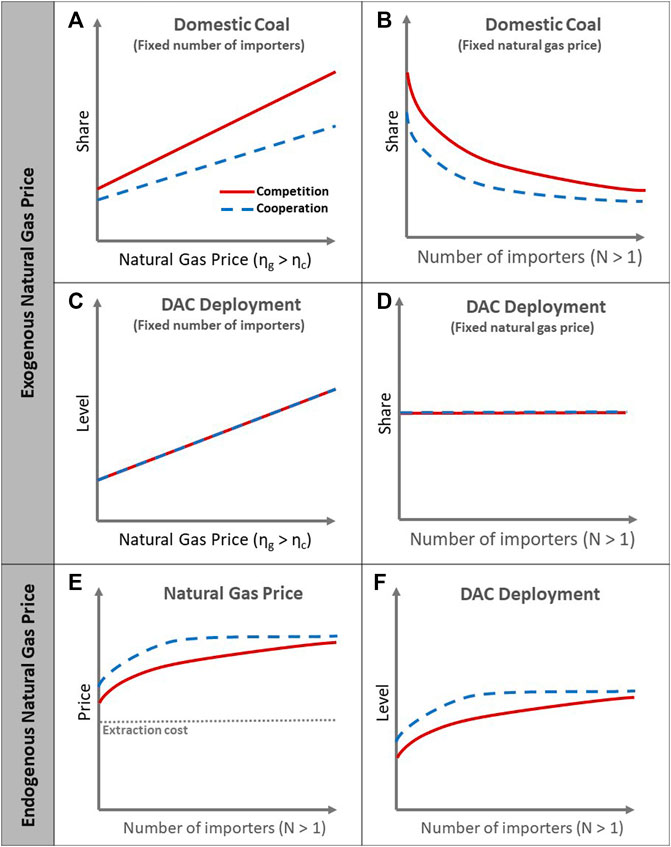
FIGURE 2. Natural gas prices impact in two cases of cooperation and competition with exogenous natural gas prices (A–D) or endogenous natural gas prices (E,F): (A) optimal share of domestic coal with a fixed number of importers, (B) optimal share of domestic coal with a fixed natural gas price, (C) optimal level of DAC deployment with a fixed number of importers, (D) optimal level of DAC deployment with a fixed natural gas price, (E) natural gas price with varying number of importing entities, and (F) DAC deployment levels with varying number of importing entities.
The first 2 rows of this figure correspond to the case with exogenous gas price while the last row is related to the results of the case with endogenous gas price. In Figures 2A,C we assume that the number of importing countries is fixed at N = 10. In Figures 2B,D we assume that the natural gas price is fixed at ηg = 0.76.
Using this set of numerical values, we are able to make a quantitative comparison between the two cases of full cooperation and competition.
4.1 Exogenous natural gas prices
Keeping the number of importing countries constant at N = 10, in the cooperation case, from Eqs 14, 15 we get.
Similarly, in the competition case, from Eqs 21, 22 we get.
As price increases, there is more incentive for importing entities to rely on their domestic energy production in both cases. However, as stated in Proposition 1, such reliance on domestic production is always higher in the competition case compared to the cooperation case. Therefore, the red continuous line corresponding to optimal import share in the competition case cooperation stays above the blue dashed line (Figure 2A).
Alternatively, keeping the natural gas price constant at ηg = 0.76, in the cooperation case, from Eqs 14, 15 we get.
Similarly, in the competition case, from Eqs 21, 22 we get.
In this case, the optimal domestic coal shares follow a reciprocal form and converge in the limit case where N → ∞ (i.e., a large number of countries import natural gas from Russia). In this situation, the difference between the two cases will approaches zero and the cooperative share of domestic coal nears the competitive share (m* → 0.36) implying that every country imports the same share of their energy consumption from Russia regardless of the market structure. Therefore, and similar to the previous case, by following Proposition 1 we have the red continuous curve corresponding to optimal import share in the competition case cooperation staying above the blue dashed curve corresponding to optimal import share in the cooperation case (Figure 2B).
Optimal deployment of DAC however, is always the same in both cooperation and competition cases according to Eq. 15 and Eq. 22 and only depends on the price of imported natural gas. Therefore, when the natural gas price increases the optimal DAC deployment level increases (Figure 2C) while it stays constant when the natural gas price is fixed (Figure 2D). Nevertheless, since the optimal level of DAC deployment is the same in both cases of cooperation and competition, climate change outcomes such as global mean temperature will be worse off under the competitive market conditions due to higher rates of domestic coal consumption.
Finally, as shown in Eq. 24, the difference in the optimal share of domestic coal between the cooperation and competition cases do not depend on the DAC’s effectiveness (θr) or cost (ηr). Therefore, if a reliable DAC technology is not available (e.g., θr = 0), Proposition 1 still holds and the free-riding phenomenon discussed above, still applies to a limit case without DAC.
4.2 Endogenous natural gas prices
Figure 2E shows the schematic comparison of natural gas prices between the two cases of cooperation and competition by changing the number of importing entities. The natural gas price can be obtained from Eq. 16 in the cooperation case and Eq. 23 in the competition case:
As shown in Figure 2E and according to Proposition 2, the red continuous curve corresponding to optimal import share in the competition case cooperation stays below the blue dashed curve corresponding to optimal import share in the cooperation case. As N increases, the imported natural gas price grows slowly in both cases while the price in cooperative case is always dominating the price in the competitive case. In the limit case where N → ∞ (i.e., a large number of countries import natural gas from Russia), the natural gas price in both cases approaches.
Similarly, for the optimal DAC level, from Eq. 15 and Eq. 22 we get.
The results of this case are shown in Figure 2F similar to the natural gas price, the red continuous curve corresponding to optimal DAC level in the competition case cooperation stays below the blue dashed curve corresponding to optimal import share in the cooperation case. As N increases, the imported natural gas price grows slowly in both cases while the price in cooperative case is always dominating the DAC levels in the competitive case. In the limit case where N → ∞, the DAC level in both cases approaches R* → 251.
This has a significant implications for energy security and climate stability. As the optimal share of domestic coal and the optimal level of DAC deployment both directly depend on the natural gas price, an increase in the price of natural gas induce more reliance on domestic coal for energy security and DAC for climate stability. For any given number of importers, N > 1, since the natural gas price is higher in the cooperative case, the optimal level of DAC deployment will also be higher in the cooperative case compared to the competitive case.
5 Discussion
The topic of energy security cannot and should not be addressed without climate change considerations. In particular, and in the case of the EU, the European Green Deal and the Climate law have already provided legal frameworks for the member states to achieve the collective objective of reaching climate neutrality by 2050 while ensuring their energy security (Claeys et al., 2019). Nevertheless, the recent developments in energy markets due to the invasion of Ukraine by Russia has revealed growing vulnerability of the EU energy market to foreign actions beyond its jurisdiction (Mišík, 2022).
This paper has provided an analytical framework for assessing the implications of cooperation and competition among the members of a union such as the EU, for their energy security and climate stability. Despite many simplifying assumptions in this model, it provides several findings which shed light on some less-discussed mechanisms shaping energy markets and climate change solutions.
First, when the natural gas prices are exogenous meaning that they are determined globally and are not affected by the coordination decisions of importing entities at the local level, the optimal level of climate intervention through carbon removal technologies such as DAC is independent of the market conditions (e.g., cooperation or competition). It only depends on the properties of the removal technology (e.g., cost and effectiveness) and the degree that imported and domestic fossil fuels differ in costs and warming potentials. In other words, while energy security considerations yield an optimal combination of imported natural gas and domestic coal to meet the energy demand in any member state, carbon removal measures such as DAC are deployed to offset the emissions rising from such energy portfolio and provide better climate stability.
Furthermore, cooperation among importing entities result in more reliance on imported natural gas in the case of exogenous natural gas prices. This is due to the fact that cooperation induces a coordinated and aggressive emission cuts which implies lowering the consumption of domestic coal and relying on cleaner but more expensive natural gas. Therefore, while cooperation may undermine energy security, competition threatens climate stability despite the availability of DAC.
Finally, if the natural gas price is sensitive to the market conditions, cooperation among importers sends a strong signal about their climate ambitions to curb emissions by reducing domestic coal consumption. The natural gas exporter in this case, is likely to increase the price. If the price increase is significant enough (i.e., if the price in the cooperation case is at least N times the price in the competition case), then the cooperative importers are forced to reduce their import and rely more on their domestic coal. However, higher natural gas price in the cooperation case will also results in higher DAC deployment to offset the emissions resulting from such energy policy switch.
This paper only considered two extreme cases of full cooperation and full competition among identical entities assuming a simple linear form of energy cost and benefit. In reality, the EU countries have very different social, political, and economic characteristics and the energy cost structures are far from being linear. The level of coordination among the EU member states varies significantly over time and over different issues ranging from cooperation to competition side of the spectrum. Furthermore, significant differences in energy system and climate damage cost among the EU member countries or any other economic or political block of cooperating countries can lead to very different outcomes in terms of optimal level of energy import or CDR deployment. Nevertheless, this paper tried to provide an analytical framework for investigating the issues at the intersection of climate security and climate stability. Future research may extend this analysis by incorporating other players and other energy options as well more sophisticated climate change models. A full assessment of energy policies at the national and the EU levels require deeper understanding of domestic energy needs and ambitions as well as potential climate change damages that affect local communities and can translate into political demand for action in the realm of climate change intervention.
Data availability statement
The original contributions presented in the study are included in the article/Supplementary Materials, further inquiries can be directed to the corresponding author.
Author contributions
SS carried out the research and wrote the manuscript solely.
Funding
This project has received funding from the European Union’s Horizon 2020 research and innovation programme under grant agreements No. 101036458—LOCALISED—Localised decarbonisation pathways for citizens, local administrations and businesses to inform for mitigation and adaptation action.
Acknowledgments
I acknowledge the full LOCALISED project team for their support.
Conflict of interest
The author declares that the research was conducted in the absence of any commercial or financial relationships that could be construed as a potential conflict of interest.
Publisher’s note
All claims expressed in this article are solely those of the authors and do not necessarily represent those of their affiliated organizations, or those of the publisher, the editors and the reviewers. Any product that may be evaluated in this article, or claim that may be made by its manufacturer, is not guaranteed or endorsed by the publisher.
Footnotes
1It is worth noting that there are already a dozen commercial applications of DAC in Europe and North America with pioneering companies such as Climeworks (Switzerland), Global Thermostat (United States), and Carbon Engineering (Canada) among others leading the way (McQueen et al., 2021).
References
Aliprantis, C. D. (1999). On the backward induction method. Econ. Lett. 64, 125–131. doi:10.1016/s0165-1765(99)00068-3
Bednar, J., Obersteiner, M., Baklanov, A., Thomson, M., Wagner, F., Geden, O., et al. (2021). Operationalizing the net-negative carbon economy. Nature 596, 377–383. doi:10.1038/s41586-021-03723-9
Beuttler, C., Charles, L., and Wurzbacher, J. (2019). The role of direct air capture in mitigation of anthropogenic greenhouse gas emissions. Front. Clim. 1, 10. doi:10.3389/fclim.2019.00010
Buck, H. J. (2016). Rapid scale-up of negative emissions technologies: Social barriers and social implications. Clim. Change 139, 155–167. doi:10.1007/s10584-016-1770-6
Buschle, D., and Westphal, K. (2019). A challenge to governance in the eu: Decarbonization and energy security. Eur. Energy & Clim. J. 8, 53–64. doi:10.4337/eecj.2019.03-04.04
Chatterjee, S., and Huang, K.-W. (2020). Unrealistic energy and materials requirement for direct air capture in deep mitigation pathways. Nat. Commun. 11, 3287–3293. doi:10.1038/s41467-020-17203-7
Claeys, G., Tagliapietra, S., and Zachmann, G. (2019). How to make the European Green Deal work (JSTOR) Brussels: Bruegel.
Commission, E., and for Communication, D. G. (2021). European green deal: Delivering on our targets. Publications Office. doi:10.2775/595210
Cox, E., Spence, E., and Pidgeon, N. (2020). Public perceptions of carbon dioxide removal in the United States and the United Kingdom. Nat. Clim. Change 10, 744–749. doi:10.1038/s41558-020-0823-z
Erans, M., Sanz-Pérez, E. S., Hanak, D. P., Clulow, Z., Reiner, D. M., and Mutch, G. A. (2022). Direct air capture: Process technology, techno-economic and socio-political challenges. Energy Environ. Sci. 15, 1360–1405. doi:10.1039/d1ee03523a
Fasihi, M., Efimova, O., and Breyer, C. (2019). Techno-economic assessment of co2 direct air capture plants. J. Clean. Prod. 224, 957–980. doi:10.1016/j.jclepro.2019.03.086
Galán-Martín, Á., Vázquez, D., Cobo, S., Mac Dowell, N., Caballero, J. A., and Guillén-Gosálbez, G. (2021). Delaying carbon dioxide removal in the European Union puts climate targets at risk. Nat. Commun. 12, 6490–6512. doi:10.1038/s41467-021-26680-3
Hanna, R., Abdulla, A., Xu, Y., and Victor, D. G. (2021). Emergency deployment of direct air capture as a response to the climate crisis. Nat. Commun. 12, 368. doi:10.1038/s41467-020-20437-0
IPCC (2022). Climate change 2022: Mitigation of climate change. Contribution of working group III to the sixth assessment report of the intergovernmental panel on climate change. Cambridge, UK and New York, NY, USA: Cambridge University Press. doi:10.1017/9781009157926
Maris, G., and Flouros, F. (2021). The green deal, national energy and climate plans in Europe: Member states’ compliance and strategies. Adm. Sci. 11, 75. doi:10.3390/admsci11030075
McQueen, N., Gomes, K. V., McCormick, C., Blumanthal, K., Pisciotta, M., and Wilcox, J. (2021). A review of direct air capture (dac): Scaling up commercial technologies and innovating for the future. Prog. Energy 3, 032001. doi:10.1088/2516-1083/abf1ce
Mišík, M. (2022). The eu needs to improve its external energy security. Energy Policy 165, 112930. doi:10.1016/j.enpol.2022.112930
National Academies of Sciences Engineering and Medicine (2019). Negative emissions technologies and reliable sequestration: A research agenda. National Academies Press.
Nemet, G. F., Callaghan, M. W., Creutzig, F., Fuss, S., Hartmann, J., Hilaire, J., et al. (2018). Negative emissions—Part 3: Innovation and upscaling. Environ. Res. Lett. 13, 063003. doi:10.1088/1748-9326/aabff4
Nordhaus, W. D. (2017). Revisiting the social cost of carbon. Proc. Natl. Acad. Sci. 114, 1518–1523. doi:10.1073/pnas.1609244114
Osička, J., and Černoch, F. (2022). European energy politics after Ukraine: The road ahead. Energy Res. Soc. Sci. 91, 102757. doi:10.1016/j.erss.2022.102757
Pérez, M. d. l. E. M., Scholten, D., and Stegen, K. S. (2019). The multi-speed energy transition in Europe: Opportunities and challenges for eu energy security. Energy Strategy Rev. 26, 100415. doi:10.1016/j.esr.2019.100415
Richard, S. (1995). The damage costs of climate change toward more comprehensive calculations. Environ. Resour. Econ. 5, 353–374. doi:10.1007/bf00691574
Shayegh, S., Bosetti, V., and Tavoni, M. (2021). Future prospects of direct air capture technologies: Insights from an expert elicitation survey. Front. Clim. 46. doi:10.3389/fclim.2021.630893
Smith, P., Davis, S. J., Creutzig, F., Fuss, S., Minx, J., Gabrielle, B., et al. (2016). Biophysical and economic limits to negative co2 emissions. Nat. Clim. change 6, 42–50. doi:10.1038/nclimate2870
Sovacool, B. K., Baum, C. M., and Low, S. (2023). Reviewing the sociotechnical dynamics of carbon removal. Joule 7, 57–82. doi:10.1016/j.joule.2022.11.008
Urmee, T., and Md, A. (2016). Social, cultural and political dimensions of off-grid renewable energy programs in developing countries. Renew. Energy 93, 159–167. doi:10.1016/j.renene.2016.02.040
Keywords: energy policy, direct air capture, climate change, energy security, climate stability, negative emission technologies
Citation: Shayegh S (2023) The prospect of direct air capture for energy security and climate stability. Front. Chem. Eng. 5:1140953. doi: 10.3389/fceng.2023.1140953
Received: 09 January 2023; Accepted: 30 March 2023;
Published: 11 April 2023.
Edited by:
Bita Bayatsarmadi, Commonwealth Scientific and Industrial Research Organisation (CSIRO), AustraliaReviewed by:
Jaewon Byun, Chonnam National University, Republic of KoreaCopyright © 2023 Shayegh. This is an open-access article distributed under the terms of the Creative Commons Attribution License (CC BY). The use, distribution or reproduction in other forums is permitted, provided the original author(s) and the copyright owner(s) are credited and that the original publication in this journal is cited, in accordance with accepted academic practice. No use, distribution or reproduction is permitted which does not comply with these terms.
*Correspondence: Soheil Shayegh, c29oZWlsLnNoYXllZ2hAZWllZS5vcmc=