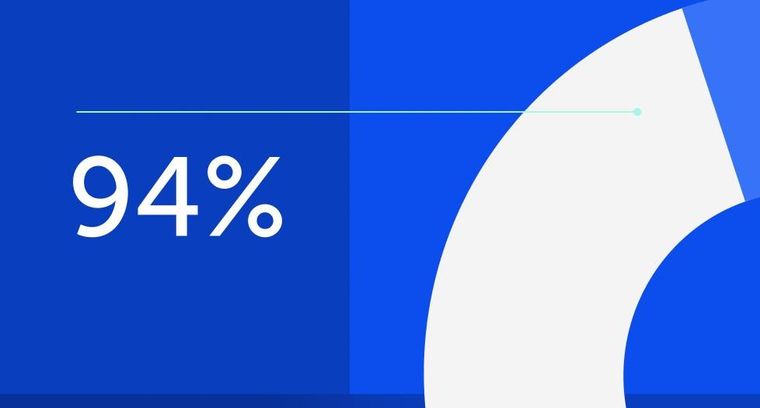
94% of researchers rate our articles as excellent or good
Learn more about the work of our research integrity team to safeguard the quality of each article we publish.
Find out more
ORIGINAL RESEARCH article
Front. Chem. Eng., 10 February 2023
Sec. Chemical Reaction Engineering
Volume 5 - 2023 | https://doi.org/10.3389/fceng.2023.1055896
This article is part of the Research TopicAdsorption-Enhanced Reactions: Design, Engineering and OptimizationView all 5 articles
In this work the continuous production of dimethyl ether (DME) by sorption-enhanced DME synthesis (SEDMES) technology has been demonstrated for the first time with a multi-column test-rig. A continuous single-pass carbon yield up to 95%, higher than ever reported before, has been achieved. The multi-column experiments have also shown that SEDMES can be operated at lower temperatures (220°C) than previously reported. This allows a higher temperature rise, making higher conversions possible while allowing even larger reactor tube diameters. Whereas the anticipated multi-tubular reactor concept is complex and costly, larger reactors could facilitate the economic valorisation. The SEDMES reactor model cannot only describe the transient behaviour of the process during the cyclic steady-state well, but also the dynamic approach towards the cyclic steady-state is adequately captured. Capturing the dynamic operation is of large interest with respect to process flexibility, especially for Power-to-X systems.
Dimethyl ether (DME), the simplest ether and the dehydrated form of methanol, is a valuable platform chemical and synthetic fuel. DME, which is gaseous at ambient conditions, can easily and safely be liquefied, transported and stored. In contrast to several other ethers it does not form explosive peroxides. The chemical and physical properties of DME, as well as the combustion characteristics, make that it can be used as fuel in domestic applications replacing LPG, in compression ignition engines (100% DME), in spark ignition engines (30% DME/70% LPG), and in power generation (Semelsberger et al., 2006; Arcoumanis et al., 2008; Azizi et al., 2014). Hence, DME is expected to play an important role in the energy transition, where fossil-based chemicals and fuels have to be replaced by products from renewable feedstocks, including the chemical recycling of carbon dioxide (Centi and Perathoner, 2009). Conventionally, DME is produced from synthesis gas with methanol as intermediate chemical. The following equilibrium reactions are involved in DME synthesis:
Methanol synthesis:
Water-gas shift:
Methanol dehydration:
Direct DME synthesis (from CO):
Sorption-enhanced direct DME synthesis (from CO2):
The indirect production of DME comprises the production of intermediate methanol, and the subsequent methanol dehydration (Bakhtyari and Rahimpour, 2018). The incomplete methanol and DME yields require extensive separation sections and recycles. The single-step direct DME synthesis process offers a reduction in process steps and increased overall conversion to DME (Ng et al., 1999; Dadgar et al., 2016; Mondal and Yadav, 2019; Liuzzi et al., 2020). Although the direct DME synthesis process outperforms the indirect process in terms of efficiency, separation and recycling are still required. The conventional production methods are considered unattractive, both because of heat management and equilibrium limitations, the latter especially when starting from CO2 instead of synthesis gas (Detz et al., 2018; Dieterich et al., 2020). Process intensification provides clear opportunities for improving the process performance (Bakhtyari and Rahimpour, 2018). As for many other industrial CO2 utilisation processes, the main hurdle is the production and efficient handling of steam (Centi and Perathoner, 2009; Kätelhön et al., 2019; van Kampen et al., 2019). Steam separation enhancement is shown to be a promising route for CO2 conversion; promising results have been reported using membranes and adsorbents (van Kampen et al., 2019; van Kampen et al., 2021a; Bakhtyari et al., 2021). In particular, as the focus of the current work, sorption-enhanced DME synthesis (SEDMES) is a novel process for the production of DME, which is based on in situ water removal by a solid adsorbent (Iliuta et al., 2011; Boon et al., 2017; van Kampen et al., 2018). Previous studies focused on the catalysis and adsorption aspects of the process (Boon et al., 2019; Liuzzi et al., 2020; van Kampen et al., 2021b). Experimental investigation into the single column bench-scale sorption-enhanced production of DME, including model validation, demonstrated over 80% single-pass carbon selectivity to DME with pressure swing regeneration using various feed compositions, including relatively CO2-rich feed. Pressure swing regeneration, rather than the time and energy intensive temperature swing regeneration, allows for a factor four increase in productivity. This is close to the reported direct DME pilot plant productivity for CO to DME, which would strongly deteriorate for CO2-rich feed, and further optimisation is still possible (van Kampen et al., 2020a; van Kampen et al., 2021c). Inherent to adsorption processes because of the required regeneration of the sorbent (typically a LTA zeolite), sorption-enhanced DME synthesis is a multi-column process (van Kampen et al., 2019; van Kampen et al., 2020b). Traditional single column reactor setups as used previously therefore do not allow demonstration and testing of continuous DME production. In this work, the SEDMES technology is validated experimentally with a multi-column test-rig under industrially relevant conditions. For the larger scale, continuous production of DME by sorption enhancement will be demonstrated for the first time in the open literature. Confirmation of the improvements by pressure-swing regeneration, as shown previously (van Kampen et al., 2020a; van Kampen et al., 2021c), is sought. The multi-column experiment allows to evaluate the system operating as an ensemble of columns, instead of earlier work focusing on adsorption and regeneration separately. Sorption-enhancement results in transient and dynamic processes in multiple columns (Carvill et al., 1996; Boon et al., 2015; Abanades et al., 2017; Rodrigues et al., 2017). In contrast to conventional processes operating at steady-state, there is a cyclic steady-state (CSS). The transient behaviour at cyclic steady-state is often investigated in detail. However, process flexibility for systems where the feedstock and operating conditions change, such as for Power-to-X (PtX) systems, could require dynamic operation (Miguel et al., 2017; Detz et al., 2018; Dieterich et al., 2020; Skorikova et al., 2020). Therefore, the dynamic operation is of particular interest for the multi-column experiments. As an ensemble, it is possible for the first time to study the transient behaviour of the system in between two cyclic steady states.
In this article the results of an experimental investigation on the multi-column sorption-enhanced production of DME are discussed, including the SEDMES reactor model for data interpretation. Attention is paid to the confirmation of pressure swing regeneration during the continuous production of DME. Additionally, the dynamic operation is studied in more detail, including operation at lower temperatures than previously reported for SEDMES.
In the next section, the used materials, the multi-column reactor setup and experimental procedures, and model interpretation are reported. In the results and discussion section, the experimental validation of continuous DME production is shown, results of the investigation into lower temperature operation are reported and the process dynamics are discussed. Finally, the main conclusions for the continuous multi-column sorption-enhanced dimethyl ether synthesis are summarized.
Experimental validation of sorption-enhanced DME synthesis was performed using a homogeneous physical mixture of commercially available catalyst and adsorbent: copper-zinc oxide-alumina (CZA) catalyst, γ-Al2O3 (assay>98%, Riogen NJ, USA), obtained as 3 mm pellets, and molecular sieve type 3A, purchased as 1.6 mm pellets (UOP Molecular Sieves, Obermeier, DE).
Nitrogen adsorption on the purchased γ-Al2O3 resulted in a Brunauer-Emmett-Teller (BET) specific surface area of 192 m2g−1 with an average pore diameter of 8.98 nm, which are within typical ranges reported for this type of material (Boon et al., 2019). The pore size of the molecular sieve type 3A is too small to characterize by nitrogen adsorption, however the adsorption capacity (max 25.5 wt% for the zeolite crystals) and mass transfer rate have been measured in more detail (van Kampen et al., 2021b).
A combination of commercially obtained CZA catalyst, γ-Al2O3 catalyst and zeolite 3A adsorbent was used for the experimental demonstration of the direct DME synthesis from CO2 with stoichiometric H2. The experimental runs were conducted on a multi-column high-pressure reactor setup (Figure 1) allowing continuous production with 36 L (divided over six columns of 6 m in length, 1.5″internal diameter) of sample, consisting of a 1:4 ratio (weight basis) catalyst (1:1 CZA: γ-Al2O3) to sorbent. The ratio between catalyst and sorbent was selected based on earlier investigations (van Kampen et al., 2020b) and not further optimised in this work. Adsorption was performed at 25 bar(a) in a temperature range of 220°C–250°C with argon as tracer. The inert balance was used to keep the overall pressure stable, considering the nett mole consumption by the reaction and the adsorption of water. Regeneration was done by depressurisation to 1–3bar(a) and switching to dry, inert gas (nitrogen) for the purge step. Finally, either the inert purge gas or the reactive feed gas was used for repressurisation. During depressurization and repressurization two columns can be physically connected, resulting in partial repressurization of one column by the depressurization gas from another column, called pressure equalization. Transient gas analysis was performed by Agilent CP490 micro-GC (with Thermal Conductivity Detectors (TCD); three channels: molsieve 5A 20 m, Poraplot PPU 10 m, CP-wax-52CB, all 0.25 mm id, 0.25 µm film; oven 80°C isotherm; carrier helium for the measurement of methane, CO, CO2, nitrogen, argon, methanol and DME) and mass spectrometry measuring hydrogen (m/z = 2), methane (m/z = 15), water (m/z = 18), carbon monoxide/nitrogen (m/z = 28), methanol (m/z = 31), carbon dioxide (m/z = 44) and DME (m/z = 45) for the outlet gas stream from reactor column 6.
FIGURE 1. Schematic of 2 interconnect reactor columns, including the various feed and product streams. The connections shown for one reactor column are identical for all six columns in the multi-column test-rig.
In order to facilitate data interpretation, several key metrics have been defined to be able to quantify the SEDMES performance. The most important metric, the carbon selectivity S(i), used here is defined as follows, (van Kampen et al., 2020b)
The carbon selectivities were calculated as molar concentration-based selectivities for each of the carbon containing species, y(i). For example, the selectivity towards DME can be calculated as
Considering CO2 as the carbon feed, the carbon selectivity to DME is also directly a measure of the product yield, whereas the carbon selectivity to CO2 is a measure of its conversion. Time integration (over the duration of a step) of the streams gives an overall yield and selectivity for the cyclic (steady state) performance of the SEDMES process.
A one-dimensional pseudo-homogeneous dynamic reactor model was developed in Matlab, verified and validated (van Kampen et al., 2020b; van Kampen et al., 2021c). For the description of the fluid flow, heat and mass transfer, the 1D non-steady differential mass, energy and momentum balances are solved. The total mass, momentum, component mass and overall energy balances are given in Table 1. The reaction rate equations are shown in Table 2. The reaction kinetics have been determined and validated under relevant conditions for the used catalyst materials by fitting the parameters in the kinetic models by Graaf et al. (1988) and Berčič et al. (1992) for the methanol synthesis, starting from CO, CO2 and mixtures thereof, and methanol dehydration respectively (Graaf et al., 1988; Berčič and Levec, 1992; Ng et al., 1999; Boon et al., 2019). Although not derived for sorption-enhanced DME synthesis, it has been shown previously that the relations provide a suitable means to describe kinetics in sorption-enhanced conditions (Boon et al., 2017; Liuzzi et al., 2020; van Kampen et al., 2021c). These reaction rate parameters are given in Table 3. The steam adsorption isotherm of the LTA zeolite adsorbent is determined under the high pressure and temperature working conditions of the SEDMES process (van Kampen et al., 2021b). Numerically, a single reactor column is simulated in time following the consecutive steps in the cycle. The obtained system of partial differential equations is solved in Matlab as a set of ordinary differential equations following the Method of Lines. A method for stiff differential equations is used with Matlab built-in variable-step, variable-order solver ode15s after uniform spatial discretization in finite differences, using a second-order TVD upwind approximation for the convective terms. The feed flow rate and temperature are specified at the reactor inlet, the pressure at the reactor outlet. Danckwerts boundary conditions are used for the heat and mass balances. Full details of the different aspects of the model can be found in previous work (van Kampen et al., 2020b).
TABLE 3. Reaction rate parameters for methanol synthesis and dehydration reaction kinetics (van Kampen et al., 2021c).
In the development of the sorption-enhanced DME synthesis process, for the first time the SEDMES technology is validated under industrially relevant conditions on a multi-column test-rig, allowing for continuous DME production. The previously developed model is used for data interpretation. Finally, learnings can be drawn from the multi-column experiments and improvements are suggested as the way forward to enhance the productivity and carbon selectivity.
The demonstration of the SEDMES technology on a multi-column test rig allows for continuous production of DME by sorption enhancement, for the first time in the open literature. Figure 2 shows the continuous concentration profiles (balance unconverted H2), collected as product during the reactive adsorption step which shifts to the subsequent reactor column. In Figure 3 the corresponding continuous DME outlet flowrate is shown for the multi-column test-rig compared to the discontinuous single column operation. Whereas only the outlet concentrations of reactor column 6 are continuously monitored, these concentrations are extrapolated to the other reactor columns which show very similar behaviour for the same step in the full cycle. In these experiments the nitrogen purge gas is used for repressurisation, therefore initially nitrogen is flushed out. After this flush, a representative breakthrough profile of sorption-enhanced DME synthesis is apparent from Figure 2. Prior to steam breakthrough, DME and unconverted CO are the primary products. After steam breakthrough the concentration of DME drops, accompanied by the breakthrough of CO2 and methanol indicating saturation of the adsorbent. Although the conversion is still far higher than conventional conversion levels (maximum of 1.9% DME), dictated by thermodynamics, the DME concentration drops relatively rapidly. Clearly, the purge times (35 min) used here, which are shorter than the adsorption times (45 min), do not regenerate the system completely and therefore result in a relatively fast steam breakthrough. By improving the regeneration, extending the pressure swing purge time, the theoretical maximum full conversion of CO2 to DME is approached. A carbon yield of up to 95% towards DME is observed, higher than ever reported before for experimental work on the direct conversion of CO2 to DME (Figure 4). However, here the classical trade-off between productivity, affected by the extent of regeneration, and the selectivity occurs (van Kampen et al., 2021c), (Kätelhön et al., 2019). Hence, the other experiments in this work are performed with 65%–90% carbon yield. Typical productivity is in the range of 0.17 kg h-1.
FIGURE 2. Experimental data at 250°C and 25 bar(a) for a CO2 feed with stoichiometric hydrogen, with argon tracer (24.5% CO2, 73.6% H2, 1.9% Ar, GHSV 93 h-1), measured for reactor column 6 (filled dots). For reactor columns 1–5 the data from column 6 is extrapolated (copied, open dots), for the purpose of interpreting the performance of the ensemble of columns.
FIGURE 3. Continuous DME outlet flow (after N2 flush) for the multi-column system and a single column, normalized with respect to the inlet carbon flow (carbon yield).
FIGURE 4. Comparison of experimental CO2 conversion and DME yield for sorption enhanced reactors (SER; green) and membrane reactors (MR; dark blue) with results for traditional reactors (TR; light blue), reported in the open literature. Adapted from (van Kampen et al., 2021a).
Sorption-enhanced DME synthesis was initially operated at 275°C (van Kampen et al., 2019; Liuzzi et al., 2020), which was lowered to 250°C with improvements in the performance, provided that both the catalyst activity and regeneration are sufficient (van Kampen et al., 2020a; van Kampen et al., 2021c). Also an initial modelling study indicated temperatures of around 250°C to be optimal for SEDMES (van Kampen et al., 2020b). The direct synthesis of DME is thermodynamically favoured at lower temperatures. However, the catalyst activity requires temperatures around 250°C. In sorption-enhanced synthesis, the temperature also affects the adsorption capacity. As indicated by the study of the adsorbent material, the adsorption capacity benefits from operation at lower temperatures (van Kampen et al., 2021b). Although with a pressure swing regeneration, the operating temperature must be balanced for both good adsorption and desorption properties, maximizing the cyclic working capacity.
As shown in Figure 5, the multi-column testing demonstrates SEDMES at temperatures as low as 220°C with very similar performance to the original higher temperature operation. As mentioned before, after an initial nitrogen flush (grey line), a representative SEDMES breakthrough profile can be seen. Prior to steam breakthrough, DME and unconverted CO are the primary products. After steam breakthrough the concentration of DME drops, accompanied by an increase of CO2 and methanol indicating the increasing adsorbent saturation. This temperature of 220°C is on the low side, especially for the methanol dehydration over an alumina catalyst. However, it has been observed that periodic regeneration can have a positive effect on catalyst activity and can even restore lost activity (van Kampen et al., 2018; Boon et al., 2019). This result seems very promising with respect to the heat management of the system. Both methanol synthesis and direct DME synthesis are exothermic reactions, limiting the conversion and deactivating the methanol catalyst by hydrothermal sintering, and therefore require a cooled reactor, such as a multi-tubular reactor concept (Song et al., 2008; Guffanti et al., 2021a; Guffanti et al., 2021b). In sorption-enhanced DME synthesis, the exothermic adsorption is added to the already exothermic reaction system. SEDMES temperature control appears not to be an issue in a multi-tubular cooled reactor. Larger tube diameters can be adopted compared to conventional direct DME synthesis. However, operation at lower temperatures would allow a higher maximum temperature rise, and therefore makes higher conversions possible and it allows even larger diameters of the tubes in the multi-tubular reactor (Guffanti et al., 2021a). Where a multi-tubular reactor concept is also a costly part of the DME synthesis process, larger reactors would benefit the economic valorisation of CO2 conversion (Skorikova et al., 2020).
FIGURE 5. Breakthrough experiment at 220°C and 25 bar(a) for a CO2 feed with stoichiometric hydrogen, with argon tracer (24.5% CO2, 73.6% H2, 1.9% Ar, GHSV 93 h-1), (dots; DME (green), CO (red), CO2 (black), methanol (blue), argon (yellow), methane (brown), nitrogen (grey)) and model prediction (lines).
Figure 5 also shows the typical transient response after sorbent regeneration and column repressurisation with nitrogen, during the feeding of CO2, H2, and Ar. A large DME peak appears, followed by a steady decline due to the gradual saturation of the column with water. The SEDMES reactor model adequately describes the transient concentration profiles at cyclic steady-state, confirming previous model validation. However, not only the behaviour at cyclic steady-state is described by the model. The SEDMES reactor model is a dynamic cycle model, simulating each step of a cycle towards cyclic steady-state. The model predicts the dynamic behaviour of the system, both the various steps in a cycle and consequently the subsequent cycles, very well, as demonstrated in Figure 6 by the approach to CSS observed experimentally and predicted by the model. Initially, in the first cycle shown in Figure 6, the adsorbent material is relatively dry. For the chosen operating conditions and cycle design, more water is adsorbed during the reactive adsorption step than desorbed during the regeneration. This results in an accumulation of water loaded on the adsorbent material, hence a lower working capacity and a decreasing carbon selectivity to DME until a cyclic steady-state is reached, where an equal amount of water is adsorbed and desorbed during a cycle.
FIGURE 6. Experimental carbon selectivity (dots) to DME (green), CO (red), CO2 (black) and methanol (blue) and model prediction (lines) as function of the number of subsequent cycles. The model working capacity q (dashed blue line) is shown on the right axis. Conditions: 220°C and 25 bar(a) for a CO2 feed with stoichiometric hydrogen, with argon tracer.
The approach to a new cyclic steady-state goes relatively quickly, especially if the CSS values are close to the old values. In Figure 7 it can be seen that the experimental carbon selectivity at 250°C reaches the predicted cyclic steady-state within approximately 5 cycles. Whereas, the working capacity is slightly higher (8.8%) at this elevated temperature, a small increase in the carbon selectivity to DME and a small decrease in the carbon selectivity to CO2 can be observed during the first cycles.
FIGURE 7. Experimental carbon selectivity (dots) to DME (green), CO (red), CO2 (black) and methanol (blue) as function of the number of subsequent cycles. Model prediction for cyclic steady-state (lines). Conditions: 250°C and 25 bar(a) for a CO2 feed with stoichiometric hydrogen, with argon tracer.
The dynamics of operation are an essential element in the Power-to-X (PtX) context where CO2 can be converted with intermittently produced green hydrogen from electrolysis (Miguel et al., 2017; Detz et al., 2018; Skorikova et al., 2020; Cloete et al., 2021). Whereas SEDMES is a dynamic process in nature, it allows for process flexibility and coping with this intermittency. Common process variables for adsorption processes include the cycle time and flowrates for the various process steps, which can be used to control performance parameters such as purity and productivity (de Witte et al., 2021). Here, integrating scheduling, by means of cycle design, and control strategies would result in more efficient and economical operation (Dias and Ierapetritou, 2016). However, the real-time dynamic performance strongly depends on these decision variables in a non-linear manner, making the modelling, simulation and control challenging (Dowling et al., 2012; Khajuria and Pistikopoulos, 2013). The complex and highly non-linear dynamic behaviour still poses challenges for the control of such intensified processes, but recent advances enable the solution of complex, non-linear problems and the implementation of non-linear controllers (Dias and Ierapetritou, 2019). The detailed investigation of SEDMES operation and control are outside the scope of the current work, but are part of currently ongoing research.
In this article, for the first time in the open literature, sorption-enhanced dimethyl ether synthesis (SEDMES) technology is validated experimentally on a multi-column test-rig under industrially relevant conditions. Multi-column operation allowed for the demonstration of continuous DME production by sorption enhancement, for which up to 95% carbon yield is observed. Pressure swing regeneration is confirmed as the preferential mode of regeneration, which is essential for further optimisation of the process.
The multi-column experiments have demonstrated that SEDMES can be operated at 220°C, which is lower than expected based on previous data. Operation at lower temperatures would allow for a higher maximum temperature rise and would ease the heat management of the system. Where the typical multi-tubular reactor concept for DME synthesis is also a costly part of the process, larger reactors would benefit the economic valorisation of the process.
SEDMES is a transient and dynamic process, resulting in more degrees of freedom compared to conventional technologies. The SEDMES reactor model not only describes the transient behaviour during cyclic steady-state well, also the dynamic approach to the cyclic steady-state is modelled well. Dynamic operation is of large interest with respect to process flexibility, especially for Power-to-X (PtX) systems.
In conclusion, the multi-column experiments have demonstrated the continuous production of DME with a high single-pass conversion of CO2. Experimental evidence, supported by modelling, of the dynamic operation at lower temperatures will allow further optimisation of a PtX SEDMES process.
The raw data supporting the conclusions of this article will be made available by the authors, without undue reservation.
JVK: Conceptualization, Methodology, Software, Formal analysis, Validation, Investigation, Visualization, Writing—Original Draft JO: Methodology, Investigation JB: Conceptualization, Writing—Review & Editing, Project administration, Funding acquisition MVS: Writing—Review & Editing, Resources, Supervision.
This work has received funding from the European Union’s Horizon 2020 research and innovation programme under grant agreement No 727600.
S. Booneveld and M. Koppes of TNO are kindly acknowledged for experimental support.
The authors declare that the research was conducted in the absence of any commercial or financial relationships that could be construed as a potential conflict of interest.
All claims expressed in this article are solely those of the authors and do not necessarily represent those of their affiliated organizations, or those of the publisher, the editors and the reviewers. Any product that may be evaluated in this article, or claim that may be made by its manufacturer, is not guaranteed or endorsed by the publisher.
Abanades, J. C., Boon, J., Cobden, P., Coenen, K., Constantino, D. S. M., Faria, R. P. V., et al. (2017). Sorption enhancement of chemical processes. 1st ed. Academic Press.
Arcoumanis, C., Bae, C., Crookes, R., and Kinoshita, E. (2008). The potential of di-methyl ether (DME) as an alternative fuel for compression-ignition engines: A review. Fuel 87, 1014–1030. doi:10.1016/j.fuel.2007.06.007
Azizi, Z., Rezaeimanesh, M., Tohidian, T., and Rahimpour, M. R. (2014). Dimethyl ether: A review of technologies and production challenges. Chem. Eng. Process. Process Intensif. 82, 150–172. doi:10.1016/j.cep.2014.06.007
Bakhtyari, A., Bardool, R., Rahimpour, M. R., and Iulianelli, A. (2021). Dehydration of bio-alcohols in an enhanced membrane-assisted reactor: A rigorous sensitivity analysis and multi-objective optimization. Renew. Energy. 177, 519–543. doi:10.1016/J.RENENE.2021.05.161
Bakhtyari, A., and Rahimpour, M. R. (2018). Methanol to dimethyl ether. Methanol Sci. Eng., 281–311. doi:10.1016/B978-0-444-63903-5.00010-8
Berčič, G., and Levec, J. (1992). Intrinsic and global reaction rate of methanol dehydration over.gamma.-alumina pellets. Ind. Eng. Chem. Res. 31, 1035–1040. doi:10.1021/ie00004a010
Boon, J., Cobden, P. D., van Dijk, H. A. J., and van Sint Annaland, M. (2015). High-temperature pressure swing adsorption cycle design for sorption-enhanced water-gas shift. Chem. Eng. Sci. 122, 219–231. doi:10.1016/j.ces.2014.09.034
Boon, J., van Berkel, F., van Dijk, H., and Vente, J. (2017). Separation enhanced dimethyl ether synthesis. Aachen: 5th TMFB International Conference.
Boon, J., van Kampen, J., Hoogendoorn, R., Tanase, S., van Berkel, F. P. F., and van Sint Annaland, M. (2019). Reversible deactivation of γ-alumina by steam in the gas-phase dehydration of methanol to dimethyl ether. Catal. Commun. 119, 22–27. doi:10.1016/j.catcom.2018.10.008
Carvill, B. T., Hufton, J. R., Anand, M., and Sircar, S. (1996). Sorption-enhanced reaction process. AIChE J. 42, 2765–2772. doi:10.1002/aic.690421008
Centi, G., and Perathoner, S. (2009). Opportunities and prospects in the chemical recycling of carbon dioxide to fuels. Catal. Today 148, 191–205. doi:10.1016/j.cattod.2009.07.075
Cloete, S., Ruhnau, O., and Hirth, L. (2021). On capital utilization in the hydrogen economy: The quest to minimize idle capacity in renewables-rich energy systems. Int. J. Hydrogen Energy 46, 169–188. doi:10.1016/j.ijhydene.2020.09.197
Dadgar, F., Myrstad, R., Pfeifer, P., Holmen, A., and Venvik, H. J. (2016). Direct dimethyl ether synthesis from synthesis gas: The influence of methanol dehydration on methanol synthesis reaction. Catal. Today 270, 76–84. doi:10.1016/j.cattod.2015.09.024
de Witte, N., Denayer, J. F. M., and van Assche, T. R. C. (2021). Effect of adsorption duration and purge flowrate on pressure swing adsorption performance. Ind. Eng. Chem. Res. 60, 13684–13691. doi:10.1021/acs.iecr.1c02291
Detz, R. J., Reek, J. N. H., and van der Zwaan, B. C. C. (2018). The future of solar fuels: When could they become competitive? Energy Environ. Sci. 11, 1653–1669. doi:10.1039/c8ee00111a
Dias, L. S., and Ierapetritou, M. G. (2016). Integration of scheduling and control under uncertainties: Review and challenges. Chem. Eng. Res. Des. 116, 98–113. doi:10.1016/j.cherd.2016.10.047
Dias, L. S., and Ierapetritou, M. G. (2019). Optimal operation and control of intensified processes — Challenges and opportunities. Curr. Opin. Chem. Eng. 25, 82–86. doi:10.1016/j.coche.2018.12.008
Dieterich, V., Buttler, A., Hanel, A., Spliethoff, H., and Fendt, S. (2020). Power-to-liquid via synthesis of methanol, DME or fischer–tropsch-fuels: A review. Energy Environ. Sci. 13, 3207–3252. doi:10.1039/d0ee01187h
Dowling, A. W., Vetukuri, S. R. R., and Biegler, L. T. (2012). Large-scale optimization strategies for pressure swing adsorption cycle synthesis. AIChE J. 58, 3777–3791. doi:10.1002/aic.13928
Graaf, G. H., Stamhuis, E. J., and Beenackers, A. A. C. M. (1988). Kinetics of low-pressure methanol synthesis. Chem. Eng. Sci. 43, 3185–3195. doi:10.1016/0009-2509(88)85127-3
Guffanti, S., Visconti, C. G., and Groppi, G. (2021). Model analysis of the role of kinetics, adsorption capacity, and heat and mass transfer effects in sorption enhanced dimethyl ether synthesis. Ind. Eng. Chem. Res. 60, 6767–6783. doi:10.1021/acs.iecr.1c00521
Guffanti, S., Visconti, C. G., van Kampen, J., Boon, J., and Groppi, G. (2021). Reactor modelling and design for sorption enhanced dimethyl ether synthesis. Chem. Eng. J. 404, 126573. doi:10.1016/j.cej.2020.126573
Iliuta, I., Iliuta, M. C., and Larachi, F. (2011). Sorption-enhanced dimethyl ether synthesis-Multiscale reactor modeling. Chem. Eng. Sci. 66, 2241–2251. doi:10.1016/j.ces.2011.02.047
Kätelhön, A., Meys, R., Deutz, S., Suh, S., and Bardow, A. (2019). Climate change mitigation potential of carbon capture and utilization in the chemical industry. Proc. Natl. Acad. Sci. U. S. A. 166, 11187–11194. doi:10.1073/pnas.1821029116
Khajuria, H., and Pistikopoulos, E. N. (2013). Optimization and control of pressure swing adsorption processes under uncertainty. AIChE J. 59, 120–131. doi:10.1002/aic.13783
Liuzzi, D., Peinado, C., Peña, M. A., van Kampen, J., Boon, J., and Rojas, S. (2020). Increasing dimethyl ether production from biomass-derived syngas via sorption enhanced dimethyl ether synthesis. Sustain Energy Fuels 4, 5674–5681. doi:10.1039/D0SE01172J
Miguel, C. v., Soria, M. A., Mendes, A., and Madeira, L. M. (2017). A sorptive reactor for CO2 capture and conversion to renewable methane. Chem. Eng. J. 322, 590–602. doi:10.1016/j.cej.2017.04.024
Mondal, U., and Yadav, G. D. (2019). Perspective of dimethyl ether as fuel: Part I. Catalysis. J. CO2 Util. 32, 299–320. doi:10.1016/j.jcou.2019.02.003
Ng, K. L., Chadwick, D., and Toseland, B. A. (1999). Kinetics and modelling of dimethyl ether synthesis from synthesis gas. Chem. Eng. Sci. 54, 3587–3592. doi:10.1016/S0009-2509(98)00514-4
Rodrigues, A. E., Madeira, L. M., Wu, Y.-J., and Faria, R. (2017). Sorption enhanced reaction processes. World Scientific.
Semelsberger, T. A., Borup, R. L., and Greene, H. L. (2006). Dimethyl ether (DME) as an alternative fuel. J. Power Sources 156, 497–511. doi:10.1016/j.jpowsour.2005.05.082
Skorikova, G., Saric, M., Sluijter, S. N., van Kampen, J., Sánchez-Martínez, C., and Boon, J. (2020). The techno-economic benefit of sorption enhancement: Evaluation of sorption-enhanced dimethyl ether synthesis for CO2 utilization. Front. Chem. Eng. 2, 2. doi:10.3389/fceng.2020.594884
Song, D., Cho, W., Lee, G., Park, D. K., and Yoon, E. S. (2008). Numerical analysis of a pilot-scale fixed-bed reactor for dimethyl ether (DME) synthesis. Ind. Eng. Chem. Res. 47, 4553–4559. doi:10.1021/ie071589e
van Kampen, J., Boon, J., van Berkel, F., van Dijk, H., Vente, J., and van Sint Annaland, M. (2018). “Regeneration conditions as the key to sorption enhanced dimethyl ether synthesis,” in 5th international symposium on chemical reaction engineering (Florence.
van Kampen, J., Boon, J., van Berkel, F., Vente, J., and van Sint Annaland, M. (2019). Steam separation enhanced reactions: Review and outlook. Chem. Eng. J. 374, 1286–1303. doi:10.1016/j.cej.2019.06.031
van Kampen, J., Boon, J., and van Sint Annaland, M. (2021). Separation enhanced methanol and dimethyl ether synthesis. J. Mater Chem. A Mater 9, 14627–14629. doi:10.1039/D1TA03405G
van Kampen, J., Boon, J., and van Sint Annaland, M. (2021). Steam adsorption on molecular sieve 3A for sorption enhanced reaction processes. Adsorption 27, 577–589. doi:10.1007/s10450-020-00283-8
van Kampen, J., Boon, J., Vente, J., and van Sint Annaland, M. (2020). Sorption enhanced dimethyl ether synthesis for high efficiency carbon conversion: Modelling and cycle design. J. CO2 Util. 37, 295–308. doi:10.1016/j.jcou.2019.12.021
van Kampen, J., Boon, J., Vente, J., and van Sint Annaland, M. (2021). Sorption enhanced dimethyl ether synthesis under industrially relevant conditions: Experimental validation of pressure swing regeneration. React. Chem. Eng. 6, 244–257. doi:10.1039/D0RE00431F
van Kampen, J., Booneveld, S., Boon, J., Vente, J., and van Sint Annaland, M. (2020). Experimental validation of pressure swing regeneration for faster cycling in sorption enhanced dimethyl ether synthesis. Chem. Commun. 56, 13540–13542. doi:10.1039/D0CC06093C
ap Particle interfacial area (m2 m−3)
ci Concentration of component i (mol m−3)
Cp Specific heat capacity gas (J kg−1 K−1)
Cpp Specific heat capacity particles (J kg−1 K−1)
dp Particle diameter (m)
Dz Axial dispersion coefficient (m2 s−1)
G Ergun constant (−)
ΔHads Adsorption enthalpy (J mol−1)
ΔHr,i Reaction enthalpy (J mol−1)
Mi Molecular weight of component i (kg mol−1)
Ni Mole flux of component i (mol m−2 s−1)
P Reactor pressure (bara)
qi Adsorbent loading (mol kg−1)
ri Reaction rate of component i (mol m−3 s−1) or (mol kg−1 s−1)
or (kmol kg−1 hr−1)
R Ideal gas constant (J mol−1 K−1)
t Time (s)
T Temperature (K)
u Superficial gas velocity (m s−1)
U Overall heat transfer coefficient (W m−2 K−1)
v Interstitial gas velocity (m s−1)
z axial coordinate (m)
εb Bed voidage (−)
λ Axial thermal conductivity (W m−1 Kis1)
ρ Density (kg m−3)
ρp Particle density (kg m−3)
ωi Weight fraction of component i (−)
Keywords: CO2 utilization, continuous cyclic production, dimethyl ether, reactive adsorption, steam separation enhancement
Citation: van Kampen J, Overbeek J, Boon J and van Sint Annaland M (2023) Continuous multi-column sorption-enhanced dimethyl ether synthesis (SEDMES): Dynamic operation. Front. Chem. Eng. 5:1055896. doi: 10.3389/fceng.2023.1055896
Received: 28 September 2022; Accepted: 30 January 2023;
Published: 10 February 2023.
Edited by:
Akshat Tanksale, Monash University, AustraliaReviewed by:
Ali Bakhtyari, Shiraz University, IranCopyright © 2023 van Kampen, Overbeek, Boon and van Sint Annaland. This is an open-access article distributed under the terms of the Creative Commons Attribution License (CC BY). The use, distribution or reproduction in other forums is permitted, provided the original author(s) and the copyright owner(s) are credited and that the original publication in this journal is cited, in accordance with accepted academic practice. No use, distribution or reproduction is permitted which does not comply with these terms.
*Correspondence: Jasper van Kampen, amFzcGVyLnZhbi1rYW1wZW5AdGF0YXN0ZWVsZXVyb3BlLmNvbQ==; Jurriaan Boon, anVycmlhYW4uYm9vbkB0bm8ubmw=; Martin van Sint Annaland, bS52LnNpbnRhbm5hbGFuZEB0dWUubmw=
†Present address: Jasper van Kampen, Tata Steel Europe, IJmuiden, Netherlands
Disclaimer: All claims expressed in this article are solely those of the authors and do not necessarily represent those of their affiliated organizations, or those of the publisher, the editors and the reviewers. Any product that may be evaluated in this article or claim that may be made by its manufacturer is not guaranteed or endorsed by the publisher.
Research integrity at Frontiers
Learn more about the work of our research integrity team to safeguard the quality of each article we publish.