- 1Department of Chemical and Biological Engineering, University of Wisconsin–Madison, Madison, WI, United States
- 2Department of Neurological Surgery, University of Wisconsin–Madison, Madison, WI, United States
The blood-brain barrier (BBB) is a highly impermeable barrier separating circulating blood and brain tissue. A functional BBB is critical for brain health, and BBB dysfunction has been linked to the pathophysiology of diseases such as stroke and Alzheimer’s disease. A variety of models have been developed to study the formation and maintenance of the BBB, ranging from in vivo animal models to in vitro models consisting of primary cells or cells differentiated from human pluripotent stem cells (hPSCs). These models must consider the composition and source of the cellular components of the neurovascular unit (NVU), including brain microvascular endothelial cells (BMECs), brain pericytes, astrocytes, and neurons, and how these cell types interact. In addition, the non-cellular components of the BBB microenvironment, such as the brain vascular basement membrane (BM) that is in direct contact with the NVU, also play key roles in BBB function. Here, we review how extracellular matrix (ECM) proteins in the brain vascular BM affect the BBB, with a particular focus on studies using hPSC-derived in vitro BBB models, and discuss how future studies are needed to advance our understanding of how the ECM affects BBB models to improve model performance and expand our knowledge on the formation and maintenance of the BBB.
1 Introduction
The neuronal network in the central nervous system (CNS) is highly complex, requiring a specific microenvironmental composition to function properly (Abbott, 1992; Shao et al., 2021). Brain capillaries, which account for ∼85% of the ∼644 km cerebral vessels (Zlokovic, 2008; Sweeney et al., 2018), not only supply nutrients and oxygen and remove wastes to support the metabolic demands of the CNS, but also form a highly impermeable, regulated barrier that restricts the entry of most molecules in the bloodstream and actively transports specific classes of molecules in a polarized manner. This barrier is referred to as the blood-brain barrier (BBB) and is essential to a healthy brain, with dysfunction linked to prevalent and debilitating diseases such as Alzheimer’s disease (AD), multiple sclerosis (MS), stroke, and traumatic brain injury (Cirrito, 2005; Weiss et al., 2009; Shlosberg et al., 2010). Anatomically, the BBB consists of brain microvascular endothelial cells (BMECs) surrounded by brain pericytes embedded in a shared basement membrane (BM), and encased by astrocytic endfeet (Figure 1) (Abbott et al., 2006; Tornabene and Brodin, 2016). The BM is mainly composed of extracellular matrix (ECM) proteins that are secreted and deposited by BMECs, pericytes, and astrocytes.
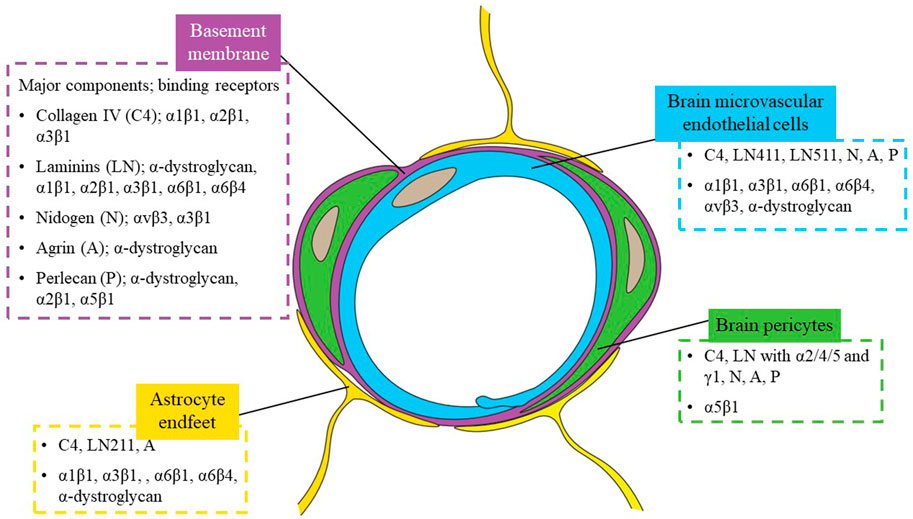
FIGURE 1. Schematic of a brain capillary cross-section. The blood-brain barrier (BBB) anatomically consists of brain microvascular endothelial cells (BMECs), surrounded by brain pericytes embedded in a shared basement membrane (BM), and astrocyte endfeet. The major extracellular matrix (ECM) proteins in the BM, collagen type IV (C4), laminin (LN), nidogen (N), agrin (A) and perlecan (P), are listed with examples of corresponding receptors in the figure. ECM proteins and example receptors expressed by each cell type are in respective colored boxes.
Compared to microvascular endothelial cells (ECs) outside of the CNS, BMECs have no fenestra, few intracellular vesicles, and high expression of tight junction (TJ) and TJ-related proteins such as Occludin, Claudin-5, and ZO-1. These differences result in low rates of endocytosis and transcytosis, and continuous tight junctions that restrict paracellular permeability, respectively. BMECs also differentiate themselves from non-brain ECs by the expression of nutrient transporters and polarized efflux transporters such as glucose transporter (Glut-1), and P-glycoprotein (P-gp), respectively. The quality of the barrier formed by BMECs is typically measured by its low permeability to molecular tracers such as fluorescent dyes and other small molecules, its high transendothelial electrical resistance (TEER), and by the directional transport of specific transporter substrates.
The cellular neighbors of BMECs within the neurovascular unit (NVU), brain pericytes and astrocytes, also are crucial to a functional BBB. As early as the 1970s, Bradbury et al. suggested a special relationship between brain capillaries and astrocytes (Bradbury, 1979), soon after which in vivo and in vitro studies confirmed the importance of endothelial-astrocyte interactions for barrier properties in BMECs (Debault and Cancilla, 1980; Janzer and Raff, 1987). Building upon this knowledge, a standardized, reliable in vitro co-culture protocol was described in 1990 using primary rat astrocytes for improved barrier function in bovine BMECs (Dehouck et al., 1990). More recent studies described how pericytes stabilize capillary structures and upregulate barrier function in endothelial cells (Ramsauer et al., 2002; Hori et al., 2004; Dohgu et al., 2005). In 2010, two independent studies demonstrated how brain pericytes are required for both development of the embryonic BBB and maintenance of the adult BBB (Armulik et al., 2010; Daneman et al., 2010). More specifically, these studies demonstrated that BBB permeability was inversely correlated to brain pericyte coverage in developing mouse brains (Daneman et al., 2010) and that pericyte deficiency increased BBB permeability in adult mice (Armulik et al., 2010).
While these studies have provided insight into the role of the cellular components of the NVU in regulating the BBB, we have less of an understanding of how the BM regulates BBB formation and maintenance. Here, we first introduce the major components of the vascular BM and review studies demonstrating the importance of each component as it relates to the in vivo BBB. We then discuss the impact of ECM components on in vitro BBB models, focusing on models comprised of hPSC-derived cells, and briefly discuss how cell type-specific ECM could be important in BBB models. Finally, we describe how hPSC-derived models can be used to explore the effects of brain BM on the BBB to improve these models, to advance our understanding of the BBB in development and disease, and to develop improved strategies to target therapies across the BBB.
2 Major ECM components and dynamics of the BM
Biochemically, the vascular BM has been profiled on the protein level, primarily by targeted in situ immunostaining and western blotting (Barber and Lieth, 1997; Paulsson, 1992; Pöschl et al., 2004; Sixt et al., 2001; Song et al., 2017; Sorokin et al., 1994). These experiments have demonstrated that the primary constituents of the BM can be classified into four major types of ECM proteins: collagen IV, laminin, nidogen (also called entactin), and heparan sulfate proteoglycans (HSPGs), which include perlecan and agrin.
2.1 Collagen
Collagens are proteins with a characteristic triple helix of three α-chains. There are more than 20 types of collagens. Type I collagen, the most abundant and well-studied collagen, is found in bones, tendons, skin, ligaments, cornea and many interstitial connective tissues (Gelse, 2003). Type IV collagen is mainly found in basement membranes, and six different collagen IV α-chains have been identified. Collagen IV, with mainly α1 and α2 chains (encoded by COL4A1 and COL4A2), is synthesized by BMECs, pericytes, and astrocytes (Webersinke et al., 1992; Tilling et al., 2002; Stratman et al., 2009; Vanlandewijck et al., 2018). Collagen IV can bind to α1β1 and α3β1 integrins which are expressed by BMECs and astrocytes (Baeten and Akassoglou, 2011).
Collagen IV is a major structural component of vascular BMs throughout the body. Global knockout of COL4A1/2 results in lethality as early as embryonic day 10.5 (E10.5) in mouse models (Pöschl et al., 2004). COL4A1 mutations are also correlated with cerebrovascular and neurological diseases such as ischemic stroke, intracerebral hemorrhage (ICH), and porencephaly in human families (Gould et al., 2006, 2005). Conditional deletion of COL4A1 in mouse BMECs or pericytes caused fully penetrant ICH, incompletely penetrant porencephaly, and macro-angiopathy, demonstrating a central role of collagen IV in vascular defects and brain damage (Jeanne et al., 2015).
2.2 Laminin
Laminins are trimeric proteins composed of α, β, γ chains. Five different α, four β, and three γ chains have been identified, and laminin isoforms are denoted by their chain composition (e.g., laminin 111, or LN111, for the heterotrimer consisting of α1, β1, γ1 chains) (Aumailley, 2013). BMECs mainly synthesize LN411 and LN511 (Sorokin et al., 1997; Sixt et al., 2001), and pericytes also secrete α2/4/5- and γ1-containing laminins (Stratman et al., 2009; Gautam et al., 2016; Vanlandewijck et al., 2018). Astrocytes primarily generate LN211 (Jucker et al., 1996; Sixt et al., 2001). Laminins can bind to α-dystroglycan and integrins such as α1β1, α2β1, α3β1, α6β1, α6β4, to activate a variety of signaling pathways that regulate cell proliferation, differentiation and migration (Belkin and Stepp, 2000; Baeten and Akassoglou, 2011; Arimori et al., 2021). For example, endothelial cells exhibit growth arrest when their α2β1 integrins bind to laminins and activate cyclin-dependent kinases (CDKs) CDK4 and CDK6 (Mettouchi et al., 2001).
Like collagen IV, laminin is a major structural component of vascular BM, and most global knockouts of its subunits (e.g., α5, β1, γ1) are embryonically lethal. Recently, viable genetic mouse models revealed that laminin contributes to BBB integrity. In 2014, Yao et al. (2014) showed that conditional deletion of laminin γ1 in mouse neural progenitor cells (and thus astrocytes differentiated from these cells) resulted in BBB breakdown and spontaneous ICH. Menezes et al. (2014) showed that global knockout of laminin α2 in mice resulted in defective BBB with increased permeability to Evans blue. In 2019, Gautam et al. (2019) showed that conditional deletion of laminin α5 in mouse endothelial cells had little effect under homeostatic conditions, but resulted in elevated BBB permeability after ICH was induced by intracerebral injection of collagenase. In 2020, Gautam et al. (2020) reported that conditional knockout of pericyte-derived laminins in mice resulted in mild BBB breakdown during aging. In short, laminins are important to the BBB, especially for maintaining BBB integrity.
2.3 Nidogen, perlecan, agrin
Nidogen has two isoforms, nidogen-1 and nidogen-2. Neither self-polymerizes, but both can crosslink collagen IV and laminin (Fox et al., 1991). Similarly, perlecan (HSPG2) does not self-assemble into sheet-like structures but can interact with other BM components and heparin-binding growth factors (Farach-Carson and Carson, 2007). Both nidogens and perlecan are synthesized by BMECs and pericytes (Vanlandewijck et al., 2018). Agrin can self-aggregate or interact with laminin (Bezakova and Ruegg, 2003), and is produced by BMECs, pericytes, and astrocytes (Vanlandewijck et al., 2018). It was found that nidogen can bind to αvβ3 and α3β1 integrins, perlecan to α-dystroglycan, α2β1 and α5β1 integrins, and agrin to α-dystroglycan (Baeten and Akassoglou, 2011; Nakamura et al., 2019).
Global knockout of both nidogen isoforms in mice is perinatally lethal (Bader et al., 2005), although mice lacking nidogen-2 showed no overt abnormalities (Schymeinsky et al., 2002). In mice with global knockout of nidogen-1, the thickness of brain capillary BM was significantly reduced compared to the wild type. In some cases, the brain capillary BM was completely absent and the BMECs appeared swollen in the cerebral cortex, which suggests possible functional defects of the CNS (Dong et al., 2002). However, in a later study, the authors reported no significant defects or abnormalities in the CNS of nidogen-1-null mice and concluded that there was no significant difference between mutant mice and wild type mice in terms of the exclusion of Evans blue by the BBB (Vasudevan et al., 2009).
Global knockout of perlecan leads to embryonic lethality in mice (Arikawa-Hirasawa et al., 1999; Costell et al., 1999). In 2019, Nakamura et al. (2019) reported that even though perlecan deficiency does not appear to affect the BBB under normal conditions, more BBB leakage and larger infarct volumes were detected in conditional perlecan-deficient mice after transient middle cerebral artery occlusion. Moreover, primary human BMECs and brain pericytes attached to substrates coated with either full-length or the C-terminal domain V of perlecan (perlecan DV) in vitro, and the use of perlecan DV coating promoted PDGF-BB-induced pericyte migration in an in vitro wound healing assay.
Global knockout of agrin is also embryonically lethal in mice (Gautam et al., 1996). Conditional knockout of agrin in mouse endothelial cells resulted in increased brain vascular accumulation of β-amyloid (Aβ), which is linked to AD pathology (Rauch et al., 2011). During chick and rat development, agrin was detected in association with brain microvessels around the time when the BBB was formed (Barber and Lieth, 1997). Taken together, nidogen and agrin have been linked to BBB formation and function, although their mechanisms of action are unclear, while perlecan has been suggested to support BBB maintenance and repair via pericyte recruitment following ischemic stroke.
2.4 Other ECM components of the vascular BM
Unlike capillaries and postcapillary venules, arterioles and venules are not surrounded by pericytes embedded in endothelial BM, but instead vascular ECs are surrounded by endothelial BM, interstitial matrix, then smooth muscle cells and their BM, in that order. The interstitial matrix consists mainly of collagen I and collagen III, together with other minor components such as decorin, biglycan, fibronectin, and vitronectin (Thyberg et al., 1990; Dufourcq et al., 1998; Yousif et al., 2013). However, it is noteworthy that most of our understanding of the composition of the vascular BM surrounding arterioles and venules is based on studies of vessels from organs other than the brain. Profiling the composition of the BM in larger vessels in the brain will be important to identify similarities and differences to the BM in other vessels in the body.
2.5 Developmental dynamics of the BM
The composition of the vascular BM during development is dynamic, guiding formation and maturation of vessels. For instance, based on in situ hybridization, capillary ECs did not express LAMA5 in embryonic and newborn mouse brains, but LAMA5 was found in mouse brain capillary ECs ∼4 weeks after birth (Sorokin et al., 1997). LAMA4 expression on the other hand, was detected by in situ hybridization in mouse brain capillary ECs as early as embryonic day 13 (Frieser et al., 1997). Thus, laminin composition appears to be developmentally dynamic in brain with LAMA4 expressed throughout development but LAMA5 induced in mature capillaries. Other than laminin, the details of the developmental dynamics of brain-specific vascular BM and their role in regulating BBB induction remain largely unclear.
2.6 Changes in BM in neurological degenerative diseases
AD is the most common form of dementia, and one of the pathological hallmarks of AD is cerebral amyloid angiopathy (CAA), the abnormal accumulation of Aβ in cerebral blood vessel walls. Recent hypotheses propose a combination of genetic factors and vascular factors, such as BBB dysfunction (Nelson et al., 2016), in AD pathogenesis. For instance, BMECs and pericytes are found to be impaired in terms of their Aβ-clearing abilities in the early stage of AD (Gorelick et al., 2011; Montagne et al., 2018). At the same time, various studies using mouse models and post-mortem human tissues have demonstrated BM thickening in AD brains (Mancardi et al., 1980; Claudio, 1995; ZAROW et al., 1997; Bourasset et al., 2009; Gama Sosa et al., 2010; Merlini et al., 2011; Lepelletier et al., 2017), thus it is hypothesized that BM thickening may play a role in compromising overall Aβ clearance and exacerbating Aβ accumulation. However, variable results have been reported regarding the changes in specific BM components enriched or depleted using transgenic AD model mice and/or post-mortem human tissues. For instance, Hawkes et al. (2013), Hawkes et al. (2012) reported increased collagen IV in the BM of mouse models of AD, while Bourasset et al. (2009) and Mehta et al. (2013) reported decreased collagen IV.
The second most common neurodegenerative disease, Parkinson’s disease (PD), is also linked to BBB dysfunction (Li et al., 2014; Booth et al., 2017) and to thickening of brain capillary BM (Farkas et al., 2000; Bertrand et al., 2008). The number of string vessels (collapsed BM without endothelium) was found to be significantly increased in PD patient samples (Yang et al., 2015). In addition to AD and PD, BM changes coincided with BBB dysfunction or breakdown in amyotrophic lateral sclerosis (ALS) (Garbuzova-Davis et al., 2007; Coatti et al., 2017; Yoshikawa et al., 2022). However, similar to AD, it is unclear how specific BM components change as a result of ALS. For instance, while Wiksten et al. (2007) reported increased laminin in the BM of ALS patients, Liu et al. (2011) reported decreased laminin.
Unlike AD and PD which were associated with BM thickening, degradation or dissolution of the BM was found following stroke in mice, rats and baboons (Hamann et al., 1995; Fukuda et al., 2004; Hamann et al., 2004; Kwon et al., 2009; Katsu et al., 2010). Most stroke studies reported that collagen IV, laminin, perlecan and agrin were degraded in both animal models and human postmortem tissues (Hamann et al., 1995; Horstmann et al., 2003; Vosko et al., 2003; Fukuda et al., 2004; Solé et al., 2004; Gu et al., 2005; McColl et al., 2008; Rosell et al., 2008; Baumann et al., 2009; Lee et al., 2011). However, some studies reported increased abundance of collagen IV and laminins following ischemic stroke in rodents (Anik et al., 2011; Ji and Tsirka, 2012). In addition to AD, PD and stroke, MS was also found to be associated with changes in NVU BM structure and composition. Specifically, BM in MS lesions was irregular and discontinuous, and BM components including laminins and HSPGs were abnormally deposited into the ECM of the CNS white matter in MS patients (van Horssen et al., 2006, 2005). Future studies may be able to address how BM composition changes during disease and uncover mechanisms by which ECM components affect neurodegenerative disease pathologies using disease models built with patient-derived induced pluripotent stem cells (iPSCs) or genetically modified hPSCs, in conjunction with animal studies and human postmortem samples.
3 Use of BM ECM components in modeling the BBB in vitro
3.1 Collagen IV
3.1.1 Primary culture models
In vitro studies often use collagen-coated surfaces to culture primary BMECs or purify for primary BMECs via selective adhesion. In 1986, one of the first established protocols for in vitro culturing of primary animal BMECs used a rat tail collagen coating which mainly consists of collagen I (Freshney, 1986; Abbott et al., 1992). In 1998, Tilling et al. (1998) found that Transwell inserts coated with collagen IV, laminin, fibronectin, or 1:1 (mass ratio) mixtures of any two of these proteins all significantly increased TEER across the barrier formed by primary porcine BMECs, compared to the rat tail collagen coating. Since then, many studies adopted collagen IV or collagen IV + fibronectin (C4/Fn) coatings to culture primary BMECs. For example, in 1999, Igarashi et al. (1999) cultured primary porcine BMECs on collagen IV-coated plates to study how glial cell-derived neurotropic factor affects barrier function. Perrière et al. (2005) cultured primary rat BMECs on collagen IV-coated Petri dishes in 2005, and Calabria et al. (2006) cultured primary rat BMECs on C4/Fn-coated surfaces including Transwell inserts in 2006 to study how puromycin can be used to purify primary BMECs in in vitro cultures. Other studies followed a protocol published in 2013 (Navone et al., 2013) where primary human and mouse BMECs were purified by selective adhesion to collagen I-coated flasks and cultured on collagen I-coated surfaces, such as Transwell inserts (Chan et al., 2018; Fan et al., 2019; Zhong et al., 2020).
3.1.2 Stem cell-based BBB models
In the last decade, an increasing number of studies have used hPSC-derived cells to build human in vitro BBB models for their human origin, high scalability, and physiological barrier properties. It was first reported in 2012 that cells possessing some key functional characteristics of BMECs can be derived from hPSCs. These characteristics include expression of tight junction proteins, nutrient transporters and polarized efflux transporters, the ability to form a barrier with high, physiological TEER and low passive permeability comparable to that of primary animal BMECs, and responsiveness to astrocyte- and pericyte-derived cues (Lippmann et al., 2012; Canfield et al., 2019; Di Marco et al., 2020). These hPSC-derived BMEC-like cells (hPSC-BMECs for short) were generated with the following steps (Figure 2A): hPSCs were seeded and expanded in hPSC culture medium on plates coated with Matrigel, a complex ECM protein mixture (largely laminin, collagen IV and nidogen) derived from Engelbreth-Holm-Swarm mouse sarcomas (Hughes et al., 2010). The medium was first switched to unconditioned medium which drives simultaneous differentiation of neural cells and BMEC-like cells, simulating the microenvironment of the developing brain. The medium was then switched to one that selectively expands endothelial cells, and the mixed population was re-plated onto C4/Fn-coated surfaces to purify the endothelial cells.
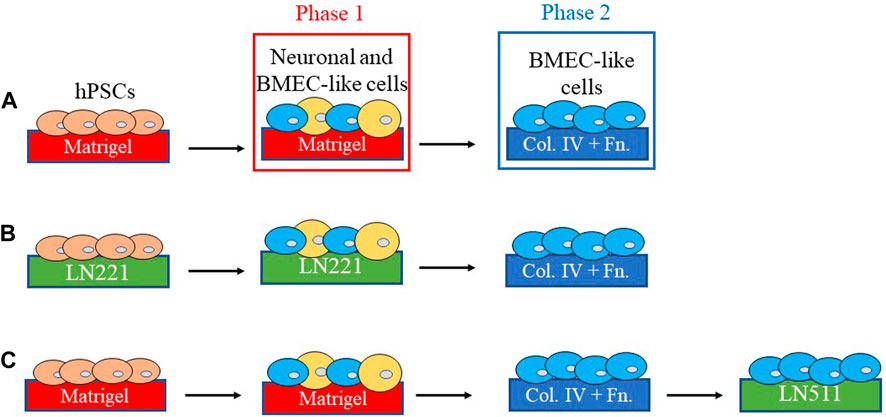
FIGURE 2. Schematics of differentiation and characterization of hPSC-BMEC like cells. (A) BMEC-like cells can be derived from human pluripotent stem cells (hPSCs) by first co-differentiating hPSCs to neural cells and BMEC-like cells on plates coated with Matrigel, then purifying and maintaining endothelial cells on endothelial-selective collagen IV/fibronectin (C4/Fn)-coated surfaces such as Transwell inserts for transendothelial electrical resistance (TEER) measurement(Lippmann et al., 2012). (B–C) Schematics of experimental setups for studies on how laminin isoforms affect hPSC-BBB models. It was found that laminin isoforms 221 and 511 can replace or supplement Matrigel in hPSC-BMEC differentiation phase 1(Aoki et al., 2020) and C4/Fn in phase 2(Motallebnejad and Azarin, 2020), respectively, for improved hPSC-BMEC characteristics such as higher TEER and lower permeability.
Since the initial success of generating hPSC-BMECs, numerous modifications have been made to the differentiation protocol, but the vast majority of the published protocols use the same ECM coatings: Matrigel for the 1st phase of the differentiation, and C4/Fn for the 2nd phase (Figure 2A) (Lippmann et al., 2014, 2012; Hollmann et al., 2017; Qian et al., 2017; Neal et al., 2019). The authors of the first hPSC-BMEC publication chose C4/Fn coating because it is “commonly used for primary BMEC culture” (Lippmann et al., 2012). However, the C4/Fn coating may not optimally support all BMEC phenotypes. For example, Nakakura et al. (2021) reported in 2021 that fibronectin can maintain functional fenestra in rat ECs from fenestrated capillaries (i.e., leaky capillaries, as opposed to highly impermeable, barrier-possessing capillaries in the brain), which suggests that the use of fibronectin may not be the best choice for BBB models. However, at the same time, recent in silico analyses of RNA sequencing results indicate that human brain pericytes express FN1 (the gene encoding fibronectin) at a higher level than mouse brain pericytes (Gastfriend et al., 2021a), thus future studies are needed to uncover species-specific effects of fibronectin on the BBB formation and/or maintenance.
C4 is not only used in ECM coatings to aid in purifying endothelial-like cells from a mixed cell population during the 1st phase of hPSC-BMEC differentiation, but also supports the continued culture of differentiated hPSC-BMECs during the 2nd phase. It was reported that hPSC-BMECs can also be purified and cultured until the formation of confluent monolayers on collagen I-coated glass surfaces for simultaneous imaging analyses and permeability assays (Ruano-Salguero and Lee, 2018). The final cell products expressed Occludin, Claudin-5, Glut-1 and Mfsd2a. Permeabilities of sodium fluorescein (NaFL) and IgG measured through confocal microscopy of hPSC-BMECs cultured on collagen I-coated glass surfaces were comparable to previously reported values from hPSC-BMECs cultured on C4/Fn-coated Transwell inserts (Ruano-Salguero and Lee, 2018). It is possible that this system may be further improved in terms of physiological relevance if ECM components found in the brain vascular BM are used in conjunction with collagen I gels, for example, if the collagen I gel was coated with collagen IV and/or laminin.
In contrast to 2D studies where hPSC-BMECs are cultured on C4/Fn-coated plastic surfaces or porous polymer membranes, recent studies aiming to construct 3D brain microvessels often use collagen I gels as the scaffolds, adjust and optimize gel stiffness, then coat the gel with physiologically relevant BM components. In pilot 2D experiments preparing for 3D microvessel construction, Katt et al. (2018) noticed that gels with a higher collagen I concentration, thus higher stiffness, led to better cell coverage after seeding hPSC-BMECs. Katt et al. (2018) also found that the collagen I gel fabricated with or without LN/nidogen before coating the gel with C4/Fn had no effects on cell coverage. Eventually, Katt et al. (2018) chose to construct 3D microvessels by seeding hPSC-BMECs into cylindrical collagen I gels coated with C4/Fn (Figures 3A, B). The resulting microvessels expressed ZO-1 and exhibited much lower permeability to Lucifer yellow (LY) than in previous studies using microvessels formed by human umbilical vein endothelial cells (HUVECs). In 2019, two additional studies used the cross-linker genipin to adjust the stiffness of a collagen I gel used to generate the cylindrical structure. Grifno et al. (2019) coated the 3D structure with Matrigel before seeding hPSC-BMECs, and the resulting microvessels expressed Claudin-5 and exhibited low permeability to LY. Linville et al. (2019) coated the collagen I gel with C4/Fn before seeding hPSC-BMECs. The resulting hPSC-BMEC vessels expressed BBB markers such as Claudin-5 and Glut-1, and exhibited low permeability to LY, Rhodamine 123 (R123), and 10 kD dextran compared to vessels formed with HUVECs. These two studies reported consistent results of low permeability across hPSC-BMECs cultured in 3D vessels built with collagen I gels coated with Matrigel or C4/Fn (Figures 3A, B).
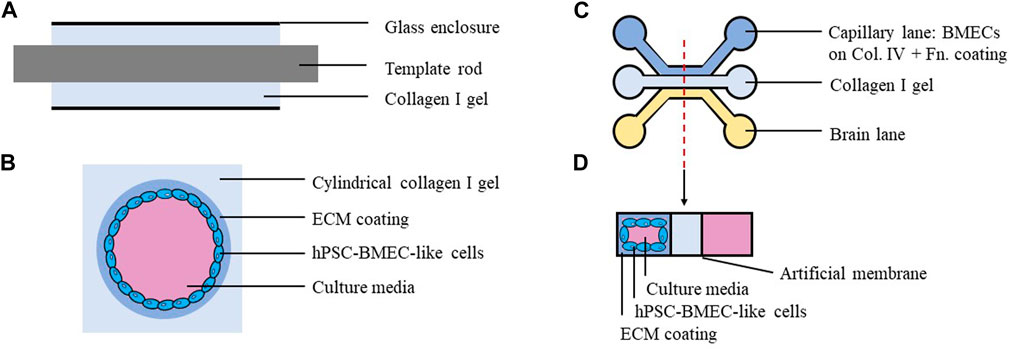
FIGURE 3. Schematics of three-dimensional models built with hPSC-BMEC like cells. (A) Side view of the collagen I gel during model construction. Neutralized collagen I was injected into glass enclosure around a template rod. (B) Cross-sectional view of finished model. The cylindrical gel was treated with ECM coating solutions, then seeded with hPSC-derived BMEC-like cells. (C) One well in the OrganoPlate 3-lane plate (with 40 clusters of 9 wells on each plate). (D) Cross-sectional view of the finished model. Three lanes are separated by artificial membranes. Capillary lane was coated with collagen IV and fibronectin, then seeded with hPSC-derived BMEC-like cells. The middle lane was filled with collagen I gel.
Recent studies have also demonstrated effectiveness of C4/Fn coatings on other substrates and scaffolds in hPSC-BMEC models. For instance, in a study published in 2022, electrospun fiber mats from gelatin and/or poly-ε-caprolactone (PCL) solutions were synthesized, and then the fiber mats were coated with C4/Fn before seeding hPSC-BMECs. This study showed that NaFL permeability was significantly lower for hPSC-BMECs cultured on PCL fiber mats coated with C4/Fn compared to those cultured on Matrigel-coated Transwell inserts (Rohde et al., 2022). Another study published in 2022 used the capillary lane of a MIMETAS OrganoPlate® 3-lane (a device with three divided lanes, namely, capillary, middle (hydrogel), and brain lanes) coated with C4/Fn (Figures 3C, D). BMECs cultured in this system expressed BBB markers such as Claudin-5, ZO-1, Glut-1 and Breast Cancer Resistance Protein (BCRP) and exhibited low permeability to LY. Notably, the authors found that ABCG2 (gene encoding BCRP) expression in BMECs was 3.3-fold higher in their 3D system compared to 2D monolayer culture measured by qRT-PCR, and that transport of BCRP substrates in their 3D system was comparable to that in previous in vivo rat studies (Kurosawa et al., 2022). It is possible that these systems could be further improved and lead to better in vitro BBB models if coatings alternative to C4/Fn were tested and optimized.
Collagen gels have also been used to embed cells for co-culture experiments to model cellular interactions in the NVU. For example, in 2019, hPSC-derived mesodermal pericytes were embedded in collagen I gels placed onto Transwell inserts, then hPSC-BMECs were cultured on the gel. There were no significant differences in barrier tightness measured by TEER with and without embedded pericytes. However, the authors found that the presence of hPSC-BMECs caused the pericytes to migrate further away from the gel surface compared to pericytes in gels without BMEC coverage, demonstrating that hPSC-pericytes respond to cues derived from hPSC-BMECs (Jamieson et al., 2019). While brain pericytes are indeed embedded in vascular BM in vivo [Figure 1], the use of brain vascular BM components (e.g., collagen IV instead of collagen I) to embed primary human pericytes or hPSC-derived brain pericyte-like cells (Stebbins et al., 2019) may provide an in vitro platform that better models BMEC-pericyte interactions.
3.2 Laminin
Isolating and purifying specific laminin isoforms from tissues has been difficult or impractical, and production protocols for recombinant laminins have a relatively short history (∼10 years) (Miyazaki et al., 2012) compared to laminin-containing ECM mixtures such as Matrigel (30+ years) (Kleinman et al., 1986; Guzelian et al., 1988; Fridman et al., 1990; Taub et al., 1990). This results in considerably higher costs of purified laminin isoforms compared to Matrigel. As discussed in Section 3.1.2, most studies developing and optimizing hPSC-BMEC differentiation protocols focused on the composition of the culture media and parameters such as the seeding density of hPSCs, with little regard to the composition of the ECM coatings (Lu et al., 2021a; Yan et al., 2021). Due to limited access to purified laminins and lack of consideration of the ECM in hPSC-BMEC differentiation, effects of specific laminins in in vitro BBB models were not systematically studied until recently. In the past few years, researchers hypothesized that the use of specific laminins can influence performance of in vitro BBB models given the physiological roles of laminins in the NVU BM. Specifically, Aoki et al. (2020) compared coating 2D cell-culture surfaces with Matrigel, Fn, vitronectin, LN221, LN411, and LN511 during the 1st phase (days 0–8) of hPSC-BMEC differentiation (Figure 2B). All groups were re-plated onto the standard C4/Fn-coated surfaces for the 2nd phase of differentiation. The authors found that hPSC-BMECs differentiated on LN211 and those on vitronectin had significantly higher TEER compared to those differentiated on Matrigel. hPSC-BMECs differentiated on LN221 also exhibited significantly lower permeabilities to FD4 and LY and significantly higher P-gp and BCRP activities, measured by accumulation of their substrates R123 and Hoechst, respectively. The authors speculated that LN211 coating might be optimal for the 1st phase of hPSC-BMEC differentiation because the laminin α2 subunit plays a role in BBB formation, as demonstrated by previous in vivo study conducted by Menezes et al. (2014).
In another study published in 2020, Motallebnejad et al. hypothesized that the use of laminin coating might be beneficial for later phases of hPSC-BMEC differentiation, since there exists evidence for a switch from fibronectin-mediated signaling during development to laminin-mediated signaling during maturation in mouse CNS (Milner, 2002). The authors compared LN511, LN411, and C4/Fn coatings following a shortened (1–2 h incubation instead of up to several days) 2nd phase of hPSC-BMEC differentiation (Figure 2C), while keeping the preceding differentiation substrates unchanged from standard protocols (i.e., Matrigel for the 1st phase and C4/Fn for the selective purification). The authors found that LN511, but not LN411, improved expression and localization of Occludin, Claudin-5, ZO-1, and VE-cadherin. The use of LN511 resulted in a more activated phenotype in hPSC-BMECs (e.g., significantly lower expression of ANGPT2, MMP1, MMP9, FN1 and LAMA5 measured by mRNA abundance, and more prominent migration in wound healing assay). The use of LN511 also enhanced responses of hPSC-BMECs to shear stress under dynamic flow conditions: Analysis of phase contrast images revealed a significant increase in cell elongation measured by aspect ratio of the cells; fluorescent staining for F-actin demonstrated formation of stress fibers which were not present in the static culture, and immunocytochemistry analysis showed increased expression of Claudin-5, ZO-1, and VE-cadherin compared to cells cultured in static conditions (Motallebnejad and Azarin, 2020). Few studies building 3D models of the BBB using hPSC-BMECs have incorporated laminins in the scaffolds. Katt et al. (2018) compared various ECM coatings on collagen I gels and found no significant differences in cell adhesion or coverage between coatings with one, two, or three components among collagen IV, Fn, and laminin.
NVU cell types other than BMECs differentiated on specific laminin isoforms were also found to differ in their BMEC barrier-inducing capacities. For instance, in 2019, hPSC-astroglia differentiated on human LN521 were found to induce barrier properties in hPSC-BMECs (e.g., lower NaFL permeability and higher VE-cadherin expression level) more than those differentiated on murine laminins (Delsing et al., 2019), demonstrating that BM components can also affect the BMEC barrier indirectly through associated NVU cells.
3.3 Nidogen, perlecan, agrin, and other ECM proteins
There has been very little exploration of the roles of nidogen, perlecan, agrin, or other ECM proteins using in vitro BBB models. In 2018, Katt et al. (2018) found that the addition of agrin, but not perlecan, to the C4/Fn coating on collagen I gels significantly increased coverage of hPSC-BMECs. However, the addition of agrin and laminin to C4/Fn coating also significantly decreased TEER of the final hPSC-BMEC monolayers. This effect was speculated by the authors to be agrin/laminin-mediated enhancement of focal adhesion formation to the detriment of barrier function. In 2019, Qian et al. (2017) found that Synthemax or recombinant human vitronectin coating can be used to replace Matrigel for hPSC culture and the 1st phase of hPSC-BMEC differentiation (Figure 2A), and that the resulting cells expressed key proteins such as Claudin-5, Occludin, Glut-1, and P-gp, similar to those differentiated on Matrigel. More systematic and quantitative comparisons are needed to examine the effects of incorporating nidogen/agrin/perlecan and other ECM proteins into in vitro BBB models.
4 Decellularized ECM and the BBB
A complementary strategy to systematic comparison of single or combinations of ECM components is the use of decellularized ECM, which is typically obtained using detergent-containing wash buffers to remove cells from confluent culture, leaving a layer of ECM deposited by those cells on the cell culture surfaces. In 2007, Hartmann et al. (2007) cultured primary porcine BMECs on decellularized ECMs derived from porcine brain pericytes, mouse astrocytes, porcine aorta ECs, and porcine BMECs. Both pericyte-ECM and astrocyte-ECM increased TEER, while aorta-ECM decreased TEER, compared to the BMEC-ECM control. In 2016, Zobel et al. (2016) cultured primary porcine BMECs on decellularized ECMs derived from porcine brain pericytes, spinal cord astrocytes, and porcine BMECs. BMECs cultured on pericyte-derived ECM exhibited significantly higher TEER than those cultured on ECMs derived from astrocytes or BMECs. Zobel et al. (2016) also generated layered decellularized ECMs by sequentially culturing and removing astrocytes followed by pericytes (AP-ECM), or pericytes followed by astrocytes (PA-ECM). Both AP-ECM and PA-ECM were found to induce TEER to a greater extent compared to double layers of BMEC-ECM. These studies suggest that decellularized ECMs derived from NVU cell types could enhance BMEC phenotypes in in vitro BBB models. Compared to using surfaces coated with specific ECM proteins or protein mixtures, decellularized ECM has the advantage of better recapitulation of the complex structure and composition of ECM in vivo, providing appropriate chemical and mechanical cues for cell function from specific cell types (i.e., cell types used for generating decellularized ECMs are cell types from which chemical and mechanical cues are derived). However, the use of decellularized ECM has some disadvantages as well, including being largely uncharacterized, thus not directly providing insights regarding molecular mechanisms, having larger batch-to-batch variability, and source material availability issues that complicate large-scale experimentation.
5 Perspectives and conclusion
The vascular BM is an indispensable part of the BBB. Major BM components in the healthy adult NVU, including collagen IV, laminin, nidogen, perlecan, and agrin, were all found to play roles in BBB development and/or organism viability based on animal studies with targeted deletions. Initial in vitro BBB models were typically comprised of primary BMECs or hPSC-derived BMEC-like cells cultured on surfaces coated with Matrigel, collagen I, collagen IV, fibronectin, and mixtures of these ECM proteins, and the resulting models capture key BBB phenotypes including barrier formation and transporter activities. More recent models have cultured hPSC-BMECs on specific combinations and isoforms of BM components that are informed by neurovascular development, such as laminin 511 following collagen IV-fibronectin mixture. These combinations improved BBB phenotypes, including elevated barrier function and/or enhanced responses to shear stress in hPSC-BMECs, resulting in improved in vitro human BBB models (Table 1). In this review, we focused on how hPSC-BMEC models are affected by different ECM components since the hPSC-BMEC model is currently the only in vitro human BBB model with both a physiologically tight barrier (e.g., measured by TEER greater than one thousand Ω∙cm2) and organotypic transporter activities (Aazmi et al., 2022), but admittedly, current hPSC-BMEC models have significant limitations. For example, it was found that hPSC-BMECs exhibit epithelial transcriptional signatures and low expression of endothelial genes (Lippmann et al., 2020; Workman and Svendsen, 2020; Lu et al., 2021b, 2021a). Introducing endothelial-specific transcription factors ETV2, ERG, and FLI1 to hPSC-BMECs induced expression of endothelial transcripts, but also diminished BBB phenotypes, including barrier properties (Lu et al., 2021b). Generating hPSC-BMECs that both possess endothelial identity and recapitulate BBB phenotypes is a critical, current roadblock to employing hPSC-derived models of the BBB, and identifying appropriate ECM substrates for differentiation and culture of these cells could play an important role in improving the fidelity of hPSC-BMECs.
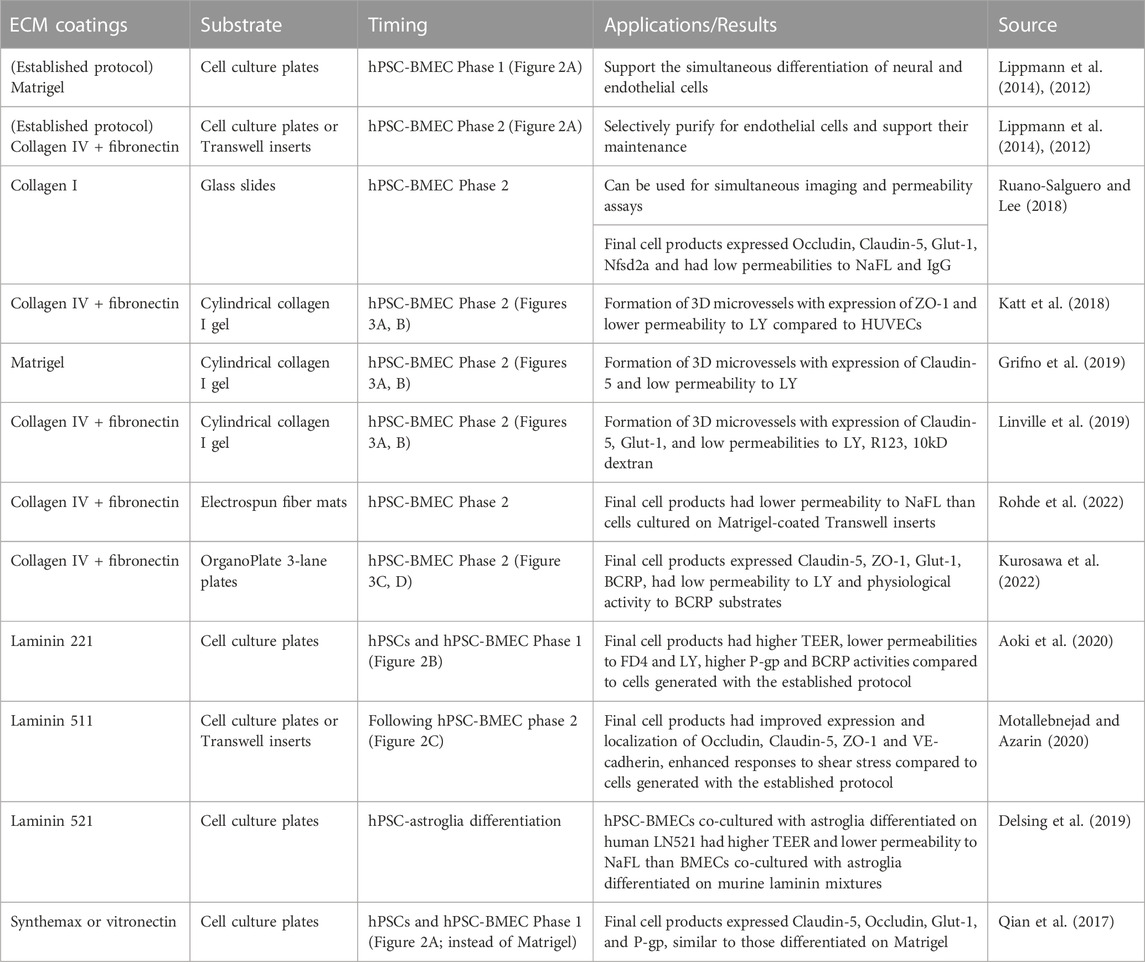
TABLE 1. ECM coatings used in in vitro hPSC-BBB models. Summary of ECM coatings used in recent in vitro hPSC-BBB models, compared to the established protocol described in section 3.1.2 and illustrated in Figure 2A.
As a complementary approach to inducing endothelial gene expression in existing hPSC-BMEC models, efforts are underway to impart BBB phenotypes to naïve or “generic” endothelial cells (ECs) (e.g., Lian et al., 2014; Patsch et al., 2015) by expressing transcription factors (Roudnicky et al., 2020) or treating the cells with small molecules (Gastfriend et al., 2021b). These strategies can only induce a limited subset of BBB properties in generic ECs; for example, the resulting cells do not form physiologically tight barriers and lacked efflux transporter activity. In addition, the role of ECM in stimulating BBB specification in these ECs has not yet been studied and could be a fruitful path forward. While recent reviews of in vitro BBB models have thoroughly summarized how different models compare and can be used to study BBB/NVU function (Erickson et al., 2020; Linville and Searson, 2021; Yan et al., 2021; Aazmi et al., 2022), none has recognized the role of ECM in hPSC-derived BBB models, indicating a continued need for ECM exploration in the BBB modeling field.
Moving forward, a better fundamental understanding of the composition, organization and dynamics of the BBB BM during development, health, and disease is needed to guide in vitro BBB model development. Notably, while NVU-specific transcriptomic data provides some insight into cell type-specific synthesis of ECM components, quantitative proteomics specific to the NVU BM is largely lacking, making it difficult to screen and rationally design biomimetic ECM mixtures for BBB modeling. Thus, future studies that profile and analyze the brain vascular BM composition and dynamics would accelerate design of defined mixtures of ECM components that are more physiologically relevant and/or provide improved functional phenotypes of BMECs. Although better proteomic data may inform optimal ECM coatings for in vitro BBB models, such “bottom-up” methods (i.e., building mixtures with individual components) could still be difficult due to challenges in sourcing and scaffolding specific proteins and protein isoforms. Future studies should also utilize decellularized matrices from primary or hPSC-derived cells to elucidate how BM components produced by different cell types affect BBB properties. Proteomic profiling of the decellularized matrices followed by “top-down” methods (e.g., selective depletion of components via gene disruption or protein blocking) may also provide insight into the roles of various ECM molecules in regulating BBB function. In addition to the BM on the abluminal side, the endothelial glycocalyx (EG) on the luminal side of BMECs is also comprised of a mixture of ECM molecules (e.g., proteoglycan proteins and glycosaminoglycan chains) synthesized by BMECs and has been found to be important for regulating the BBB (Kabedev and Lobaskin, 2018; Jin et al., 2021; Zhao et al., 2021). Although it would be challenging or impractical to artificially deposit ECM molecules on BMECs to simulate the EG in in vitro BBB models, future studies should assess the ECM secreted by BMECs on the luminal side to benchmark how well the EG is recapitulated in in vitro models, and to make appropriate improvements.
Both the BBB and BM change during development and under disease conditions, but we have insufficient understanding about what changes occur and the mechanisms by which these changes regulate developmental or disease progression. As discussed in previous sections, a few studies have explored how different laminin isoforms used at certain time points of the experiments affect the in vitro human BBB models, but none has investigated effects of dynamic changes in laminin and/or other ECM coating composition that may be physiologically relevant for BMEC cultures. An hPSC-derived BBB model incorporating BM dynamics would be a powerful tool to assess the roles of ECM in human BBB development and neurodegenerative diseases and to develop new ECM-based strategies to restore BBB function during disease.
Author contributions
FD: Conceptualization, writing–original draft and editing; ES: Conceptualization, supervision, writing–review and editing, SP: Conceptualization, supervision, writing–review and editing.
Funding
This work was supported by the National Institutes of Health (grant numbers NS103844, NS107461, NS109486, and HL154254).
Conflict of interest
The authors declare that the research was conducted in the absence of any commercial or financial relationships that could be construed as a potential conflict of interest.
Publisher’s note
All claims expressed in this article are solely those of the authors and do not necessarily represent those of their affiliated organizations, or those of the publisher, the editors and the reviewers. Any product that may be evaluated in this article, or claim that may be made by its manufacturer, is not guaranteed or endorsed by the publisher.
References
Aazmi, A., Zhou, H., Lv, W., Yu, M., Xu, X., Yang, H., et al. (2022). Vascularizing the brain in vitro. iScience 25, 104110. doi:10.1016/j.isci.2022.104110
Abbott, N. J. (1992). “Comparative physiology of the blood-brain barrier,” in Physiology and pharmacology of the blood–brain barrier (Berlin, Germany: Springer), 371–396. doi:10.1007/978-3-642-76894-1_15
Abbott, N. J., Hughes, C. C., Revest, P. A., and Greenwood, J. (1992). Development and characterisation of a rat brain capillary endothelial culture: Towards an in vitro blood-brain barrier. J. Cell Sci. 103, 23–37. doi:10.1242/jcs.103.1.23
Abbott, N. J., Rönnbäck, L., and Hansson, E. (2006). Astrocyte-endothelial interactions at the blood-brain barrier. Nat. Rev. Neurosci. 7, 41–53. doi:10.1038/nrn1824
Anik, I., Kokturk, S., Genc, H., Cabuk, B., Koc, K., Yavuz, S., et al. (2011). Immunohistochemical analysis of TIMP-2 and collagen types I and IV in experimental spinal cord ischemia–reperfusion injury in rats. J. Spinal Cord. Med. 34, 257–264. doi:10.1179/107902611X12972448729648
Aoki, H., Yamashita, M., Hashita, T., Iwao, T., and Matsunaga, T. (2020). Laminin 221 fragment is suitable for the differentiation of human induced pluripotent stem cells into brain microvascular endothelial-like cells with robust barrier integrity. Fluids Barriers CNS 17, 25. doi:10.1186/s12987-020-00186-4
Arikawa-Hirasawa, E., Watanabe, H., Takami, H., Hassell, J. R., and Yamada, Y. (1999). Perlecan is essential for cartilage and cephalic development. Nat. Genet. 23, 354–358. doi:10.1038/15537
Arimori, T., Miyazaki, N., Mihara, E., Takizawa, M., Taniguchi, Y., Cabañas, C., et al. (2021). Structural mechanism of laminin recognition by integrin. Nat. Commun. 12, 4012. doi:10.1038/s41467-021-24184-8
Armulik, A., Genové, G., Mäe, M., Nisancioglu, M. H., Wallgard, E., Niaudet, C., et al. (2010). Pericytes regulate the blood-brain barrier. Nature 468, 557–561. doi:10.1038/nature09522
Bader, B. L., Smyth, N., Nedbal, S., Miosge, N., Baranowsky, A., Mokkapati, S., et al. (2005). Compound genetic ablation of nidogen 1 and 2 causes basement membrane defects and perinatal lethality in mice. Mol. Cell Biol. 25, 6846–6856. doi:10.1128/MCB.25.15.6846-6856.2005
Baeten, K. M., and Akassoglou, K. (2011). Extracellular matrix and matrix receptors in blood-brain barrier formation and stroke. Dev. Neurobiol. 71, 1018–1039. doi:10.1002/dneu.20954
Barber, A. J., and Lieth, E. (1997). Agrin accumulates in the brain microvascular basal lamina during development of the blood-brain barrier. Dev. Dyn. 208, 62–74. doi:10.1002/(sici)1097-0177(199701)208:1<62::aid-aja6>3.0.co;2
Baumann, E., Preston, E., Slinn, J., and Stanimirovic, D. (2009). Post-ischemic hypothermia attenuates loss of the vascular basement membrane proteins, agrin and SPARC, and the blood–brain barrier disruption after global cerebral ischemia. Brain Res. 1269, 185–197. doi:10.1016/j.brainres.2009.02.062
Belkin, A. M., and Stepp, M. A. (2000). Integrins as receptors for laminins. Microsc. Res. Tech. 51, 280–301. doi:10.1002/1097-0029(20001101)51:3<280::AID-JEMT7>3.0.CO;2-O
Bertrand, E., Lewandowska, E., Stepień, T., Szpak, G. M., Pasennik, E., and Modzelewska, J. (2008). Amyloid angiopathy in idiopathic Parkinson’s disease. Immunohistochemical and ultrastructural study. Folia Neuropathol. 46, 255–270.
Bezakova, G., and Ruegg, M. A. (2003). New insights into the roles of agrin. Nat. Rev. Mol. Cell Biol. 4, 295–309. doi:10.1038/nrm1074
Booth, H. D. E., Hirst, W. D., and Wade-Martins, R. (2017). The role of astrocyte dysfunction in Parkinson’s disease pathogenesis. Trends Neurosci. 40, 358–370. doi:10.1016/j.tins.2017.04.001
Bourasset, F., Ouellet, M., Tremblay, C., Julien, C., Do, T. M., Oddo, S., et al. (2009). Reduction of the cerebrovascular volume in a transgenic mouse model of Alzheimer’s disease. Neuropharmacology 56, 808–813. doi:10.1016/j.neuropharm.2009.01.006
Bradbury, M. (1979). Why a blood-brain barrier? Trends Neurosci. 2, 36–38. doi:10.1016/0166-2236(79)90016-X
Calabria, A. R., Weidenfeller, C., Jones, A. R., de Vries, H. E., and Shusta, E. v. (2006). Puromycin-purified rat brain microvascular endothelial cell cultures exhibit improved barrier properties in response to glucocorticoid induction. J. Neurochem. 97, 922–933. doi:10.1111/j.1471-4159.2006.03793.x
Canfield, S. G., Stebbins, M. J., Faubion, M. G., Gastfriend, B. D., Palecek, S. P., and Shusta, E. v. (2019). An isogenic neurovascular unit model comprised of human induced pluripotent stem cell-derived brain microvascular endothelial cells, pericytes, astrocytes, and neurons. Fluids Barriers CNS 16, 25. doi:10.1186/s12987-019-0145-6
Chan, J. P., Wong, B. H., Chin, C. F., Galam, D. L. A., Foo, J. C., Wong, L. C., et al. (2018). The lysolipid transporter Mfsd2a regulates lipogenesis in the developing brain. PLoS Biol. 16, e2006443. doi:10.1371/journal.pbio.2006443
Cirrito, J. R. (2005). P-glycoprotein deficiency at the blood-brain barrier increases amyloid-deposition in an Alzheimer disease mouse model. J. Clin. Investigation 115, 3285–3290. doi:10.1172/JCI25247
Claudio, L. (1995). Ultrastructural features of the blood-brain barrier in biopsy tissue from Alzheimer’s disease patients. Acta Neuropathol. 91, 6–14. doi:10.1007/s004010050386
Coatti, G. C., Frangini, M., Valadares, M. C., Gomes, J. P., Lima, N. O., Cavaçana, N., et al. (2017). Pericytes extend survival of ALS SOD1 mice and induce the expression of antioxidant enzymes in the murine model and in IPSCs derived neuronal cells from an ALS patient. Stem Cell Rev. Rep. 13, 686–698. doi:10.1007/s12015-017-9752-2
Costell, M., Gustafsson, E., Aszódi, A., Mörgelin, M., Bloch, W., Hunziker, E., et al. (1999). Perlecan maintains the integrity of cartilage and some basement membranes. J. Cell Biol. 147, 1109–1122. doi:10.1083/jcb.147.5.1109
Daneman, R., Zhou, L., Kebede, A. A., and Barres, B. A. (2010). Pericytes are required for blood-brain barrier integrity during embryogenesis. Nature 468, 562–566. doi:10.1038/nature09513
DeBault, L. E., and Cancilla, P. A. (1980). γ-Glutamyl transpeptidase in isolated brain endothelial cells: Induction by glial cells in vitro. Science 207, 653–655. doi:10.1126/science.6101511
Dehouck, M.-P., Méresse, S., Delorme, P., Fruchart, J.-C., and Cecchelli, R. (1990). An easier, reproducible, and mass-production method to study the blood-brain barrier in vitro. J. Neurochem. 54, 1798–1801. doi:10.1111/j.1471-4159.1990.tb01236.x
Delsing, L., Kallur, T., Zetterberg, H., Hicks, R., and Synnergren, J. (2019). Establishment of an in vitro human blood-brain barrier model derived from induced pluripotent stem cells and comparison to a porcine cell-based system. Fluids Barriers CNS 16, 27. doi:10.1186/s12987-019-0147-4
di Marco, A., Vignone, D., Gonzalez Paz, O., Fini, I., Battista, M. R., Cellucci, A., et al. (2020). Establishment of an in vitro human blood-brain barrier model derived from induced pluripotent stem cells and comparison to a porcine cell-based system. Cells 9, 994. doi:10.3390/cells9040994
Dohgu, S., Takata, F., Yamauchi, A., Nakagawa, S., Egawa, T., Naito, M., et al. (2005). Brain pericytes contribute to the induction and up-regulation of blood–brain barrier functions through transforming growth factor-β production. Brain Res. 1038, 208–215. doi:10.1016/j.brainres.2005.01.027
Dong, L., Chen, Y., Lewis, M., Hsieh, J.-C., Reing, J., Chaillet, J. R., et al. (2002). Neurologic defects and selective disruption of basement membranes in mice lacking entactin-1/nidogen-1. Lab. Investig. 82, 1617–1630. doi:10.1097/01.LAB.0000042240.52093.0F
Dufourcq, P., Louis, H., Moreau, C., Daret, D., Boisseau, M. R., Lamazière, J. M., et al. (1998). Vitronectin expression and interaction with receptors in smooth muscle cells from human atheromatous plaque. Arterioscler. Thromb. Vasc. Biol. 18, 168–176. doi:10.1161/01.atv.18.2.168
Erickson, M. A., Wilson, M. L., and Banks, W. A. (2020). In vitro modeling of blood–brain barrier and interface functions in neuroimmune communication. Fluids Barriers CNS 17, 26. doi:10.1186/s12987-020-00187-3
Fan, L. M., Geng, L., Cahill-Smith, S., Liu, F., Douglas, G., Mckenzie, C.-A., et al. (2019). Nox2 contributes to age-related oxidative damage to neurons and the cerebral vasculature. J. Clin. Investigation 129, 3374–3386. doi:10.1172/JCI125173
Farach-Carson, M. C., and Carson, D. D. (2007). Perlecan a multifunctional extracellular proteoglycan scaffold. Glycobiology 17, 897–905. doi:10.1093/glycob/cwm043
Farkas, E., de Jong, G. I., de Vos, R. A. I., Jansen Steur, E. N. H., and Luiten, P. G. M. (2000). Pathological features of cerebral cortical capillaries are doubled in Alzheimer’s disease and Parkinson’s disease. Acta Neuropathol. 100, 395–402. doi:10.1007/s004010000195
Fox, J. W., Mayer, U., Nischt, R., Aumailley, M., Reinhardt, D., Wiedemann, H., et al. (1991). Recombinant nidogen consists of three globular domains and mediates binding of laminin to collagen type IV. EMBO J. 10, 3137–3146. doi:10.1002/j.1460-2075.1991.tb04875.x
Freshney, R. I. (1986). Animal cell culture: A practical approach. Oxford and Washington, DC: IRL Press.
Fridman, R., Giaccone, G., Kanemoto, T., Martin, G. R., Gazdar, A. F., and Mulshine, J. L. (1990). Reconstituted basement membrane (matrigel) and laminin can enhance the tumorigenicity and the drug resistance of small cell lung cancer cell lines. Proc. Natl. Acad. Sci. 87, 6698–6702. doi:10.1073/pnas.87.17.6698
Frieser, M., Nöckel, H., Pausch, F., Röder, C., Hahn, A., Deutzmann, R., et al. (1997). Cloning of the mouse laminin alpha4 cDNA. Expression in a subset of endothelium. Eur. J. Biochem. 246, 727–735. doi:10.1111/j.1432-1033.1997.t01-1-00727.x
Fukuda, S., Fini, C. A., Mabuchi, T., Koziol, J. A., Eggleston, L. L., and del Zoppo, G. J. (2004). Focal cerebral ischemia induces active proteases that degrade microvascular matrix. Stroke 35, 998–1004. doi:10.1161/01.STR.0000119383.76447.05
Gama Sosa, M. A., Gasperi, R. de, Rocher, A. B., Wang, A. C.-J., Janssen, W. G. M., Flores, T., et al. (2010). Age-related vascular pathology in transgenic mice expressing presenilin 1-associated familial Alzheimer’s disease mutations. Am. J. Pathol. 176, 353–368. doi:10.2353/ajpath.2010.090482
Garbuzova-Davis, S., Haller, E., Saporta, S., Kolomey, I., Nicosia, S. v., and Sanberg, P. R. (2007). Ultrastructure of blood–brain barrier and blood–spinal cord barrier in SOD1 mice modeling ALS. Brain Res. 1157, 126–137. doi:10.1016/j.brainres.2007.04.044
Gastfriend, B. D., Foreman, K. L., Katt, M. E., Palecek, S. P., and Shusta, E. (2021a). Integrative analysis of the human brain mural cell transcriptome. J. Cereb. Blood Flow Metabolism 41, 3052–3068. doi:10.1177/0271678X211013700
Gastfriend, B. D., Nishihara, H., Canfield, S. G., Foreman, K. L., Engelhardt, B., Palecek, S. P., et al. (2021b). Wnt signaling mediates acquisition of blood–brain barrier properties in naïve endothelium derived from human pluripotent stem cells. Elife 10, e70992. doi:10.7554/eLife.70992
Gautam, J., Cao, Y., and Yao, Y. (2020). Pericytic laminin maintains blood-brain barrier integrity in an age-dependent manner. Transl. Stroke Res. 11, 228–242. doi:10.1007/s12975-019-00709-8
Gautam, J., Miner, J. H., and Yao, Y. (2019). Loss of endothelial laminin α5 exacerbates hemorrhagic brain injury. Transl. Stroke Res. 10, 705–718. doi:10.1007/s12975-019-0688-5
Gautam, J., Zhang, X., and Yao, Y. (2016). The role of pericytic laminin in blood brain barrier integrity maintenance. Sci. Rep. 6, 36450. doi:10.1038/srep36450
Gautam, M., Noakes, P. G., Moscoso, L., Rupp, F., Scheller, R. H., Merlie, J. P., et al. (1996). Defective neuromuscular synaptogenesis in agrin-deficient mutant mice. Cell 85, 525–535. doi:10.1016/S0092-8674(00)81253-2
Gelse, K. (2003). Collagens—Structure, function, and biosynthesis. Adv. Drug Deliv. Rev. 55, 1531–1546. doi:10.1016/j.addr.2003.08.002
Gorelick, P. B., Scuteri, A., Black, S. E., DeCarli, C., Greenberg, S. M., Iadecola, C., et al. (2011). Vascular contributions to cognitive impairment and dementia. Stroke 42, 2672–2713. doi:10.1161/STR.0b013e3182299496
Gould, D. B., Phalan, F. C., Breedveld, G. J., van Mil, S. E., Smith, R. S., Schimenti, J. C., et al. (2005). Mutations in Col4a1 cause perinatal cerebral hemorrhage and porencephaly. Science 308, 1167–1171. doi:10.1126/science.1109418
Gould, D. B., Phalan, F. C., van Mil, S. E., Sundberg, J. P., Vahedi, K., Massin, P., et al. (2006). Role of COL4A1 in small-vessel disease and hemorrhagic stroke. N. Engl. J. Med. 354, 1489–1496. doi:10.1056/NEJMoa053727
Grifno, G. N., Farrell, A. M., Linville, R. M., Arevalo, D., Kim, J. H., Gu, L., et al. (2019). Tissue-engineered blood-brain barrier models via directed differentiation of human induced pluripotent stem cells. Sci. Rep. 9, 13957. doi:10.1038/s41598-019-50193-1
Gu, Z., Cui, J., Brown, S., Fridman, R., Mobashery, S., Strongin, A., et al. (2005). A highly specific inhibitor of matrix metalloproteinase-9 rescues laminin from proteolysis and neurons from apoptosis in transient focal cerebral ischemia. J. Neurosci. 25, 6401–6408. doi:10.1523/JNEUROSCI.1563-05.2005
Guzelian, P. S., Li, D., Schuetz, E. G., Thomas, P., Levin, W., Mode, A., et al. (1988). Sex change in cytochrome P-450 phenotype by growth hormone treatment of adult rat hepatocytes maintained in a culture system on matrigel. Proc. Natl. Acad. Sci. 85, 9783–9787. doi:10.1073/pnas.85.24.9783
Hamann, G. F., Burggraf, D., Martens, H. K., Liebetrau, M., Jäger, G., Wunderlich, N., et al. (2004). Mild to moderate hypothermia prevents microvascular basal lamina antigen loss in experimental focal cerebral ischemia. Stroke 35, 764–769. doi:10.1161/01.STR.0000116866.60794.21
Hamann, G. F., Okada, Y., Fitridge, R., and del Zoppo, G. J. (1995). Microvascular basal lamina antigens disappear during cerebral ischemia and reperfusion. Stroke 26, 2120–2126. doi:10.1161/01.STR.26.11.2120
Hartmann, C., Zozulya, A., Wegener, J., and Galla, H.-J. (2007). The impact of glia-derived extracellular matrices on the barrier function of cerebral endothelial cells: An in vitro study. Exp. Cell Res. 313, 1318–1325. doi:10.1016/j.yexcr.2007.01.024
Hawkes, C. A., Gatherer, M., Sharp, M. M., Dorr, A., Yuen, H. M., Kalaria, R., et al. (2013). Regional differences in the morphological and functional effects of aging on cerebral basement membranes and perivascular drainage of amyloid-β from the mouse brain. Aging Cell 12, 224–236. doi:10.1111/acel.12045
Hawkes, C. A., Sullivan, P. M., Hands, S., Weller, R. O., Nicoll, J. A. R., and Carare, R. O. (2012). Disruption of arterial perivascular drainage of amyloid-β from the brains of mice expressing the human APOE ε4 allele. PLoS One 7, e41636. doi:10.1371/journal.pone.0041636
Hollmann, E. K., Bailey, A. K., Potharazu, A. v., Neely, M. D., Bowman, A. B., and Lippmann, E. S. (2017). Accelerated differentiation of human induced pluripotent stem cells to blood–brain barrier endothelial cells. Fluids Barriers CNS 14, 9. doi:10.1186/s12987-017-0059-0
Hori, S., Ohtsuki, S., Hosoya, K., Nakashima, E., and Terasaki, T. (2004). A pericyte-derived angiopoietin-1 multimeric complex induces occludin gene expression in brain capillary endothelial cells through Tie-2 activation in vitro. J. Neurochem. 89, 503–513. doi:10.1111/j.1471-4159.2004.02343.x
Horstmann, S., Kalb, P., Koziol, J., Gardner, H., and Wagner, S. (2003). Profiles of matrix metalloproteinases, their inhibitors, and laminin in stroke patients. Stroke 34, 2165–2170. doi:10.1161/01.STR.0000088062.86084.F2
Hughes, C. S., Postovit, L. M., and Lajoie, G. A. (2010). Matrigel: A complex protein mixture required for optimal growth of cell culture. Proteomics 10, 1886–1890. doi:10.1002/pmic.200900758
Igarashi, Y., Utsumi, H., Chiba, H., Yamada-Sasamori, Y., Tobioka, H., Kamimura, Y., et al. (1999). Glial cell line-derived neurotrophic factor induces barrier function of endothelial cells forming the blood–brain barrier. Biochem. Biophys. Res. Commun. 261, 108–112. doi:10.1006/bbrc.1999.0992
Jamieson, J. J., Linville, R. M., Ding, Y. Y., Gerecht, S., and Searson, P. C. (2019). Role of iPSC-derived pericytes on barrier function of iPSC-derived brain microvascular endothelial cells in 2D and 3D. Fluids Barriers CNS 16, 15. doi:10.1186/s12987-019-0136-7
Janzer, R. C., and Raff, M. C. (1987). Astrocytes induce blood–brain barrier properties in endothelial cells. Nature 325, 253–257. doi:10.1038/325253a0
Jeanne, M., Jorgensen, J., and Gould, D. B. (2015). Molecular and genetic analyses of collagen type IV mutant mouse models of spontaneous intracerebral hemorrhage identify mechanisms for stroke prevention. Circulation 131, 1555–1565. doi:10.1161/CIRCULATIONAHA.114.013395
Ji, K., and Tsirka, S. E. (2012). Inflammation modulates expression of laminin in the central nervous system following ischemic injury. J. Neuroinflammation 9, 610. doi:10.1186/1742-2094-9-159
Jin, J., Fang, F., Gao, W., Chen, H., Wen, J., Wen, X., et al. (2021). The structure and function of the glycocalyx and its connection with blood-brain barrier. Front. Cell Neurosci. 15, 739699. doi:10.3389/fncel.2021.739699
Jucker, M., Tian, M., Norton, D. D., Sherman, C., and Kusiak, J. W. (1996). Laminin α2 is a component of brain capillary basement membrane: Reduced expression in dystrophic dy mice. Neuroscience 71, 1153–1161. doi:10.1016/0306-4522(95)00496-3
Kabedev, A., and Lobaskin, V. (2018). Structure and elasticity of bush and brush-like models of the endothelial glycocalyx. Sci. Rep. 8, 240. doi:10.1038/s41598-017-18577-3
Katsu, M., Niizuma, K., Yoshioka, H., Okami, N., Sakata, H., and Chan, P. H. (2010). Hemoglobin-induced oxidative stress contributes to matrix metalloproteinase activation and blood–brain barrier dysfunction in vivo. J. Cereb. Blood Flow Metabolism 30, 1939–1950. doi:10.1038/jcbfm.2010.45
Katt, M. E., Linville, R. M., Mayo, L. N., Xu, Z. S., and Searson, P. C. (2018). Functional brain-specific microvessels from iPSC-derived human brain microvascular endothelial cells: The role of matrix composition on monolayer formation. Fluids Barriers CNS 15, 7. doi:10.1186/s12987-018-0092-7
Kleinman, H. K., McGarvey, M. L., Hassell, J. R., Star, V. L., Cannon, F. B., Laurie, G. W., et al. (1986). Basement membrane complexes with biological activity. Biochemistry 25, 312–318. doi:10.1021/bi00350a005
Kurosawa, T., Sako, D., Tega, Y., Debori, Y., Tomihara, Y., Aoyama, K., et al. (2022). Construction and functional evaluation of a three-dimensional blood–brain barrier model equipped with human induced pluripotent stem cell-derived brain microvascular endothelial cells. Pharm. Res. 39, 1535–1547. doi:10.1007/s11095-022-03249-3
Kwon, I., Kim, E. H., del Zoppo, G. J., and Heo, J. H. (2009). Ultrastructural and temporal changes of the microvascular basement membrane and astrocyte interface following focal cerebral ischemia. J. Neurosci. Res. 87, 668–676. doi:10.1002/jnr.21877
Lee, B., Clarke, D., al Ahmad, A., Kahle, M., Parham, C., Auckland, L., et al. (2011). Perlecan domain V is neuroprotective and proangiogenic following ischemic stroke in rodents. J. Clin. Investigation 121, 3005–3023. doi:10.1172/JCI46358
Lepelletier, F.-X., Mann, D. M. A., Robinson, A. C., Pinteaux, E., and Boutin, H. (2017). Early changes in extracellular matrix in Alzheimer’s disease. Neuropathol. Appl. Neurobiol. 43, 167–182. doi:10.1111/nan.12295
Li, Y., Li, Y., Pang, S., Huang, W., Zhang, A., Hawley, R. G., et al. (2014). Novel and functional ABCB1 gene variant in sporadic Parkinson’s disease. Neurosci. Lett. 566, 61–66. doi:10.1016/j.neulet.2014.02.025
Lian, X., Bao, X., Al-Ahmad, A., Liu, J., Wu, Y., Dong, W., et al. (2014). Efficient differentiation of human pluripotent stem cells to endothelial progenitors via small-molecule activation of WNT signaling. Stem Cell Rep. 3, 804–816. doi:10.1016/j.stemcr.2014.09.005
Linville, R. M., DeStefano, J. G., Sklar, M. B., Xu, Z., Farrell, A. M., Bogorad, M. I., et al. (2019). Human iPSC-derived blood-brain barrier microvessels: Validation of barrier function and endothelial cell behavior. Biomaterials 191, 24–37. doi:10.1016/j.biomaterials.2018.10.023
Linville, R. M., and Searson, P. C. (2021). Next-generation in vitro blood–brain barrier models: Benchmarking and improving model accuracy. Fluids Barriers CNS 18, 56. doi:10.1186/s12987-021-00291-y
Lippmann, E. S., Al-Ahmad, A., Azarin, S. M., Palecek, S. P., and Shusta, E. v. (2014). A retinoic acid-enhanced, multicellular human blood-brain barrier model derived from stem cell sources. Sci. Rep. 4, 4160. doi:10.1038/srep04160
Lippmann, E. S., Azarin, S. M., Kay, J. E., Nessler, R. A., Wilson, H. K., Al-Ahmad, A., et al. (2012). Derivation of blood-brain barrier endothelial cells from human pluripotent stem cells. Nat. Biotechnol. 30, 783–791. doi:10.1038/nbt.2247
Lippmann, E. S., Azarin, S. M., Palecek, S. P., and Shusta, E. v. (2020). Commentary on human pluripotent stem cell-based blood–brain barrier models. Fluids Barriers CNS 17, 64. doi:10.1186/s12987-020-00222-3
Liu, J.-X., Brännström, T., Andersen, P. M., and Pedrosa-Domellöf, F. (2011). Different impact of ALS on laminin isoforms in human extraocular muscles versus limb muscles. Investigative Opthalmology Vis. Sci. 52, 4842. doi:10.1167/iovs.10-7132
Lu, T. M., Barcia Durán, J. G., Houghton, S., Rafii, S., Redmond, D., and Lis, R. (2021a). Human induced pluripotent stem cell-derived brain endothelial cells: Current controversies. Front. Physiol. 12, 642812. doi:10.3389/fphys.2021.642812
Lu, T. M., Houghton, S., Magdeldin, T., Durán, J. G. B., Minotti, A. P., Snead, A., et al. (2021b). Pluripotent stem cell-derived epithelium misidentified as brain microvascular endothelium requires ETS factors to acquire vascular fate. Proc. Natl. Acad. Sci. 118, e2016950118. doi:10.1073/pnas.2016950118
Mancardi, G. L., Perdelli, F., Rivano, C., Leonardi, A., and Bugiani, O. (1980). Thickening of the basement membrane of cortical capillaries in Alzheimer’s disease. Acta Neuropathol. 49, 79–83. doi:10.1007/BF00692225
McColl, B. W., Rothwell, N. J., and Allan, S. M. (2008). Systemic inflammation alters the kinetics of cerebrovascular tight junction disruption after experimental stroke in mice. J. Neurosci. 28, 9451–9462. doi:10.1523/JNEUROSCI.2674-08.2008
Mehta, D. C., Short, J. L., and Nicolazzo, J. A. (2013). Altered brain uptake of therapeutics in a triple transgenic mouse model of Alzheimer’s disease. Pharm. Res. 30, 2868–2879. doi:10.1007/s11095-013-1116-2
Menezes, M. J., McClenahan, F. K., Leiton, C. v., Aranmolate, A., Shan, X., and Colognato, H. (2014). The extracellular matrix protein laminin α2 regulates the maturation and function of the blood–brain barrier. J. Neurosci. 34, 15260–15280. doi:10.1523/JNEUROSCI.3678-13.2014
Merlini, M., Meyer, E. P., Ulmann-Schuler, A., and Nitsch, R. M. (2011). Vascular β-amyloid and early astrocyte alterations impair cerebrovascular function and cerebral metabolism in transgenic arcAβ mice. Acta Neuropathol. 122, 293–311. doi:10.1007/s00401-011-0834-y
Mettouchi, A., Klein, S., Guo, W., Lopez-Lago, M., Lemichez, E., Westwick, J. K., et al. (2001). Integrin-specific activation of rac controls progression through the G1 phase of the cell cycle. Mol. Cell 8, 115–127. doi:10.1016/S1097-2765(01)00285-4
Milner, R. (2002). Developmental regulation of β1 integrins during angiogenesis in the central nervous system. Mol. Cell. Neurosci. 20, 616–626. doi:10.1006/mcne.2002.1151
Miyazaki, T., Futaki, S., Suemori, H., Taniguchi, Y., Yamada, M., Kawasaki, M., et al. (2012). Laminin E8 fragments support efficient adhesion and expansion of dissociated human pluripotent stem cells. Nat. Commun. 3, 1236. doi:10.1038/ncomms2231
Montagne, A., Nikolakopoulou, A. M., Zhao, Z., Sagare, A. P., Si, G., Lazic, D., et al. (2018). Pericyte degeneration causes white matter dysfunction in the mouse central nervous system. Nat. Med. 24, 326–337. doi:10.1038/nm.4482
Motallebnejad, P., and Azarin, S. M. (2020). Chemically defined human vascular laminins for biologically relevant culture of hiPSC-derived brain microvascular endothelial cells. Fluids Barriers CNS 17, 54. doi:10.1186/s12987-020-00215-2
Nakakura, T., Suzuki, T., Tanaka, H., Arisawa, K., Miyashita, T., Nekooki-Machida, Y., et al. (2021). Fibronectin is essential for formation of fenestrae in endothelial cells of the fenestrated capillary. Cell Tissue Res. 383, 823–833. doi:10.1007/s00441-020-03273-y
Nakamura, K., Ikeuchi, T., Nara, K., Rhodes, C. S., Zhang, P., Chiba, Y., et al. (2019). Perlecan regulates pericyte dynamics in the maintenance and repair of the blood–brain barrier. J. Cell Biol. 218, 3506–3525. doi:10.1083/jcb.201807178
Navone, S. E., Marfia, G., Invernici, G., Cristini, S., Nava, S., Balbi, S., et al. (2013). Isolation and expansion of human and mouse brain microvascular endothelial cells. Nat. Protoc. 8, 1680–1693. doi:10.1038/nprot.2013.107
Neal, E. H., Marinelli, N. A., Shi, Y., McClatchey, P. M., Balotin, K. M., Gullett, D. R., et al. (2019). A simplified, fully defined differentiation scheme for producing blood-brain barrier endothelial cells from human iPSCs. Stem Cell Rep. 12, 1380–1388. doi:10.1016/j.stemcr.2019.05.008
Nelson, A. R., Sweeney, M. D., Sagare, A. P., and Zlokovic, B. v. (2016). Neurovascular dysfunction and neurodegeneration in dementia and Alzheimer’s disease. Biochimica Biophysica Acta (BBA) - Mol. Basis Dis. 1862, 887–900. doi:10.1016/j.bbadis.2015.12.016
Patsch, C., Challet-Meylan, L., Thoma, E. C., Urich, E., Heckel, T., O’Sullivan, J. F., et al. (2015). Generation of vascular endothelial and smooth muscle cells from human pluripotent stem cells. Nat. Cell Biol. 17, 994–1003. doi:10.1038/ncb3205
Paulsson, M. (1992). Basement membrane proteins: Structure, assembly, and cellular interactions. Crit. Rev. Biochem. Mol. Biol. 27, 93–127. doi:10.3109/10409239209082560
Perrière, N., Demeuse, P. H., Garcia, E., Regina, A., Debray, M., Andreux, J.-P., et al. (2005). Puromycin-based purification of rat brain capillary endothelial cell cultures. Effect on the expression of blood-brain barrier-specific properties. J. Neurochem. 93, 279–289. doi:10.1111/j.1471-4159.2004.03020.x
Pöschl, E., Schlötzer-Schrehardt, U., Brachvogel, B., Saito, K., Ninomiya, Y., and Mayer, U. (2004). Collagen IV is essential for basement membrane stability but dispensable for initiation of its assembly during early development. Development 131, 1619–1628. doi:10.1242/dev.01037
Qian, T., Maguire, S. E., Canfield, S. G., Bao, X., Olson, W. R., Shusta, E. v., et al. (2017). Directed differentiation of human pluripotent stem cells to blood-brain barrier endothelial cells. Sci. Adv. 3, e1701679. doi:10.1126/sciadv.1701679
Ramsauer, M., Krause, D., and Dermietzel, R. (2002). Angiogenesis of the blood-brain barrier in vitro and the function of cerebral pericytes. FASEB J. 16, 1274–1276. doi:10.1096/fj.01-0814fje
Rauch, S. M., Huen, K., Miller, M. C., Chaudry, H., Lau, M., Sanes, J. R., et al. (2011). Changes in brain β-amyloid deposition and aquaporin 4 levels in response to altered agrin expression in mice. J. Neuropathol. Exp. Neurol. 70, 1124–1137. doi:10.1097/NEN.0b013e31823b0b12
Rohde, F., Danz, K., Jung, N., Wagner, S., and Windbergs, M. (2022). Electrospun scaffolds as cell culture substrates for the cultivation of an in vitro blood–brain barrier model using human induced pluripotent stem cells. Pharmaceutics 14, 1308. doi:10.3390/pharmaceutics14061308
Rosell, A., Cuadrado, E., Ortega-Aznar, A., Hernández-Guillamon, M., Lo, E. H., and Montaner, J. (2008). MMP-9–Positive neutrophil infiltration is associated to blood–brain barrier breakdown and basal lamina type IV collagen degradation during hemorrhagic transformation after human ischemic stroke. Stroke 39, 1121–1126. doi:10.1161/STROKEAHA.107.500868
Roudnicky, F., Kim, B. K., Lan, Y., Schmucki, R., Küppers, V., Christensen, K., et al. (2020). Identification of a combination of transcription factors that synergistically increases endothelial cell barrier resistance. Sci. Rep. 10, 3886. doi:10.1038/s41598-020-60688-x
Ruano-Salguero, J. S., and Lee, K. H. (2018). Efflux pump substrates shuttled to cytosolic or vesicular compartments exhibit different permeability in a quantitative human blood–brain barrier model. Mol. Pharm. 15, 5081–5088. doi:10.1021/acs.molpharmaceut.8b00662
Schymeinsky, J., Nedbal, S., Miosge, N., Pöschl, E., Rao, C., Beier, D. R., et al. (2002). Gene structure and functional analysis of the mouse nidogen-2 gene: Nidogen-2 is not essential for basement membrane formation in mice. Mol. Cell Biol. 22, 6820–6830. doi:10.1128/MCB.22.19.6820-6830.2002
Shao, J., Liu, Y., Gao, D., Tu, J., and Yang, F. (2021). Neural burst firing and its roles in mental and neurological disorders. Front. Cell Neurosci. 15, 741292. doi:10.3389/fncel.2021.741292
Shlosberg, D., Benifla, M., Kaufer, D., and Friedman, A. (2010). Blood–brain barrier breakdown as a therapeutic target in traumatic brain injury. Nat. Rev. Neurol. 6, 393–403. doi:10.1038/nrneurol.2010.74
Sixt, M., Engelhardt, B., Pausch, F., Hallmann, R., Wendler, O., and Sorokin, L. M. (2001). Endothelial cell laminin isoforms, laminins 8 and 10, play decisive roles in T cell recruitment across the blood–brain barrier in experimental autoimmune encephalomyelitis. J. Cell Biol. 153, 933–946. doi:10.1083/jcb.153.5.933
Solé, S., Petegnief, V., Gorina, R., Chamorro, Á., and Planas, A. M. (2004). Activation of matrix metalloproteinase-3 and agrin cleavage in cerebral ischemia/reperfusion. J. Neuropathol. Exp. Neurol. 63, 338–349. doi:10.1093/jnen/63.4.338
Song, J., Zhang, X., Buscher, K., Wang, Y., Wang, H., di Russo, J., et al. (2017). Endothelial basement membrane laminin 511 contributes to endothelial junctional tightness and thereby inhibits leukocyte transmigration. Cell Rep. 18, 1256–1269. doi:10.1016/j.celrep.2016.12.092
Sorokin, L., Girg, W., Gopfert, T., Hallmann, R., and Deutzmann, R. (1994). Expression of novel 400-kDa laminin chains by mouse and bovine endothelial cells. Eur. J. Biochem. 223, 603–610. doi:10.1111/j.1432-1033.1994.tb19031.x
Sorokin, L. M., Pausch, F., Frieser, M., Kröger, S., Ohage, E., and Deutzmann, R. (1997). Developmental regulation of the laminin α5 chain suggests a role in epithelial and endothelial cell maturation. Dev. Biol. 189, 285–300. doi:10.1006/dbio.1997.8668
Stebbins, M. J., Gastfriend, B. D., Canfield, S. G., Lee, M. S., Richards, D., Faubion, M. G., et al. (2019). Human pluripotent stem cell–derived brain pericyte–like cells induce blood-brain barrier properties. Sci. Adv. 5, eaau7375. doi:10.1126/sciadv.aau7375
Stratman, A. N., Malotte, K. M., Mahan, R. D., Davis, M. J., and Davis, G. E. (2009). Pericyte recruitment during vasculogenic tube assembly stimulates endothelial basement membrane matrix formation. Blood 114, 5091–5101. doi:10.1182/blood-2009-05-222364
Sweeney, M. D., Sagare, A. P., and Zlokovic, B. v. (2018). Blood-brain barrier breakdown in Alzheimer disease and other neurodegenerative disorders. Nat. Rev. Neurol. 14, 133–150. doi:10.1038/nrneurol.2017.188
Taub, M., Wang, Y., Szczesny, T. M., and Kleinman, H. K. (1990). Epidermal growth factor or transforming growth factor alpha is required for kidney tubulogenesis in matrigel cultures in serum-free medium. Proc. Natl. Acad. Sci. 87, 4002–4006. doi:10.1073/pnas.87.10.4002
Thyberg, J., Hedin, U., Sjölund, M., Palmberg, L., and Bottger, B. A. (1990). Regulation of differentiated properties and proliferation of arterial smooth muscle cells. Arteriosclerosis 10, 966–990. doi:10.1161/01.atv.10.6.966
Tilling, T., Engelbertz, C., Decker, S., Korte, D., Hüwel, S., and Galla, H.-J. (2002). Expression and adhesive properties of basement membrane proteins in cerebral capillary endothelial cell cultures. Cell Tissue Res. 310, 19–29. doi:10.1007/s00441-002-0604-1
Tilling, T., Korte, D., Hoheisel, D., and Galla, H.-J. (1998). Basement membrane proteins influence brain capillary endothelial barrier function in vitro. J. Neurochem. 71, 1151–1157. doi:10.1046/j.1471-4159.1998.71031151.x
Tornabene, E., and Brodin, B. (2016). Stroke and drug delivery— in vitro models of the ischemic blood-brain barrier. J. Pharm. Sci. 105, 398–405. doi:10.1016/j.xphs.2015.11.041
van Horssen, J., Bö, L., Dijkstra, C. D., and de Vries, H. E. (2006). Extensive extracellular matrix depositions in active multiple sclerosis lesions. Neurobiol. Dis. 24, 484–491. doi:10.1016/j.nbd.2006.08.005
van Horssen, J., Bö, L., Vos, C. M. P., Virtanen, I., and de Vries, H. E. (2005). Basement membrane proteins in multiple sclerosis-associated inflammatory cuffs: Potential role in influx and transport of leukocytes. J. Neuropathol. Exp. Neurol. 64, 722–729. doi:10.1097/01.jnen.0000173894.09553.13
Vanlandewijck, M., He, L., Mäe, M. A., Andrae, J., Ando, K., del Gaudio, F., et al. (2018). A molecular atlas of cell types and zonation in the brain vasculature. Nature 554, 475–480. doi:10.1038/nature25739
Vasudevan, A., Ho, M. S. P., Weiergräber, M., Nischt, R., Schneider, T., Lie, A., et al. (2009). Basement membrane protein nidogen-1 shapes hippocampal synaptic plasticity and excitability. Hippocampus NA-NA 20, 608. doi:10.1002/hipo.20660
Vosko, M. R., Busch, E., Burggraf, D., Bültemeier, G., and Hamann, G. F. (2003). Microvascular basal lamina damage in thromboembolic stroke in a rat model. Neurosci. Lett. 353, 217–220. doi:10.1016/j.neulet.2003.09.050
Webersinke, G., Bauer, H., Amberger, A., Zach, O., and Bauer, H. C. (1992). Comparison of gene expression of extracellular matrix molecules in brain microvascular endothelial cells and astrocytes. Biochem. Biophys. Res. Commun. 189, 877–884. doi:10.1016/0006-291X(92)92285-6
Weiss, N., Miller, F., Cazaubon, S., and Couraud, P. O. (2009). The blood-brain barrier in brain homeostasis and neurological diseases. Biochim. Biophys. Acta Biomembr. 1788, 842–857. doi:10.1016/j.bbamem.2008.10.022
Wiksten, M., Väänänen, A., and Liesi, P. (2007). Selective overexpression of γ1 laminin in astrocytes in amyotrophic lateral sclerosis indicates an involvement in ALS pathology. J. Neurosci. Res. 85, 2045–2058. doi:10.1002/jnr.21314
Workman, M. J., and Svendsen, C. N. (2020). Recent advances in human iPSC-derived models of the blood–brain barrier. Fluids Barriers CNS 17, 30. doi:10.1186/s12987-020-00191-7
Yan, L., Moriarty, R. A., and Stroka, K. M. (2021). Recent progress and new challenges in modeling of human pluripotent stem cell-derived blood-brain barrier. Theranostics 11, 10148–10170. doi:10.7150/thno.63195
Yang, P., Pavlovic, D., Waldvogel, H., Dragunow, M., Synek, B., Turner, C., et al. (2015). String vessel formation is increased in the brain of Parkinson disease. J. Park. Dis. 5, 821–836. doi:10.3233/JPD-140454
Yao, Y., Chen, Z.-L., Norris, E. H., and Strickland, S. (2014). Astrocytic laminin regulates pericyte differentiation and maintains blood brain barrier integrity. Nat. Commun. 5, 3413. doi:10.1038/ncomms4413
Yoshikawa, M., Aizawa, S., Oppenheim, R. W., and Milligan, C. (2022). Neurovascular unit pathology is observed very early in disease progression in the mutant SOD1G93A mouse model of amyotrophic lateral sclerosis. Exp. Neurol. 353, 114084. doi:10.1016/j.expneurol.2022.114084
Yousif, L. F., di Russo, J., and Sorokin, L. (2013). Laminin isoforms in endothelial and perivascular basement membranes. Cell Adh Migr. 7, 101–110. doi:10.4161/cam.22680
Zarow, C., Barron, E., Chui, H. C., and Perlmutter, L. S. (1997). Vascular basement membrane pathology and Alzheimer’s disease. Ann. N. Y. Acad. Sci. 826, 147–159. doi:10.1111/j.1749-6632.1997.tb48467.x
Zhao, F., Zhong, L., and Luo, Y. (2021). Endothelial glycocalyx as an important factor in composition of blood-brain barrier. CNS Neurosci. Ther. 27, 26–35. doi:10.1111/cns.13560
Zhong, D., Cao, Y., Li, C.-J., Li, M., Rong, Z.-J., Jiang, L., et al. (2020). Neural stem cell-derived exosomes facilitate spinal cord functional recovery after injury by promoting angiogenesis. Exp. Biol. Med. 245, 54–65. doi:10.1177/1535370219895491
Zlokovic, B. v. (2008). The blood-brain barrier in health and chronic neurodegenerative disorders. Neuron 57, 178–201. doi:10.1016/j.neuron.2008.01.003
Keywords: extracellular matrix, basement membrane, blood-brain barrier, pluripotent stem cells, in vitro models
Citation: Du F, Shusta EV and Palecek SP (2023) Extracellular matrix proteins in construction and function of in vitro blood-brain barrier models. Front. Chem. Eng. 5:1130127. doi: 10.3389/fceng.2023.1130127
Received: 22 December 2022; Accepted: 07 February 2023;
Published: 20 February 2023.
Edited by:
Xiaojun Lian, The Pennsylvania State University (PSU), United StatesReviewed by:
Qiang Li, Harvard University, United StatesWentao Dong, Stanford University, United States
Yuqian Jiang, Stanford University, United States
Abraham Jacob Al-Ahmad, Jerry H. Hodge School of Pharmacy, United States
Copyright © 2023 Du, Shusta and Palecek. This is an open-access article distributed under the terms of the Creative Commons Attribution License (CC BY). The use, distribution or reproduction in other forums is permitted, provided the original author(s) and the copyright owner(s) are credited and that the original publication in this journal is cited, in accordance with accepted academic practice. No use, distribution or reproduction is permitted which does not comply with these terms.
*Correspondence: Eric V. Shusta, ZXNodXN0YUB3aXNjLmVkdQ==; Sean P. Palecek, c3BwYWxlY2VrQHdpc2MuZWR1