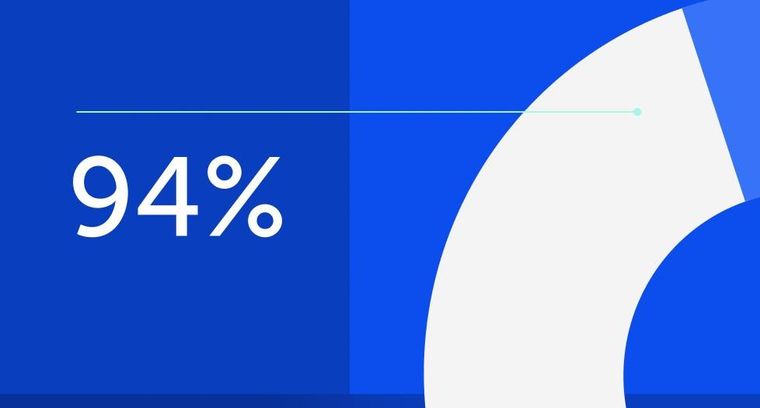
94% of researchers rate our articles as excellent or good
Learn more about the work of our research integrity team to safeguard the quality of each article we publish.
Find out more
REVIEW article
Front. Chem. Eng., 06 October 2022
Sec. Sustainable Process Engineering
Volume 4 - 2022 | https://doi.org/10.3389/fceng.2022.998289
This article is part of the Research TopicBioprocess Designing Towards Clean Energy Production From Industrial WastewaterView all 12 articles
Globally, the demand for energy is increasing with an emphasis on green fuels for a sustainable future. As the urge for alternative fuels is accelerating, microalgae have emerged as a promising source that can not only produce high lipid but many other platform chemicals. Moreover, it is a better alternative in comparison to conventional feedstock due to yearlong easy and mass cultivation, carbon fixation, and value-added products extraction. To date, numerous studies have been done to elucidate these organisms for large-scale fuel production. However, enhancing the lipid synthesis rate and reducing the production cost still remain a major bottleneck for its economic viability. Therefore, this study compiles information on algae-based biodiesel production with an emphasis on its unit operations from strain selection to biofuel production. Additionally, strategies to enhance lipid accumulation by incorporating genetic, and metabolic engineering and the use of leftover biomass for harnessing bio-products have been discussed. Besides, implementing a biorefinery for extracting oil followed by utilizing leftover biomass to generate value-added products such as nanoparticles, biofertilizers, biochar, and biopharmaceuticals has also been discussed.
Over the decade, limitation in availability of fossil fuels and increasing population has resulted in enhanced energy demands. Furthermore, continuous exploitation of non-renewable energy sources has embarked the accumulation of carbon dioxide (CO2) in the atmosphere from 295 parts per million (ppm) to more than 400 ppm in 2018 leading to global warming and climate change (Tang et al., 2020) (Rosa et al., 2019). According to some studies, it is estimated the current rate of fossil fuel consumption including natural gas, coal, and crude oil will lead to their exhaustion in approximately 50 years. Therefore, the green energy demand is set to increase from 43 billion L/day in 2020 to 55.96 billion L/day by 2045 (Organization of the Petroleum Exporting Countries - OPEC, 2020). Additionally, reports published by International Energy Agency (IEA) states that the biofuels market was valued at nearly 110 billion USD in 2021 and will increase to 2012 billion USD by 2030 (IEA, 2021). Therefore, current studies are focused on generating alternatives to fabricate green energy. Numerous sources are required which are not only renewable but also environment friendly to develop sustainable carbon neutral fuels. sequestered from CO2 the atmosphere gets converted into biomass, and upon chemical conversion, into fuel. Therefore, green fuels do not contribute to any further addition or reduction of CO2 into the atmosphere and lead to carbon neutrality (Kumar et al., 2010). According to several scientific investigations, algae can capture 10–50 times more amount of CO2 leading to high growth rate (Mondal et al., 2017).
The major cost of fuel is dependent on the biomass which can either be crops like sugarcane or plant seeds but they possess competition with food crops and restrict product yield (first generation); lignocellulosic biomass, kitchen waste, crop residue but the high cost of processing due to lignin and expensive technologies for lipid extraction makes them unsuccessful (second generation); microalgae depict rapid growth and enhanced oil yield but it is still expensive for large scale production (third generation); genetically modified algae with advanced extraction techniques have recently been deployed for large scale production of biofuel but they also imply high initial investment and extensive research (fourth generation) (Yaashikaa et al., 2022). Amongst them, microalgae, chlorophyll harboring photosynthetic microorganism is the best candidate used for green fuel production (Gonçalves et al., 2013). They have the ability to enhance oil yield and marginalize dependence on fossil fuels. Also, they are capable to reduce greenhouse gases (GHG) emissions by 50–70% in comparison to conventional fuels (Liu et al., 2013). The major steps incorporated in the production of microalgae-derived biodiesel are cultivation, harvesting, extraction of oil, and purification followed by biodiesel synthesis from individual cells (Tang et al., 2020). Also, generate carbohydrates, proteins, vitamins, lipids, and pigments depending on species and external growth factors. The downstream processing accounts for 50% of the biodiesel production cost. Hence, novel methodologies are needed to reduce the fuel production cost.
Currently, hybrid models and by-product extractions have been employed with algae to make fuel economically viable. For instance, growing algae in a waste water treatment plant can not only reduce the cultivation cost, but also remove pollutants from it, recover nutrients, and generate high-value commodities (Chandrasekhar et al., 2022). More recently, algal industry has given attention to a diverse variety of algae-based products apart from lipids including carotenoids, proteins, nucleic acids etc., for the production of nutraceutical, cosmeceutical, bio-stimulants, bio-fertilizers, and other environment remediation commodities like biochar. Therefore, implementation of a biorefinery will elucidate the production of such high-value chemicals/products for different industrial interventions (Gondi et al., 2022). While, novel pre-treatment methodologies such as cell wall weakening before cell disintegration via nanoparticles may enhance the biogas as well as biofuel yield (Gondi et al., 2022) (Yukesh Kannah et al., 2021). Therefore, valorization of algal biomass for green fuel and using the leftover to extract other industrially important products can compensate the exorbitant fuel prices in the future.
The present article aims to provide an overview on the recent information on unit operations in up and downstream processing for algae-based biofuel as well as high-value chemical/product extraction. Additionally, a detailed study on the life cycle assessment (LCA) framework along with emerging techniques including genetic, metabolomic engineering coupled with omics approach for improving lipid yield and biomass productivity has been discussed. Finally, likelihood of developing an algal biorefinery for high-value products fabrication such as carbohydrates, lipids, carotenoids, amino acids, antioxidants, fatty acids, vitamins etc., for different industrial interventions has also been conferred. Moreover, present challenges and future research directions for integrated algal biorefinery setups are outlined.
Microalgae including Cyanophyceae (blue-green algae), Bacillariophyceae (diatoms), Chlorophyceae (green algae), and Chrysophyceae (golden algae) are the most economical and efficient for harboring lipid for biodiesel production, CO2 fixation and wastewater treatment (Kumar et al., 2010). Numerous bioprocess industries rely on these microorganisms’ metabolism to convert carbohydrates to other value-added products such as vitamins, amino acids and organic acids such as acetic acid. However, the cost of carbohydrate feedstock plays a significant role in the total cost of production in such industries. While photosynthetic organisms such as microalgae provide a production alternative and can entirely eliminate the cost associated with carbohydrate feedstock. They employ inexpensive and readily available sunlight as an energy source. Cyanobacteria are known to convert 3–9% of solar energy captured into biomass whereas terrestrial plants can convert only 0.25–3% of the captured solar energy into biomass (Ducat et al., 2011).
According to the scientific studies the strain of interest shall have the following features (Chu, 2017).
- Robust with enhanced growth rate and high lipid content.
- Capable to withstand shear stress generated during mixing and tolerate microorganism interference.
- Flexible to adapt according to diverse physiochemical parameters encountered during culturing.
- Genetically manipulable for obtaining high growth rate, lipid productivity, and other related bioproducts.
In order to obtain the above characteristics in the algal strain several techniques have been described for their isolation and screening including gravimetric separation, single-cell isolation by serial dilution, micromanipulation, and atomized cell spray (Mutanda et al., 2011). These conventional methods for strain selection are not only complex but also time-consuming, and labor-intensive (Kwak et al., 2016; Lim et al., 2017). In order to simplify the selection process, Kim et al. reported the advancements in the field of microfluidic systems to study microalgal biotechnology (Kim et al., 2017). Likewise, Challagulla et al. reviewed the applications of various technologies for the analysis of lipid content and biomass productivity of the microalgal cell. These technologies include raman spectroscopy, near-infrared spectroscopy, fluorescent lipid-soluble dye-based detection, nuclear magnetic resonance spectroscopy, and fourier transform techniques (Challagulla et al., 2016). Moreover, flow cytometry, microfluidics, and spectroscopy can either be used alone or in combination with other technologies for screening high lipid and biomass yielding microalgal strains (Shokravi et al., 2020). While there are several strategies for rapid selection of microalgal strains such as bioprospecting is the most prominent approach for screening high biomass producing strains. Also, high throughput techniques including vibrational spectroscopy or flow cytometry which are highly accurate and rapid for characterizing metabolites (Shokravi et al., 2020).
Solvent extraction and gravimetric methods are used for identifying high lipid yielding strains. These procedures are not only accurate but also provide reproducible results. However, they are time-consuming, labor intensive, and require a large amount (10–15 mg wet weight) of biomass (Morschett et al., 2016). On the contrary, chromatographic methods procure high reproducibility, require less time, and provide a single report with both qualitative and quantitative estimates of fatty acids. Such methods entail cell disruption to release cellular contents and derivatives of released fatty acids but they comprise highly expensive instrumentation (Dołowy and Pyka, 2015). Another technique is nile red staining, an in situ colorimetric detection technique for lipid estimation. Though it is simple, rapid, and highly efficient, still the accuracy of results can be influenced by several factors. Although, these methods are quick, cheap, and simple in nature but cannot detect fatty acid chains having less than 12 carbon atoms (Morschett et al., 2016). Time-domain nuclear magnetic resonance (TD-NMR) an in situ technique can be used for estimation as it is highly rapid, simple, and economical but the accuracy of this method depends on cellular lipid content. Therefore, it requires additional analytical equipment (Romel Malapascua, 2012). More recently, inverted fluorescence microscopy has been used for determining lipid content and growth for several microalgal species (Hathwaik and Cushman, 2017). Also, fluorescence-assisted cell sorting (FACS) has been employed for the detection of high lipid yielding cells in Chlorococcum littorale. Similarly, a microfluidic cytometer has been applied to quantify lipid accumulation and photosynthetic efficiency in Phaeodactylum tricornutum. While fourier-transform infrared spectroscopy (FTIR) has been used for examining both the lipid yield and growth in the case of Dunaliella salina (Romel Malapascua, 2012). Similarly, for Chlamydomonas reinhardtii mutants, droplet microfluidics-based screening has been applied for analyzing growth and lipid content (Hathwaik and Cushman, 2017).
In comparison to terrestrial plants, microalgae entail several advantages including a short life cycle from hours to days as compared to seasonal cycles seen in plants, are unicellular organisms that can rapidly breed in a confined space thus reducing the setup/land cost, and can do both sexual as well as asexual reproduction and boost the genetic diversity of the species. A variety of species can be screened on the basis of desired phenotypes via flow cytometry and other high throughput techniques. According to recent studies, transgenic microalgae are gaining more interest because of efficient phenotype construction (Hallmann, 2007). Novel strains can be developed by targeting specific genes through forward or reverse genetics. The former approach has much to offer by screening the results of random knockout events. Currently, there is a lack of transformation technologies that can be applied to biodiesel-producing algae. However, alternative strategies such as isolation and breeding of highly potent non-genetically modified organism (GMO) strains can be employed. One such method is the screening of libraries produced through UV or chemical mutagen treatment. This method also bypasses the regulatory procedure for GMOs for outdoor systems (Larkum et al., 2012).
Traditionally, open ponds and land-based photobioreactors (PBRs) have been used for algal cultivation. However, in recent times, the focus has been shifted to ocean-based floating microalgal culture systems due to the availability of large areas and wave energy for continuous mixing. In addition to this, seawater provides nutrients and impregnates thermoregulation (Park et al., 2018). However, there is still a need for the development of novel and advanced technologies for the harvesting of microalgae biomass, and lipids from the cells. So, to enhance lipid productivity, two-stage microalgal cultivation setups have been proposed 1) Efficient growth stage: either in culture medium or in wastewater effluent medium, and; 2) High lipid accumulation inducing stage: salt stress stage (Hang et al., 2020).
To date several attempts have been made to reduce the production cost of biodiesel, some of them are as follows: (Gong and Jiang, 2011):
- Sequester CO2 from thermal power plants to enhance biomass productivity
- Use nutrient-enriched wastewater for culturing microalgae
- Design and develop outdoor photobioreactors for large-scale algae cultivation
- Utilize by-products obtained after oil extraction effectively
- Incorporate genetic and metabolic engineering for the optimization of biodiesel production
Common strategies utilized for the cultivation of microalgae are: (Pavithra et al., 2020).
1) Single stage–Cultivation of microalgae using continuous, semi-continuous, or fed-batch system with lower lipid content due to nutrient deprivation in continuous or fed-batch setup.
2) Two-stage–Cultivation of microalgae in nutrient-rich medium followed by subjecting to stressful conditions.
The operation mode of a cultivation setup not only improves biomass but also lipid yield. Such cultivation setups comprise of artificial ponds, open water bodies, or containers. Besides, on the basis of architecture, they can be shallow raceways, and circular unstirred ponds, out of which, raceway ponds are the most widely implemented. Besides, raceway ponds provide continuous tender agitation, minimal energy requirements, and low cost. Usually, they are cemented/concrete construct divided by baffles arranged in a series to support gentle mixing of nutrients as well as algae biomass. A depth of 1.5 ft promotes efficient sunlight penetration and optimum CO2 sequestration (Chandra et al., 2020). However, they need an extremely large area and are capable of getting contaminated by microorganisms and birds. Moreover, the productivity quotient of microalgae cultivated in an open pond system is directly proportional to the location of the system, layout, inoculated species, and the prevailing weather conditions. For ponds with water levels higher than 200 mm, the light intensity for ponds, except for the thin upper layer, is below the photo-compensation point and thus, limiting the algal growth (Doucha and Lívanský, 2006). For such cases, thorough mixing can improve light penetration as well as cultivation efficiency. Additionally, such setups are majorly used for synthesizing, lipids, carotenoids (beta-carotene), proteins, carbohydrates and, other industrially important products. Though they are low-cost systems, but variable temperature, high water and weather dependence, and low biomass concentration limits their use for large-scale production.
PBRs are a closed artificial setup which can incorporate both mechanical as well as non-mechanical mixing strategies. Usually, they comprise of a sparger having specialized nozzles to release CO2 bubbles for non-mechanical agitation. While in some cases impellers can be installed for mechanical mixing in the PBR sets. These systems offer enhanced growth rates, higher cell density, and biomass compared to open pond cultivation systems (Huang et al., 2017). Additionally, CO2 sequestering PBRs have a high biomass transfer rate due to increased resistance to mass transfer from a gaseous phase to algal cells via the lipid phase. Moreover, such setups are composed of glass, polyvinyl chloride (PVC), polyethylene (PE), polycarbonate (PC), plexiglass, or fibre glass and provide appropriate growth conditions with desired amount of carbon, water, light, nutrients, temperature, and pH. Amongst the different bioreactor types, airlift reactors are the most suitable for sequestering flue CO2. Moreover, they provide uniform mixing, high gas transfer rate, low hydrodynamic stress, and an easy control system (Mondal et al., 2017). The selection of suitable PBR depends on the desired strain, space availability, and the preferred product’s nature. Specific advantages of using PBRs are 1) concentration monitoring and control system, 2) minimal or no chances of contamination, 3) no media evaporation, 4) high concentration of microalgal cells, and 5) provides several critical cultural conditions simultaneously such as pH, temperature, CO2, mixing and others (Pavithra et al., 2020). However, high capital and operational cost owing to complex configuration, an artificial illumination system, cooling, and agitation system, deoxygenation procedures, and other complex operating conditions are some of the limitations that prevent commercial implementation of these setups (Peng et al., 2020). Additionally, biofouling, overheating, and cellular damage due to shear stress are also some drawbacks. The PBRs are further classified into horizontal tubular, stirred tank, airlift, and bubble column PBR. Some advantages and disadvantages of these systems are discussed in Figure 1.
An effective PBR shall not only allow easy light penetration but also provide equal distribution of it. Besides it shall promote uniform mixing and mass transfer under favorable pH and temperature conditions. Also, cost is an important criterion for a long-term investment. So, it should be cost effective. Some of the aspects are discussed as follows.
Intensity of light and UV-Visible spectrum (400–700 nm) are some of the critical factors responsible for improved biomass yield. Usually, distribution of light is non-uniform inside a PBR due to scattering and absorption by individual cells (Quan et al., 2004). The radiation attenuation depends on cell concentration, PBR geometry, distance of light, and visible range. Also, according to studies intensity of light decreases with distance. So, light can penetrate only several millimetres when cell density is more than 10 g/L in a PBR. Additionally, growth decrease as the reactor depth increases. In some cases, investigators revealed that if light is above the critical value so the growth will be inhibited via photoinhibition (Richmond, 2004). On the contrary if it is below the necessary level then it will lead to photolimitation and stunned growth. Therefore, based on cell growth, PBR’s can be divided into 3 zones namely: strong illuminating zone, light energy fulfils the cell demand; weak illumination zone, light energy just meets the cell demand; and dark zone, cells are unable to grow due to limiting light (Bitog et al., 2011). Hence, for enhanced biomass productivity thickness of a PBR should be minimum.
Efficient mixing not only provides uniform distribution of nutrients, gas but also avoids stagnation of cells in dark zones, prevent fouling, and maintain the cultures (Anjos et al., 2013). Additionally, mixing exposes light equally to all the cells and endorse efficient mass transfer. But, excess of mixing might cause cell damage due to shear force. Also, it is recommended to keep the mixing velocity below 1 m/s to prevent micro eddies formation that can damage cells. A velocity of 20–50 cm/s is recommended for good mixing (Lehr & Posten, 2009). Mixing in PBR is induced via agitator, impeller, static mixer, or a CO2 enriched sparger.
Algae growth can be distributed into three processes including photosynthesis, photorespiration, and dark respiration (Wang et al., 2012). Most of the identified algal species undertake the C3 pathway or Calvin Cycle for CO2 fixation. An enzyme Ribulose-1,5-bisphosphate carboxylase/oxygenase (RuBisCo) catalyzes the formation of two molecules of the 3-carbon compound from the combination of CO2 and a 5-carbon containing compound. While, Photosynthesis is carried out in the chloroplast as a result of biophysical and biochemical reactions happening at thylakoid and stroma respectively. Biophysical reactions lead to photon absorption via chlorophyll and other accessory photosynthetic pigments. Therefore, water is oxidized and release oxygen. Free electrons from water molecules result in the formation of ATP and NADPH. The energy produced by the splitting of water is used for CO2 fixation in the dark reaction. Whereas, biochemical reactions lead to the formation of sugar and other organic molecules for cellular functions and metabolism (Raven and Beardall, 2003). Therefore, photosynthesis elucidates light and CO2 to make ATP and O2 as a by-product. However, increased amount of dissolved O2 may cause photoinhibition which is toxic and inhibit algae growth. So, closed PBR’s are equipped with a degasser to prevent such condition and mediate smooth mass transfer.
Microalgae are capable of growing only under specific temperature range. Exorbitant temperatures lead to growth inhibition followed by cell death. It is seen that Open pond cultivation maintain an approximate temperature of 40°C due to evaporation (Anto et al., 2020). While closed PBR are well equipped with thermal jackets and cooling water units which prevents desired temperature deviations. However, in tropical regions where the maximum temperature reaches upto 47°C there is a constant need of heat exchangers, IR-radiation, and light dilution is used to enhance biomass productivity. This additional cost can be compensated via solar photovoltaic panel setup (SPV) or other bioactive compounds extraction after biofuel production (Stephens et al., 2010) (Gondi et al., 2022).
Optimal pH for algae growth is in the range of 7–9. It greatly affects the CO2/carbonates solubility, and functioning of intracellular enzymes. Acidic media may enhance nutrition uptake by the cells, produce metabolites and increase metal toxicity. While a basic media interferes with the cell division. Any deviation from the standard pH range will reduce the photosynthetic efficacy. Generally, freshwater algae including cyanobacteria can tolerate altered pH, but marine algae are inflexible to any such deviations and need an optimum value around 8.2. (Lackner, 2003; Kumar et al., 2010).
Microalgal biodiesel is found to be a carbon-neutral renewable liquid fuel as half of the dry weight of the algal biomass is carbon which is sequestered from the atmosphere. Therefore, cultivation setups are continuously supplied with CO2 for enhanced biomass production. For instance, approximately 183 tons of CO2 can produce 100 tons of microalgal biomass (Chisti, 2008). Some sources of CO2 supply include atmospheric CO2 (approximately 0.0387% (v/v) in the air), industrial emissions (flue or flaring gases which typically contain approximately 15% (v/v) CO2 in the exhaust emission), and chemically fixed soluble carbonates (e.g., sodium bicarbonate and sodium carbonate). However, for large-scale setups, industrial emissions have been found to be adequately fulfilling the CO2 demands (Lackner, 2003; Kumar et al., 2010). Methods for supply and its efficacy vary with the nature of the cultivation system that is either open surface ponds or closed photobioreactors with diverse configurations. So, a closed setup is fortified with membrane transfer, gas injection, and gas exchange which offers fair CO2 supply, gas transfer, and uniform mixing of nutrients in the cultivation systems (Yamaguchi, 1996; Carvalho et al., 2006). According to the studies extracellular enzyme, carbonic anhydrase (CA)–a zinc metalloenzyme facilitates atmospheric CO2 uptake by microalgal cells. They are responsible for catalyzing the conversion of CO2 to bicarbonates. These bicarbonates can easily be taken up by the microalgal cells via specialized cell transporters. In addition to this, depending upon the microalgal species, captured CO2 can be stored in the cells as lipids, proteins, or carbohydrates. (Kumar et al., 2010; Mondal et al., 2017).
Microalgae cultivation setup defines fuel quality and quantity extracted from the feedstock (Pavithra et al., 2020). Under ideal cultivation conditions, the algal strain proliferates with a synchronous increase in the lipid content. However, the culture condition required for cellular growth differs from the one required for lipid production. Thus, the culture condition favors biomass production with a lower lipid content (Alishah Aratboni et al., 2019). In order to enhance the lipid content of the microalgal cells, other approaches must be employed, such as inducing nitrogen or phosphate limitation and increased salinity during growth. However, these conditions may reduce the cell growth rate. Therefore, a balance is required so that lipid content and cell growth occur (Qiu et al., 2017). A two-stage cultivation setup provides this balance for microalgae growth. Initially, a nutrient-rich medium is used to maximize biomass production in heterotrophic conditions, followed by inducing stress in the phototrophic mode for enhanced lipid productivity. The most commonly used two-stage strategies are based on: starvation, inducer addition, metabolic switch, and irradiation (Nagappan et al., 2019) (Yap et al., 2021).
There are two major culturing systems 1) Suspended cell cultivation and 2) Immobilized cell activation system. The suspended cell cultivation system is highly energy intensive and time-consuming. Circular and raceway ponds are preferred for commercial algae cultivation. The commercial ponds should be shallow, with a depth ranging between 0.2 and 0.5 m so that light can penetrate to the bottom of the pond. Microalgae absorb and utilize CO2 as a carbon source from the atmosphere. A suspended cell cultivation system has an effortless operation, low construction, operational and production cost, and functions under high CO2 concentration. The significant disadvantages of this cultivation method include a lack of light and temperature control system, and the entire system is prone to contamination. The second method, the immobilized cell activation system, is an alternative cell cultivation method. Biofilm containing microalgal cells are attached to their specific natural surface through effective adsorption, immobilization, semipermeable membrane capture, polymer traps, and covalent joint. Some widely used immobilization methods include cell adhesion, solid surface biofilm formation, and polymer matrix cell entrapment employing polymers such as alginate and carrageenan (Vasilieva et al., 2016; Lin-Lan et al., 2018).
Microalgal biomass harvesting contributes about 20–30% of the total production cost (Molina Grima et al., 2003). A detailed discussion on the advantages and disadvantages associated with different unit operations adopted in algal biofuels has been presented elsewhere (Kumar et al., 2022a). Since the harvested biomass is highly dilute and contains more than 99.6% of the water on a weight basis, dewatering is required for further downstream processing (Mata et al., 2012). Both harvesting and dewatering are bottlenecks for microalgae-based biofuels as they are energy intensive and incorporate the high cost of production (Peng et al., 2020). Furthermore, these methodologies are categorized as primary harvesting and secondary dewatering, as described in Figure 2.
After the synthesis and accumulation of lipids in microalgal cells, biomass is harvested, followed by lipid extraction and purification before transesterification reaction for biodiesel production. Numerous methods can be sequentially applied in the downstream process or directly to the bioreactors. Additionally, bulk harvesting is performed to separate algal biomass from the bulk suspension, accompanied by thickening protocols further to concentrate the slurry (Das, 2016). Harvesting algae cells are expensive due to their small size, which possesses terminal velocity and hinders mechanical separation. Also, these small-sized cells impart volume reduction and make the water removal process expensive. Algal cells are usually negatively charged, which keeps them in a dispersed state. Therefore, thermal drying and centrifugation can be incorporated to remove water. However, thermal drying is expensive, and centrifugation demands high energy making separation expensive. For economical separation, flocculation, sedimentation, and flotation can be performed before centrifugation or filtration (Jeevanandam and Danquah, 2020).
It is essential to release the lipid content produced inside the microalgal cell. Many frequently physical, chemical, biological, and mechanical cell disruption methods are incorporated for algal cells as described in Figure 3. Though algae cell walls are sometimes complex, varied chemically, and robust structurally so more energy intensive techniques are required to extract intercellular compounds which are referred as high-value products. Numerous techniques have been studied to break algal cell wall from a variety of species including the use of surfactants, ultrasound, microwave, sonication, bead milling, enzymatic lysis, high-pressure homogenization, and steam treatments (Alhattab et al., 2019). Some disruption techniques with advantages and disadvantages have been discussed below in Table 1.
Amongst all the extraction techniques available for the recovery of microalgal oil, an appropriate solvent should be selected to achieve enhanced yield and minimize the production cost. A comparative analysis of lipid extractions methods is depicted in Figure 4.
It is a widely applied method for microalgal oil extraction. It involves organic solvent selection on the basis of toxicity, recyclability, and cost. For instance, some common solvents are -hexane, benzene, acetone, cyclohexane, and chloroform. According to some recent studies, the use of Bligh and Dyer’s method which amalgamates a mixture of chloroform, methanol, and water to extract microalgal oil is the most efficient process for lipid extraction. Some studies have reported the use of the Folch method for lipid extraction which makes use of a 2:1 ratio of chloroform-methanol by volume. Currently, the Matyash method of lipid extraction is used which is comparatively more rigorous and is a modification to both of the methods. It employs methyl-tert-butyl ether (MTBE) as a solvent for lipid extraction (Kumar et al., 2015). However, their toxicity is a major concern that can be eradicated by employing cell disruption methods preceding the extraction step (Sati et al., 2019). To minimize the toxicity of chloroform, several other solvents such as ethanol, MTBE, hexane, and acetic acid esters can be employed. Recent studies have also suggested the employment of 2-ethoxyethanol (2-EE) for the enhanced extraction of lipids as compared to the traditionally used solvents (Kumar et al., 2015).
Supercritical fluids are compounds that act both as liquid or gas when exposed to varying temperatures and suppress above their critical values. These fluids are an alternative to organic solvents and are used for the extraction of lipids, essential oils, and other functional groups from plants and microalgae (Mata et al., 2012). Based on improved solvation powers of fluids such as CO2, water, ethane, methanol, and butane above their critical points these fluids have mass transfer properties similar to gases and solvating properties and diffusion coefficient comparable to that of liquids. Most commonly used is supercritical CO2 (scCO2) for oil extraction due to low critical temperature (31.1 OC) and pressure (72.9 atm). scCO2 is a green way of oil extraction and it also permits complete characterization of extracted lipid and the resultant biofuel. However, owing to low polarity it is relatively inefficient in the extraction of polar compounds. In order to enhance the extraction capability, modifiers or co-solvents are used. Modifiers are highly polar in nature and when added in small amounts can enhance the solvation properties of scCO2 (Mata et al., 2012). This technique offers several advantages over organic solvent extraction such as no toxicity levels, does not lead to oxidative degradation of extracts, and enabling easy separation of the bioproducts (Li et al., 2014).
Ionic liquids (ILs) are non-volatile, thermally stable, and have high solvation capabilities. They are a promising alternative for toxic and volatile organic solvents. ILs are also referred to as “green” solvents (Gholami et al., 2020). ILs are salts of large asymmetric cations coupled with smaller organic or inorganic anions. ILs can be maintained in a liquid state at temperatures ranging from 0 to 140°C. ILs are the non-aqueous solutions of desirable salts. IL-methanol system has been used as an alternative to imidazolium-based ILs as they are cheap and less toxic to aquatic habitat and thus, prove to be an environment-friendly method of oil extraction from microalgae (Sati et al., 2019). ILs make it possible to use varied combinations of anion and cation which in turn allows for deciding the polarity of the solvent, conductivity, hydrophobicity, and solubility (Kumar et al., 2015).
Microalgae store excess photosynthetic products as triacylglycerol, a storage lipid. Many microalgal species can be artificially induced to accumulate an increased amount of storage lipids under nutrient deficient conditions. These storage lipids are chemically similar to vegetable oils and can be extracted and converted to fatty acid methyl ester (FAME) for their use as biodiesel. Biodiesel has several advantages such as they are renewable, and biodegradable leading to the emission of fewer particulate matter into the environment upon its combustion (Dunahay et al., 1995). Extraction of lipid from biomass requires specific solvents having a high affinity for the microalgal lipids. Most commonly employed extraction methods for microalgal lipids involves the use of organic solvents, supercritical fluids, and ionic liquids (Amaro et al., 2011; Mercer and Armenta, 2011; Taher et al., 2011; Kim et al., 2012). Although these extraction techniques can be applied directly to the dewatered biomass, the efficiency is very less because the cell wall of microalgae prohibits these solvents from reaching the cytoplasm where these lipids are stored (Cravotto et al., 2008; Chen et al., 2009). Thus, in order to increase efficiency, lipid extraction is preceded by cell wall disruption methods (Gonçalves et al., 2013). Additionally, conversion of microalgal lipids to biodiesel is also done chemically by the following methods: 1) micro emulsification of oils; 2) direct use or blending of recovered oil; 3) transesterification/ alcoholysis; 4) thermochemical methods such as thermal cracking, pyrolysis, thermochemical liquefaction, gasification and; 5) biological conversion employing anaerobic digestion, fermentation, and photobiological oxygen production. Amongst all the known conversion methods, transesterification of microalgal oils is the most popularly employed methodology for oil separation (Suali and Sarbatly, 2012; Nandhini et al., 2022).
The most widely used multi-step process which converts triglycerides to low molecular weight alcohols in the presence of a catalyst (homogenous acid or alkali) is termed transesterification. This reaction is carried out in batch mode, stirred reactors. Initially, triglycerides are converted to diglycerides, monoglycerides, and then to glycerol. Such conversion reactions are reversible and each conversion step yields approximately 1 mol fatty acid ester (Quader and Ahmed, 2017). The process constitutes high conversion rates, easy process control, mild reaction temperature, and pressure. In addition to this molar ratio of alcohol, amount of free fatty acids (FFA), type of catalyst, reaction conditions and water content are the factors that affect biomass yield during the reaction (Chozhavendhan et al., 2020). However, prolonged reaction time, complex separation stage after reaction, and batch operation mode limits the use of this method extensively.
Moreover, this reaction is susceptible to the purity of feedstock (water content should be greater than 0.5% w/w and FFA content should be greater than 1% w/w). In order to obtain a pure product, some pre-treatment steps are incorporated with this method. This not only avoids low yield but also saponification. Transesterification can either be applied to the extracted microalgal oil or directly to the biomass, known as in situ transesterification. This can reduce the production cost by eliminating the cost involved in the extraction of microalgal oil (Chisti, 2008).
The multiple product recovery majorly depends on the fractioning ability of several components and maintenance of the quality and yield of the recovered products. The most crucial step is selecting an appropriate extraction technique and cell wall disruption to release the desired product. Additionally, optimal parameters and gentle extraction of different components manifest multiple product recovery (Gifuni et al., 2019). A large-scale microalgal culturing system currently produces extremely dilute cultures (0.08–3.6 g/L), and weather conditions and light penetration hamper their productivity. In order to minimize energy consumption in harvesting and extraction, a minimum of ∼100 g/L cell concentration is required (Gifuni et al., 2019). Microalgae possess an oil yield in the range of 58,700–136,900 L/ha. With 15–77% of oil on a dry basis, making them the best candidate for biodiesel production. The exact percentage of oil content is dependent on the algal species. The average cost of algal biodiesel is USD 0.48 to 2.8 per L, while the average price of conventional biodiesel produced from vegetable oil or palm oil is USD 0.52 per L. The significant expense of algae-based biodiesel depends on cultivation and harvesting techniques. In the current scenario, crude oil prices have exceeded USD 80–100 per 100 L. Therefore, microalgal biodiesel proves to be an economical and sustainable alternative.
To obtain microalgae-based fuel, species selection followed by elucidation of lipid metabolism and the connected pathway is crucial. In addition, comprehensive knowledge of carbon flux throughout cellular processes is required. Lipid catabolism plays an integral role in the metabolic processing of lipids throughout cell growth and during nutrition deficiency and is involved in lipid accumulation and homeostasis (Trentacoste et al., 2013). Analysis of various lipid classes, fatty acids, and membrane stability in transgenic strains suggested the role of lipase/phospholipase enzyme in maintaining lipid homeostasis and in membrane lipid turnover. Lipid catabolism provides acyl group for membrane recognition as a change in environmental conditions leads to the synthesis of polar lipids during dark cycles and remobilizes cell membrane when conditions get better. It is hypothesized that the knockdown of the lipase enzyme can lead to high lipid accumulation (Trentacoste et al., 2013). Understanding the microalgal lipid biosynthesis pathway paves the way to accumulate lipids in the microalgal cells, followed by the production of biodiesel and other bio-based products. In addition, genetic engineering, the addition of stimulators or repressors, or changing culture conditions may improve lipid storage inside the cells (Tang et al., 2020).
Numerous enzymes inducing biochemical reactions are responsible for synthesizing Triacylglycerol (TAG) and fatty acid (FA) in microalgae cells. It has been reported that over-expression of these enzymes would enhance lipid accumulation (Bajguz and Piotrowska-Niczyporuk, 2013). One of the most exploited enzymes is Acetyl-CoA carboxylase (ACCase), a multi-subunit enzyme found in the chloroplast of algae. According to some studies, overexpression of ACCase alone does not improve lipid accumulation (Ng et al., 2017). However, over-expression of ACCase subunit (accD) in association with malic enzyme (ME) increased lipid productivity in Dunaliella salina (Salama et al., 2014). Furthermore, overexpression of ME enhanced 2.5 times the lipid production in Phaeodactylum tricornutum without causing any negative impact on biomass productivity. Suppression of the competitive pathways such as lipid and carbohydrate catabolism are an alternative strategy to enhance oil yield (Abomohra et al., 2020). Carbohydrate metabolism is an essential microalgal pathway for storing carbon and producing starch. Therefore, the knockdown of starch metabolism may alter the carbon pathway toward lipid synthesis.
TAG accumulation can remarkably be enhanced by introducing environmental stress such as nitrogen scarcity. Limiting iron, sulfur, zinc, phosphorus, and salt may also trigger lipid and starch synthesis in microalgae. Major steps involved in lipid biosynthesis are: 1) fatty acid production in plastids; 2) glycerol-lipid formation in the endoplasmic reticulum (ER); 3) packaging of glycerol-lipids into oil bodies. Fatty acid synthesis is catalyzed through ACCase, yielding malonyl-coenzyme A from acetyl-coenzyme A and bicarbonate (Maity et al., 2014). ACCase through its two catalytic centers biotin carboxylase (BC) and carboxyl transferase (CT), catalyzes irreversible carboxylation of acetyl-CoA to produce malonyl-CoA, which is essential for fatty acid biosynthesis (Cronan and Waldrop, 2002; Fan et al., 2014).
Microalgal biomass is initially produced under nitrogen-rich conditions, while TAG accumulation is induced by creating nitrogen deficiency (Peng et al., 2020). Nitrogen limitation reduces metabolism and leads to excess photon dissipation as heat or fluorescence. The percentage reduction in photosynthetic capacity under stressful situations is species-specific and influences the amount of available energy for the synthesis of TAG. Also, it causes a reduction in photosynthetic proteins, pigments, and centers for photosystem reaction in order to minimize overexcitation-associated damage (Chisti, 2008; Kumar et al., 2010). For instance, in Neochloris oleoabundans only 8.6% of the generated electrons were used for TAG biosynthesis and the rest of the energy got dissipated in photosystems or utilized in catabolic processes (Trentacoste et al., 2013). Thus, it is highly recommended to choose a species that retains the high photosynthetic capability and TAG productivity under nitrogen depletion (Klok et al., 2014). TAG content can further be improved by employing techniques such as selective cell sorting, targeted engineering, and random mutagenesis (Remmers et al., 2018). It has been studied that the efficiency of bulk lipid production is less due to low solar-lipid conversion. Several attempts have been made to increase solar-lipid conversion efficiencies such as rational strain selection, improvement of selected strain, and process condition optimization (Yamaguchi, 1996; Carvalho et al., 2006; Mondal et al., 2017).
A variety of enzymes including acetyl-CoA synthetase (ACS), Acetyl-CoA carboxylase (ACCase), acyl-ACP esterase (AAE), diacylglycerol acyltransferase (DGAT), D5-elongase (D5 Elo), D6-elongase (D6 Elo), glycerol-3-phosphate-dehydrogenase (G3PDH), glycerol-3-phosphate acyltransferase (GPAT), lysophosphatidic acid acyltransferase (LPAT), phosphatidic acid phosphatase (PAP) is involved in lipid biosynthesis (Klok et al., 2014). According to some experimental pieces of evidence, overexpression of cytosolic ACCSase in Chlamydomonas reinhardtii lead to a 2-fold increase in starch content and a 2.4-fold higher accumulation of TAG (Rengel et al., 2018). Similarly, overexpression of Acetyl-CoA carboxylase (ACCase) in Chlamydomonas reinhardtii increased FA synthesis by approximately 56%. It is highly possible that the homeostatic regulation as in the case of redox activation of ACCase under stressful conditions ensures sufficient synthesis of fatty acyl groups (Shahid et al., 2020). Overexpression of AAE, led to fatty acid profile changes in Phaeodactylum tricornutum and Chlamydomonas reinhardtii whereas an increased lipid content was observed only in P. tricornutum (Dunahay et al., 1995; Lin et al., 2018). While, overexpression of type 2 DGAT in P. tricornutum leads to only 35% increase in TAG levels (Muñoz et al., 2019) and overexpression of some type 2 DGAT enzymes results in an increased TAG yield in C. reinhardtii (Xue et al., 2015; Sharma et al., 2021). In Chlorella minutisma, it has been studied that heterologous expression of yeast-derived LPAT, PAP, GPAT, DGAT, and G3PDH enzymes leads to a two-fold increase in TAG content as compared to overexpression of any one of the genes (Han et al., 2021). Heterologous expression of gene D5 Elo and D6 Elo from Ostreococcus tauri into P. tricornutum resulted in an increase of docosahexaenoic acid (DHA) (Klok et al., 2014).
Numerous biological barriers are still present for industrial-scale algal biodiesel production have been discussed. Figure 5 describes various upstream and downstream strategies to enhance microalgal biomass production.
- Organism survival: Current systems have been unable to maintain the best laboratory organisms under field conditions. Laboratory culture when exposed to environmental conditions gets contaminated by indigenous organisms found in the environment (Gressel, 2007).
- Carbon dioxide enrichment: Carbon dioxide levels above 5% slow the growth of higher plants and animals, yet many algae and cyanobacteria grow happily when “aerated” with 100% carbon dioxide or directly with ca. 14% CO2 flue gas from power generators. Still, the response of algae to added carbon dioxide is not as good as it could be, and there is a place for improvement of carbon assimilation (Gressel, 2007).
- Light penetration: For autotrophic cultivation of algae light is an important parameter. Its intensity decreases with the increase of water depth. Moreover, the photosynthetic efficacy of the species is highly dependent on the source of light. In open ponds, the shallow depths provide a high surface area with efficient mixing and light penetration. It can be calculated by Beer-Lambert’s law (Wang et al., 2015).
- Environmental dependence: The high yields attained in ponds is usually seasonal. Therefore, algal growth is a function of temperature. Some species do not grow well in cold temperatures while some face difficulty at high temperatures. Additionally, they require a special cooling unit (Gressel, 2007).
- Limitations: According to the literature, a major drawback of algae-based biodiesel production is the display of contrasting features such as high biomass productivity accompanied by low lipid accumulation, or high lipid accumulation with low biomass productivity. The growth rate of oleaginous algal species is often slow (Shokravi et al., 2020). It is well established that in order to produce biodiesel economically, a balance is required to be maintained between biomass productivity and lipid accumulation.
- Challenges associated with biomass production:
1) Daily and seasonal variations of sunlight during cultivation.
2) High levels of dissolved oxygen in closed PBRs inhibit photosynthesis in microalgal cells.
3) High oxygen concentration and sunlight intensity cause photo-oxidative damage to microalgal cells.
The maximum tolerance of dissolved oxygen for microalgal cells should not exceed 400% of air saturation in PBRs (Chisti, 2008).
The microalgal lipids can be classified into two major groups: polar lipids (phospholipids and glycolipids) which constitute about 41–92% of the total lipid content and non-polar or neutral lipids (sterols and free fatty acids) having 5–51% of lipid content (Tang et al., 2020). Genetic and metabolic engineering can (Chisti, 2008) enhances the photosynthetic efficiency and increase the biomass yield on light, increase biomass growth rate, elevate the oil content in algal biomass, and improve temperature tolerance of algae so that there is a reduced need for cooling consequently reduces the cost of production, and provide tolerance against photoinhibition. Therefore, elucidating the genetic makeup can improve the strain yield.
Increased levels of polar lipids inside the cell may lead to a reduction in permeability of membranes and also lead to reduced fluidity which limits an excessive flow of sodium and chloride ions into cells. A high concentration of neutral/non-polar lipids enables microalgal cells to resist osmotic pressure by enhancing membrane rigidity. The addition of salts such as sodium bicarbonate increases the lipid content of cells by enhancing the photosynthetic capability and nitrogen utilization by microalgal cells (White et al., 2013). While, ferric, cupric, zinc and chromium salts, have been reported to increase lipid content in some of the algal species (Sharma et al., 2012). However, these salts cannot be used on a large scale due to their extreme harmful environmental impact. Sodium chloride supplementation can be used more effectively and safely for enhancing lipid accumulation in microalgal cells (Xia et al., 2013). Table 2 describes various attempts to enhance algal productivity by employing various abiotic strategies.
In recent times, researchers have proposed a number of strategies for increasing lipid content in microalgal cells such as genome manipulation, random mutagenesis, metabolic engineering, blocking carbohydrate metabolism pathways, and lipid degradation which competes with the biosynthesis of lipids. As a result, engineered microalgal strains have high lipid content but slow growth rates. Amongst all the devised strategies, blocking competitive pathways has been recognized as a potential alternative to increase lipid yield (Sun et al., 2019). Researchers have suggested that inhibition of enzymes responsible for converting lipids into free fatty acids may help in improving lipid accumulation. Lipid catabolism provides acyl groups that help in membrane reorganization and acknowledgment of the photosynthetic system. Some recent studies depicted that knockdown of genes associated with lipid catabolism has a lesser impact on the microalgal growth rate as compared to the strains having disrupted carbohydrate pools. Therefore, lipid catabolizing enzymes are the best candidates for improving lipid content through knockout mutations (Nguyen et al., 2020). Table 3 describes various examples of genetic and metabolic engineering that have been carried out to enhance algal lipid productivity.
Metabolic engineering involves the addition or deletion of genes in order to optimize the genetic and regulatory processes of an organism for altering metabolic properties in a predetermined way such as to increase the production of certain metabolites in an organism (Sun et al., 2019). DGAT enzyme involved in TAGs biosynthesis is one of the most crucial enzymes in the pathway as it catalyzes the last step of the lipid synthesis pathway which is the esterification of diacylglycerol yielding triacylglycerol. Overexpression of DGAT can lead to high lipid productivity in GM microalgal cells. Alternative strategies for enhancing microalgal lipid accumulation focus on blocking metabolic pathways competing with lipid production such as starch synthesis, oxaloacetate, or phospholipid biosynthesis pathways. Till now more than 40 microalgal species have been genetically manipulated expressing different stability and efficiencies (Díaz-Santos, 2019).
Enzymes such as glycerol-3-phosphate acyltransferase (GPAT), lysophosphatidic acid acyltransferase (LPAT), and diacylglycerol acyltransferase (DGAT) of the Kennedy pathway are responsible for catalyzing key steps in the formation of TAGs in algal cells. Overexpression of these key enzymes in strains may increase TAG contents in the cells (Muñoz et al., 2019). Overexpression of malic enzyme (ME) also leads an enhanced lipid production in the microalgal cells as ME catalyzes the irreversible oxidative decarboxylation of malate to yield pyruvate, NADH, and CO2. NADH is required as reducing power for fatty acid biosynthesis. Morphological changes have also been observed in transgenic algae with shorter and thicker cells containing large oil bodies (Xue et al., 2015).
Despite all the ideal properties of microalgae, the use of genetic engineering and synthetic biology for genome modification is still a challenge. Genome manipulation helps in improving microalgal strains followed by enhanced biomass yield, productivity, and efficiency of microalgal-based industries. Recently, Clustered Regularly Interspaced Short Palindromic Repeats (CRISPR/Cas9), an advanced genome manipulation technique is gaining importance in manipulating microalgal cells for improved atmospheric CO2 fixation which eventually would lead to efficient biofuel production. This approach is highly efficient, economical, and environment friendly too (Godbole et al., 2021). The recent methods of genome editing and advancements in metabolic engineering and synthetic biology enable us to combat issues associated with traditional genetic engineering approaches such as instability of transgenes, ineffective expression, and delivery system for effective delivery of genetic material exogenous in nature and selection and screening of recombinants (Sun et al., 2019).
The current strategies employed to improve lipid biosynthesis or to modify the fatty acid profile of microalgal cells includes: 1) fatty acid beta-oxidation elimination, 2) increment in reducing power supply, 3) use of plant-based thioesterase for optimizing fatty acid chain length and 4) thioesterase overexpression to minimize feedback inhibition due to increased levels of acyl-ACP. Genetic engineering tools such as Zinc finger nucleases, CRISPR/Cas9, and chloroplast transfusion should be preferred for overexpressing and blocking the genes of interest (Shahid et al., 2020).
Genetic engineering so far has been applied to the following aspects to increase lipid content in microalgal cells:
1) Overexpressing enzymes responsible for lipid biosynthesis
2) Blocking or inhibiting competitive pathways such as inhibiting beta-oxidation, inhibiting starch synthesis, suppressing the activity of phosphoenolpyruvate carboxylase, and repression of phospholipid biosynthesis.
3) Constitutive expression of lipid biosynthesis transcription regulators in microalgal cells
4) Metabolic engineering to enhance lipid productivity (Morales et al., 2021).
Algae and cyanobacteria for third-generation biodiesel need transgenic manipulation to deal with “weeds”, light penetration, photoinhibition, carbon assimilation, etc. In order to scale up microalgal production industrially, less than 6 million hectares of land which is less than 0.4% of arable land is required across the world for fulfilling the current demands of fuel (Popp et al., 2014). Recombinant DNA technology can be used to introduce the gene of interest into the host cells through a transformation in order to manipulate physiological pathways or to produce commercially viable compounds. Currently, genetic manipulation of microalgae depends on random mutagenesis accompanied by screening and selection (Godbole et al., 2021).
Cyclotella cryptica and Navicula saprophila, two diatoms were genetically modified by E. coli neomycin phosphotransferase gene IZ (nptll) gene. Expression of nptll gene of bacterial origin in diatoms was done by using promoter and terminator sequences of ACC gene from Cyclotella cryptica T13L Reimann, Lewin and Guillard. Microprojectile bombardment was used for the introduction of vectors into Cyclotella cryptica and Navicula saprophila NAVICI L ange-Bertalot and Bonik. Putative transformants were screened and selected G418, and production of neomycin phosphor- based on their ability to grow in the presence of anti-transferase protein by transformed cells was confirmed by western blotting. It was observed that the foreign DNA got integrated into one or more random sites of the transformed algal cells, often in form of tandem repeats. This study was the first study on the reproducible, stable genetic transformation of a chlorophyll-c harboring alga (Dunahay et al., 1995).
In recent studies, it has been demonstrated that genetically engineered cyanobacteria have enhanced carbon-concentrating mechanisms (CCMs) which enables them to secrete heterologous carbonic anhydrases (CAs) (Dunahay et al., 1995). Genetic manipulations in eukaryotic microalgae are difficult due to the lack of stability in the expression of transgenes, the presence of highly efficient endogenous promotors, and the absence of complete genetic information (Lin et al., 2018). Researchers have demonstrated that CGI-58 homolog knockdown in Thalassiosira pseudonana resulted in increased polar lipid concentrations and membrane integrity during silicon starvation. This study elucidated the role of the CGI-58 enzyme in the turnover of the membrane during nutrient starvation-induced lipid accumulation. This enzyme is also beneficial for the conversion processes such as hydrothermal liquefaction. Similarly, CGI-58 homolog knockdown in Arabidopsis thaliana resulted in an increased concentration of polar lipids (Muñoz et al., 2019). It has been demonstrated recently that although about 60% of the accumulated TAGs under nutrient-deficient conditions are derived from de novo lipid synthesis pathways in the case of Phaeodactylum tricornatum, a considerable amount of phospholipids turnover also occurs (Xue et al., 2015). Scientists have also proposed a secondary TAG biosynthesis route involving turnover of intracellular membrane employing the enzyme phospholipid diacylglycerol acyltransferase (Han et al., 2021; Sharma et al., 2021). SYTOX staining results indicate that phospholipids of the plasma membrane may also act as a source of acyl groups required for TAG biosynthesis and lipid remodeling may also occur during lipid accumulation (Trentacoste et al., 2013).
Agrobacterium tumefaciens- mediated transformation has shown stability in the case of Chlorella sorokiniana as compared to other transformation methods which demonstrate transient stability (Sharma et al., 2021). Overproduction of docosahexaenoic acid (of oxidative defense pathway) by means of genetic engineering in Schizochytrium sp. is known to promote DHA production, lipid yield, and cell robustness. Attempts have been made to enhance the oxidative stress defense system of Schizochytrium sp. to reduce or lower lipids and DHA oxidation (Han et al., 2021). There have been several attempts to express sequences encoding GPDH, GPAT, LPAT, PAP, DGAT, and PDAT, which can prove to be helpful in the manipulation of metabolic pathways leading to biodiesel production. These methods have been reported to increase storage lipid content by two-fold in Chlorella minutissma UTEX 2219 as compared to wild strains (Hsieh et al., 2012).
In recent times, there has been a switch towards genetic engineering approaches to escalate lipid and biomass productivity. The possibilities of non-GM approaches should not be forgotten due to strict regulatory procedures over GMOs, environmental safety concerns, commercial, and ethical constraints associated with GMOs. One of the major challenges associated with the use of GM microalgal strains is containment. GM strains must be prevented from their loss into the environment and to mitigate unknown consequences associated with GM strains such as the risk of transgene flow through horizontal gene transfer (HGT) to other species and loss of GM strains caused due to predation or competition with wild strains while mass culturing (Driver et al., 2014).
A biorefinery is established to produce biofuels and a variety of chemicals from algal biomass via bioprocessing and other cost-effective methodologies. It can utilize low energy and produce zero waste. Algal biomass can not only harness biofuel but also other economically viable products including pigments, polyhydroxybutyrate (PHB), and starch which can be used for different interventions (Chandrasekhar et al., 2022). Additionally, investigators have identified the bio-remedial properties of algae. Their capturing capability of heavy metals and toxic chemicals from wastewater makes them a great fit for bioremediation. Algae-based biorefinery has the potential to incorporate numerous technologies to produce green fuel including biodiesel, bioethanol, gasoline, methane, and other high-value compounds like fats, eicosatetraenoic acid and docosahexaenoic acid, dyes/pigments (β-carotene/lutein/astaxanthin), antioxidants and carbohydrates. Theoretically, biorefinery would cultivate algae on a farm followed by biodiesel, bio-oil, bioethanol, biomethane, and biohydrogen extraction from the harvested biomass. While, the leftover biomass can be processed to harness other value-added products including livestock feed, organic fertilizer due to the presence of high N:P ratio, food additives, bio-adsorbents, growth regulators, and bioactive compounds for pharmaceutical industries (Chugh et al., 2022; Wang et al., 2008; Karpanai Selvan et al., 2022). Some of the biorefinery-based products are discussed in Table 4 below.
Modern agriculture is totally dependent on the use of fertilizers for the desired growth. Therefore, extensive research is going on in this field to develop sustainable fertilizers that are non-toxic to the environment. According to some recent investigations, a variety of algae species act as bio-stimulants and enhance crop yield (Win et al., 2018; Abusweireh et al., 2022). Blue-green algae (cyanobacteria) are the most promising source due to their nitrogen metabolizing capability. Additionally, they solubilize phosphates and fix nitrogen anaerobically (Chittora et al., 2020). The nitrogen fixation mechanism is switched on during the deprived conditions that are below 40 ppm. Besides, reduced fertility along with rice-mustard-mung crop rotation was preferable for cyanobacteria-based nitrogen fixation in comparison to rice-wheat-maize rotation (Jha et al., 2001). Recently, Chlorella variabilis and Lyngbya majuscula were investigated as potential biofertilizers for Zea mays L. The pot experiments were successful in reproducing recommended rate for fertilizer by algae-based biofertilizer which was nearly 65.16 g per plant.
Nano-based products have dimensions in the range of 1–100 nm and are classified as natural, incidental, or engineered. Due to the presence of hydrophilic surface groups, such as sulfate, carboxyl, and hydroxyl nano-based materials are successful in the fabrication of products possessing antioxidant, antimicrobial, antibacterial, cell imaging, and dye degradation properties. Parameters include pH, temperature, incubation time, intensity of light, and precursor effects the synthesis rate (Rahman et al., 2020; Kumar et al., 2022b). For instance, Acutodesmus dimorphus synthesized silver nanoparticles depict 79% ROS scavenging at 25 μg/ml concentration making it antioxidant (Chokshi et al., 2016). Similarly, Gracilaria corticata based ZnSe nanoparticles showed 77.9% oxidant activity. Additionally, ZnSe NPs possess an anticancer effect on the lung as well as breast cell lines (Mirzaei et al., 2021). Trichodesmium erythraeum was also studied to depict 77.1% of antioxidant activity. Most of these algae-derived NPs are successful against bacteria. Such as, ZnSe NPs elucidated antibacterial effect on S. aureus (gram-positive) and K. pneumoniae (gram-negative) bacteria. Similarly, Botryococcus braunii extract was also effective against S. aureus and K. pneumonia. While Chlorella sp.-based nanoparticles have been inhibiting E. coli, Klebsiella pneumonia, B. sphaericus, and P. mirabilis respectively (Mirzaei et al., 202; Borah et al., 2020; Kashyap et al., 2019; Sathishkumar et al., 2019). Moreover, AgNPs can do dye degradation and doped NPs can be of use in imaging root and guard cells (Zhang et al., 2017; Borah et al., 2020). Therefore, nanotechnology has the ability to not only revolutionize human health but also offer detection and disease prevention products. Some of the applications of nanoparticles have been discussed in Table 5 below.
Biochar is defined as a carbon enriched material usually produced via thermochemical decomposition of biomass at extremely high temperatures (500–1,000°C) in an O2 deficient environment. It is prominently produced from the leftover biomass after bio-oil extraction. Recently, different strategies are developed to produce biochar including conventional techniques like slow and microwave-assisted pyrolysis as well as some newly developed methodologies like hydrothermal carbonization and torrefaction (Yu et al., 2017). It is charcoal that can adsorb heavy metal impurities from wastewater, pharmaceutical metabolites, phenols, and chemical intermediates, perform dye degradation, reduce soil erosion as well as improve crop yield. Due to high nutrition content and ion-exchange capacity, they are successful in replenishing soil (Karthik et al., 2021). Moreover, recent investigations reveal that biochar can enhance soil productivity, prevent greenhouse gas emissions and enhance the degradation speed of organic matter in the soil making it an ideal solution for composting (Wu et al., 2019). More recently, N-doped biochar has been utilized as an energy absorbent and promotes energy conservation. Super capacitors, batteries, fuel cells, and biochar-based composites are a successful result of this N-type doping (Xia et al., 2020). Therefore, for over a decade the algae-derived biochar can be of great importance in energy storage as well as carbon sequestration.
Carotenoids are the bioactive compounds extracted from algal biomass and are of great importance for drug preparation. According to the investigators, pigments including β-carotene, lutein, fucoxanthin, violaxanthin, astaxanthin, and phycobiliproteins possess antitumor potential. Besides, they are also a promising source of antioxidants. For instance, Haematococcus pluvialis extracted astaxanthin provides UV protection, improves immune response, and reduces tumor formation as well as inflammation (Mota et al., 2022). Phycocyanin obtained from Spirulina possesses fluorescence and is utilized for labeling antibodies, receptors, and other biological molecules for diagnostics. Numerous brown algae including Wakame, Kombu, Mozuki are a rich source of fucoxanthin which has anti-cancer as well as anti-obesity effects. It also improves lipid metabolism (Das, 2016). Additionally, algae machinery can be used for recombinant protein production due to the easy transformation of both plastids as well as nuclear genomes and offers advantages such as cheap production, easy scale-up, lack of pathogens, and easy assembling of complex proteins (Rosales-Mendoza et al., 2012). Hence, chloroplast can be a site for antibiotics fabrication (Purton et al., 2013). The pure algal extract also possesses some antimicrobial effects on gram-positive and gram-negative species such as Staphylococcus aureus, Bacillus subtilis, Pseudomonas aeruginosa, Salmonella typhimurium, and Proteus mirabilis (Soltani et al., 2011).
Apart from biodiesel, bioethanol, biohydrogen, biomethane, and bio-oil synthesis leftover biomass can be used to reproduce products including food, feed, natural colors, and polymers. Microalgae such as Spirulina, Chlorella, Scenedesmus, Dunaliella salina, and Aphanizomenon flos-aquae have promising nutrients for not only humans but also animals. For instance, Chlorella pyrenoidosa comprise of 65% protein, 5–10% lipid, 10–20% hydrocarbons, 200–500 mg kg/L vitamin C, 120–300 mg kg/L of vitamin A and antioxidants (Shen et al., 2008). Dunaliella-based proteins are used in the baking industry while its biomass is utilized for fish and animal feed (Das, 2016). Several vitamins (vitamins A, B1, B2, B6, B12, C, E, nioctinate, folic acid, and pantothenic acid) and minerals are also enriched in species (Koyande et al., 2019). Some freshwater species have a higher prebiotic index and modulate intestinal microbiota by increasing Lactobacillus–Enterococcus and Bifidobacterium (desired bacteria) while inhibiting Prevotellaceae-Bacteroidaceae, Clostridium histolyticum, and Eubacterium rectale Clostridium coccoides (undesired bacteria) (de Medeiros et al., 2021). Algae-derived protein not only has essential amino acids (isoleucine, valine, lysine, tryptophan, methionine, threonine, and histidine) but is higher than conventional sources such as meat, fish, and dairy products. For instance, 7 g dried biomass of Spirulina maxima contains 4 g of protein, 1 g of fat (omega-3 and omega-6 fatty acids) 11% Vitamin B1, 15% vitamin B2 and 4% vitamin B3, 21% copper, and 11% iron of required daily allowance (RDA) (Milledge, 2011). While, magnesium, manganese and potassium are reported in trace amounts (Christaki et al., 2011). The inclusion of microalgae biomass in the animal feedstock can improve meat quality in ruminants, pigs, poultry, and rabbits (Madeira et al., 2017). For example, the addition of Arthrospira platensis in poultry diets increases average daily gain but negatively affects the feed conversion ratio. Similarly, Schizochytrium sp., improve fatty acid composition in poultry animals, due to the presence of a high amount of DHA. Therefore, adding algae biomass into the feed can be a cost-effective measure of fuel production. Due to the presence of chlorophylls, carotenoids, xanthophylls, and phycobiliproteins in several algal species natural colors can be harnessed as additives in ice-creams, candies, non-alcoholic beverages, and dietary foods as well as clothes (Azeem et al., 2019) (Das, 2016). According to some recent studies, species of algae are also capable of producing bioplastic which can be harnessed to produce numerous products including biodegradable plastic bags, slippers, buckets, etc. Additionally, red algae can produce some gelling polysaccharides like agar, agarose, and carrageenan possessing sulphated galactans. For instance, Hydropuntia cornea-produced agar can be blended at different concentrations with polyvinyl alcohol to produce biodegradable films for the packaging industry (Das, 2016). Therefore, numerous applications of algal species are possible by implementing a biorefinery.
Over the decade, genetic and metabolomic engineering coupled with omics has played a prominent role in improving lipid yield. While, more recently integrating biomass-based biorefinery with a waste-water treatment setup has proved to reduce the production cost of algae-based fuel. However, more innovation is required to overcome the issues of strain selection, cultivation, harvesting, drying and fuel conversion methodologies. Additionally, environmental risk assessment, resource organization, and life cycle assessment (LCA) of algal strain to biofuels is required. More recently biorefineries have been established that not only helps to produce biofuel but also several high-value-low-yield biomolecules for pharmaceutical and nutraceutical interventions. Additionally, several environmentally important products such as biofertilizers, biochar, bio-stimulants, nanomaterials, etc., are also generated. However, research on various aspects of algal-based bioactive compound extraction is in nascent phase and requires bioprospecting of high yielding native algal species, development and deployment of mass cultivation strategies, process optimization for harvest and cell disruption techniques followed by efficient biomolecule extraction procedures to make algal biorefinery sustainable and commercially viable in nature.
LK, LM, and RA- wrote the original draft, Concept. VJ and MC- Review and edited the manuscript. NB- Concept, and Supervise.
The authors declare that the research was conducted in the absence of any commercial or financial relationships that could be construed as a potential conflict of interest.
All claims expressed in this article are solely those of the authors and do not necessarily represent those of their affiliated organizations, or those of the publisher, the editors and the reviewers. Any product that may be evaluated in this article, or claim that may be made by its manufacturer, is not guaranteed or endorsed by the publisher.
Abomohra, A. E.-F., El-Naggar, A. H., Alaswad, S. O., Elsayed, M., Li, M., and Li, W. (2020). Enhancement of biodiesel yield from a halophilic green microalga isolated under extreme hypersaline conditions through stepwise salinity adaptation strategy. Bioresour. Technol. 310, 123462. doi:10.1016/j.biortech.2020.123462
Abusweireh, R. S., Rajamohan, N., and Vasseghian, Y. (2022). Enhanced production of biodiesel using nanomaterials: A detailed review on the mechanism and influencing factors. Fuel 319, 123862. doi:10.1016/J.FUEL.2022.123862
Alhattab, M., Kermanshahi-Pour, A., and Brooks, M. S. L. (2019). Microalgae disruption techniques for product recovery: Influence of cell wall composition. J. Appl. Phycol. 31, 61–88. doi:10.1007/s10811-018-1560-9
Alishah Aratboni, H., Rafiei, N., Garcia-Granados, R., Alemzadeh, A., and Morones-Ramírez, J. R. (2019). Biomass and lipid induction strategies in microalgae for biofuel production and other applications. Microb. Cell Fact. 18, 178–195. doi:10.1186/s12934-019-1228-4
Amaro, H. M., Guedes, A. C., and Malcata, F. X. (2011). Advances and perspectives in using microalgae to produce biodiesel. Appl. Energy 88, 3402–3410. doi:10.1016/J.APENERGY.2010.12.014
Angulo, E., Bula, L., Mercado, I., Montaño, A., and Cubillán, N. (2018). Bioremediation of Cephalexin with non-living Chlorella sp., biomass after lipid extraction. Bioresour. Technol. 257, 17–22. doi:10.1016/J.BIORTECH.2018.02.079
Anjos, M., Fernandes, B. D., Vicente, A. A., Teixeira, J. A., and Dragone, G. (2013). Optimization of CO2 bio-mitigation by Chlorella vulgaris. Bioresour. Technol. 139, 149–154. doi:10.1016/j.biortech.2013.04.032
Anto, S., Mukherjee, S. S., Muthappa, R., Mathimani, T., Deviram, G., Kumar, S. S., et al. (2020). Algae as green energy reserve: Technological outlook on biofuel production. Chemosphere 242, 125079. doi:10.1016/j.chemosphere.2019.125079
Arya, A., Gupta, K., Chundawat, T. S., and Vaya, D. (2018). Biogenic synthesis of copper and silver nanoparticles using green alga Botryococcus braunii and its antimicrobial activity. Bioinorganic Chem. Appl., 1–9. doi:10.1155/2018/7879403
Azeem, M., Iqbal, N., Mir, R. A., Adeel, S., Batool, F., Khan, A. A., et al. (2019). Harnessing natural colorants from algal species for fabric dyeing: A sustainable eco-friendly approach for textile processing. J. Appl. Phycol. 31. doi:10.1007/s10811-019-01848-z
Bajguz, A., and Piotrowska-Niczyporuk, A. (2013). Synergistic effect of auxins and brassinosteroids on the growth and regulation of metabolite content in the green alga Chlorella vulgaris (Trebouxiophyceae). Plant Physiol. biochem. 71, 290–297. doi:10.1016/J.PLAPHY.2013.08.003
Bitog, J. P., Lee, I. B., Lee, C. G., Kim, K. S., Hwang, H. S., Hong, S. W., et al. (2011). Application of computational fluid dynamics for modeling and designing photobioreactors for microalgae production: A review. Comput. Electron. Agric. 76, 131–147. doi:10.1016/J.COMPAG.2011.01.015
Bohutskyi, P., Ketter, B., Chow, S., Adams, K. J., Betenbaugh, M. J., Allnutt, F. C. T., et al. (2015). Anaerobic digestion of lipid-extracted Auxenochlorella protothecoides biomass for methane generation and nutrient recovery. Bioresour. Technol. 183, 229–239. doi:10.1016/J.BIORTECH.2015.02.012
Bohutskyi, P., Phan, D., Spierling, R. E., Kopachevsky, A. M., Bouwer, E. J., Lundquist, T. J., et al. (2019). Production of lipid-containing algal-bacterial polyculture in wastewater and biomethanation of lipid extracted residues: Enhancing methane yield through hydrothermal pretreatment and relieving solvent toxicity through co-digestion. Sci. Total Environ. 653, 1377–1394. doi:10.1016/J.SCITOTENV.2018.11.026
Borah, D., Das, N., Das, N., Bhattacharjee, A., Sarmah, P., Ghosh, K., et al. (2020). Alga-mediated facile green synthesis of silver nanoparticles: Photophysical, catalytic and antibacterial activity. Appl. Organomet. Chem. 34, e5597. doi:10.1002/AOC.5597
Carvalho, A. P., Meireles, L. A., and Malcata, F. X. (2006). Microalgal reactors: A review of enclosed system designs and performances. Biotechnol. Prog. 22, 1490–1506. doi:10.1002/bp060065r
Challagulla, V., Nayar, S., Walsh, K., and Fabbro, L. (2016). Advances in techniques for assessment of microalgal lipids. Crit. Rev. Biotechnol. 37, 566–578. doi:10.1080/07388551.2016.1206058
Chandra, R., Vishal, G., Sánchez, C. E. G., and Uribe, J. A. G. (2020). Bioreactor for algae cultivation and biodiesel production. Bioreact. Sustain. Des. Industrial Appl. Mitig. GHG Emiss., 289–307. doi:10.1016/B978-0-12-821264-6.00015-2
Chandrasekhar, K., Raj, T., Ramanaiah, S. V., Kumar, G., Banu, J. R., Varjani, S., et al. (2022). Algae biorefinery: A promising approach to promote microalgae industry and waste utilization. J. Biotechnol. 345, 1–16. doi:10.1016/j.jbiotec.2021.12.008
Chen, W., Zhang, C., Song, L., Sommerfeld, M., and Hu, Q. (2009). A high throughput Nile red method for quantitative measurement of neutral lipids in microalgae. J. Microbiol. Methods 77, 41–47. doi:10.1016/J.MIMET.2009.01.001
Chisti, Y. (2008). Biodiesel from microalgae beats bioethanol. Trends Biotechnol. 26, 126–131. doi:10.1016/j.tibtech.2007.12.002
Chittora, D., Meena, M., Barupal, T., Swapnil, P., and Sharma, K. (2020). Cyanobacteria as a source of biofertilizers for sustainable agriculture. Biochem. Biophysics Rep. 22, 100737. doi:10.1016/j.bbrep.2020.100737
Choi, H. J., and Lee, S. M. (2015). Effect of the N/P ratio on biomass productivity and nutrient removal from municipal wastewater. Bioprocess Biosyst. Eng. 38, 761–766. doi:10.1007/s00449-014-1317-z
Chokshi, K., Pancha, I., Ghosh, T., Paliwal, C., Maurya, R., Ghosh, A., et al. (2016). Green synthesis, characterization and antioxidant potential of silver nanoparticles biosynthesized from de-oiled biomass of thermotolerant oleaginous microalgae: Acutodesmus dimorphus. RSC Adv. 6, 72269–72274. doi:10.1039/C6RA15322D
Chozhavendhan, S., Vijay Pradhap Singh, M., Fransila, B., Praveen Kumar, R., and Karthiga Devi, G. (2020). A review on influencing parameters of biodiesel production and purification processes. Curr. Res. Green Sustain. Chem. 1 (2), 1–6. doi:10.1016/J.CRGSC.2020.04.002
Christaki, E., Florou-Paneri, P., and Bonos, E. (2011). Microalgae: A novel ingredient in nutrition. Int. J. Food Sci. Nutr. 62, 794–799. doi:10.3109/09637486.2011.582460
Chu, W. L. (2017). Strategies to enhance production of microalgal biomass and lipids for biofuel feedstock. Eur. J. Phycol. 52, 419–437. doi:10.1080/09670262.2017.1379100
Chugh, M., Kumar, L., Shah, D. M. P., and Bharadvaja, D. N. (2022). Algal bioremediation of heavy metals: An insight into removal mechanisms, recovery of by-products, challenges, and future opportunities. Energy Nexus 7, 10012. doi:10.1016/J.NEXUS.2022.100129
Cravotto, G., Boffa, L., Mantegna, S., Perego, P., Avogadro, M., and Cintas, P. (2008). Improved extraction of vegetable oils under high-intensity ultrasound and/or microwaves. Ultrason. Sonochemistry 15, 898–902. doi:10.1016/J.ULTSONCH.2007.10.009
Cronan, J. E., and Waldrop, G. L. (2002). Multi-subunit acetyl-CoA carboxylases. Prog. Lipid Res. 41, 407–435. doi:10.1016/S0163-7827(02)00007-3
Das, D., Mazumdar, P., Maity, A., Tripathy, S., Roy, S., Chattopadhyay, D., et al. (2016). Algal biorefinery: An integrated approach. J. Photochem. Photobiol. B 156, 1–10. doi:10.1016/j.jphotobiol.2016.01.003
de Medeiros, V. P. B., de Souza, E. L., de Albuquerque, T. M. R., da Costa Sassi, C. F., dos Santos Lima, M., Sivieri, K., et al. (2021). Freshwater microalgae biomasses exert a prebiotic effect on human colonic microbiota. Algal Res. 60, 102547. doi:10.1016/J.ALGAL.2021.102547
Díaz-Santos, E. (2019). Towards the genetic manipulation of microalgae to improve the carbon dioxide fixation and the production of biofuels: Present status and future prospect. Microalgae Biotechnol. Dev. Biofuel Wastewater Treat., 135–146. doi:10.1007/978-981-13-2264-8_7
Dołowy, M., and Pyka, A. (2015). Chromatographic methods in the separation of long-chain mono- and polyunsaturated fatty acids. J. Chem., 1–20. doi:10.1155/2015/120830
Doucha, J., and Lívanský, K. (2006). Productivity, CO2/O2 exchange and hydraulics in outdoor open high density microalgal (Chlorella sp.) photobioreactors operated in a Middle and Southern European climate. J. Appl. Phycol. 18 (18), 811–826. doi:10.1007/S10811-006-9100-4
Driver, T., Bajhaiya, A., and Pittman, J. K. (2014). Potential of bioenergy production from microalgae. Curr. Sustain. Renew. Energy Rep. 1, 94–103. doi:10.1007/s40518-014-0011-8
Ducat, D. C., Way, J. C., and Silver, P. A. (2011). Engineering cyanobacteria to generate high-value products. Trends Biotechnol. 29, 95–103. doi:10.1016/J.TIBTECH.2010.12.003
Dunahay, T. G., Jarvis, E. E., and Roessler, P. G. (1995). Genetic transformation of the diatoms cyclotella cryptica and navicula saprophila. J. Phycol. 31, 1004–1012. doi:10.1111/J.0022-3646.1995.01004.X
Fan, J., Cui, Y., Wan, M., Wang, W., and Li, Y. (2014). Lipid accumulation and biosynthesis genes response of the oleaginous Chlorella pyrenoidosa under three nutrition stressors. Biotechnol. Biofuels 7, 17–14. doi:10.1186/1754-6834-7-17
Gao, M. T., Shimamura, T., Ishida, N., and Takahashi, H. (2012). Investigation of utilization of the algal biomass residue after oil extraction to lower the total production cost of biodiesel. J. Biosci. Bioeng. 3, 330–333. doi:10.1016/J.JBIOSC.2012.04.002
Gholami, A., Pourfayaz, F., and Maleki, A. (2020). Recent advances of biodiesel production using ionic liquids supported on nanoporous materials as catalysts: A review. Fron. Energy Res. 8, 144. doi:10.3389/FENRG.2020.00144/BIBTEX
Gifuni, I., Pollio, A., Safi, C., Marzoc++ chella, A., and Olivieri, G. (2019). Current bottlenecks and challenges of the microalgal biorefinery. Trends Biotechnol. 37, 242–252. doi:10.1016/J.TIBTECH.2018.09.006
Giridhar Babu, A., Wu, X., Kabra, A. N., and Kim, D.-P. (2017). Cultivation of an indigenous Chlorella sorokiniana with phytohormones for biomass and lipid production under N-limitation. Algal Res. 23, 178–185. doi:10.1016/j.algal.2017.02.004
Godbole, V., Pal, M. K., and Gautam, P. (2021). A critical perspective on the scope of interdisciplinary approaches used in fourth-generation biofuel production. Algal Res. 58, 102436. doi:10.1016/J.ALGAL.2021.102436
Gonçalves, A. L., Pires, J. C. M., and Simões, M. (2013). Green fuel production: Processes applied to microalgae. Environ. Chem. Lett. 11, 315–324. doi:10.1007/S10311-013-0425-3
Gondi, R., Kavitha, S., Yukesh Kannah, R., Kumar, G., and Rajesh Banu, J. (2022). Wastewater based microalgae valorization for biofuel and value-added products recovery. Sustain. Energy Technol. Assess. 53, 102443. doi:10.1016/J.SETA.2022.102443
Gong, Y., and Jiang, M. (2011). Biodiesel production with microalgae as feedstock: From strains to biodiesel. Biotechnol. Lett. 33, 1269–1284. doi:10.1007/s10529-011-0574-z
Gressel, J. (2007). Transgenics are imperative for biofuel crops. Plant Sci. 174. doi:10.1016/j.plantsci.2007.11.009
Guldhe, A., Renuka, N., Singh, P., and Bux, F. (2019). Effect of phytohormones from different classes on gene expression of Chlorella sorokiniana under nitrogen limitation for enhanced biomass and lipid production. Algal Res. 40, 101518. doi:10.1016/j.algal.2019.101518
Han, X., Li, Z., Wen, Y., and Chen, Z. (2021). Overproduction of docosahexaenoic acid in Schizochytrium sp. through genetic engineering of oxidative stress defense pathways. Biotechnol. Biofuels 14, 70–10. doi:10.1186/s13068-021-01918-w
Hang, L. T., Mori, K., Tanaka, Y., Morikawa, M., and Toyama, T. (2020). Enhanced lipid productivity of Chlamydomonas reinhardtii with combination of NaCl and CaCl2 stresses. Bioprocess Biosyst. Eng. 43, 971–980. doi:10.1007/S00449-020-02293-W
Hathwaik, L. T., and Cushman, J. C. (2017). Strain selection strategies for improvement of algal biofuel feedstocks. Biofuels Bioenergy 1, 173–189. doi:10.1002/9781118350553.ch11
Hsieh, H. J., Su, C. H., and Chien, L. J. (2012). Accumulation of lipid production in Chlorella minutissima by triacylglycerol biosynthesis-related genes cloned from Saccharomyces cerevisiae and Yarrowia lipolytica. J. Microbiol. 50, 526–534. doi:10.1007/S12275-012-2041-5
Huang, Q., Jiang, F., Wang, L., and Yang, C. (2017). Design of photobioreactors for mass cultivation of photosynthetic organisms. Engineering 3, 318–329. doi:10.1016/J.ENG.2017.03.020
IEA (2021a). Renewables 2021. Paris, France: International Energy Agency (IEA) Publications International, 167.
Islam, M. S., Aryasomayajula, A., and Selvaganapathy, P. R. (2017). A review on macroscale and microscale cell lysis methods. Micromachines 8 (3). doi:10.3390/mi8030083
Jeevanandam, J., and Danquah, M. K. (2020). “Dewatering and drying of algal cultures,” in Handbook of microalgae-based processes and products: Fundamentals and advances in energy, food, feed, fertilizer, and bioactive compounds, 207–224. doi:10.1016/B978-0-12-818536-0.00009-9
Jeong, G. T., and Kim, S. K. (2020). Valorization of thermochemical conversion of lipid-extracted microalgae to levulinic acid. Bioresour. Technol. 313, 123684. doi:10.1016/J.BIORTECH.2020.123684
Jha, M. N., Prasad, A. N., Sharma, S. G., and Bharati, R. C. (2001). Effects of fertilization rate and crop rotation on diazotrophic cyanobacteria in paddy field. World J. Microbiol. Biotechnol. 17, 463–468. doi:10.1023/A:1011913316988
Jusoh, M., Loh, S. H., Chuah, T. S., Aziz, A., and Cha, T. S. (2015). Elucidating the role of jasmonic acid in oil accumulation, fatty acid composition and gene expression in Chlorella vulgaris (Trebouxiophyceae) during early stationary growth phase. Algal Res. 9, 14–20. doi:10.1016/J.ALGAL.2015.02.020
Karim, A., Amirul Islam, M., Khalid, Z. B., Faizal, C. K. M., Khan, M. M. R., and Yousuf, A. (2019). Microalgal cell disruption and lipid extraction techniques for potential biofuel production. Microalgae Cultiv. Biofuels Prod. 2020. doi:10.1016/B978-0-12-817536-1.00009-6
Karpanai Selvan, B., Das, S., Chandrasekar, M., Girija, R., John Vennison, S., Jaya, N., et al. (2022). Utilization of biodiesel blended fuel in a diesel engine–Combustion engine performance and emission characteristics study. Fuel 311, 122621. doi:10.1016/J.FUEL.2021.122621
Karthik, V., Senthil Kumar, P., Dai, V., Vo, N., Sindhu, J., Sneka, D., et al. (2021). Hydrothermal production of algal biochar for environmental and fertilizer applications: A review. Environ. Chem. Lett. 19, 1025–1042. doi:10.1007/s10311-020-01139-x
Kashyap, M., Samadhiya, K., Ghosh, A., Anand, V., Shirage, P. M., and Bala, K. (2019). Screening of microalgae for biosynthesis and optimization of Ag/AgCl nano hybrids having antibacterial effect. RSC Adv. 9, 25583–25591. doi:10.1039/C9RA04451E
Khandelwal, A., Vijay, A., Dixit, A., and Chhabra, M. (2018). Microbial fuel cell powered by lipid extracted algae: A promising system for algal lipids and power generation. Bioresour. Technol. 247, 520–527. doi:10.1016/J.BIORTECH.2017.09.119
Kim, H. S., Hsu, S. C., Han, S. I., Thapa, H. R., Guzman, A. R., Browne, D. R., et al. (2017). High-throughput droplet microfluidics screening platform for selecting fast-growing and high lipid-producing microalgae from a mutant library. Plant Direct 1, e00011. doi:10.1002/PLD3.11
Kim, Y. H., Choi, Y. K., Park, J., Lee, S., Yang, Y. H., Kim, H. J., et al. (2012). Ionic liquid-mediated extraction of lipids from algal biomass. Bioresour. Technol. 109, 312–315. doi:10.1016/J.BIORTECH.2011.04.064
Klok, A. J., Lamers, P. P., Martens, D. E., Draaisma, R. B., and Wijffels, R. H. (2014). Edible oils from microalgae: Insights in TAG accumulation. Trends Biotechnol. 32, 521–528. doi:10.1016/J.TIBTECH.2014.07.004
Koyande, A. K., Chew, K. W., Rambabu, K., Tao, Y., Chu, D. T., and Show, P. L. (2019). Microalgae: A potential alternative to health supplementation for humans. Food Sci. Hum. Wellness 8, 16–24. doi:10.1016/J.FSHW.2019.03.001
Kumar, A., Ergas, S., Yuan, X., Sahu, A., Zhang, Q., Dewulf, J., et al. (2010). Enhanced CO2 fixation and biofuel production via microalgae: Recent developments and future directions. Trends Biotechnol. 28, 371–380. doi:10.1016/J.TIBTECH.2010.04.004
Kumar, L., Anand, R., Shah, M. P., and Bharadvaja, N. (2022). Microalgae biodiesel: A sustainable source of energy, unit operations, technological challenges, and solutions. J. Hazard. Mater. Adv. 8, a100145. doi:10.1016/J.HAZADV.2022.100145
Kumar, L., Mohan, L., Anand, S., Bhardwaj, D., and Bharadvaja, N. (2022b). Phyconanoremediation: A sustainable approach to deal with environmental pollutants heavy metals and dyes. Vegetos. doi:10.1007/s42535-022-00399-y
Kumar, R. R., Rao, P. H., and Arumugam, M. (2015). Lipid extraction methods from microalgae: A comprehensive review. Front. Energy Res. 3, 61. doi:10.3389/fenrg.2014.00061
Kwak, H. S., Kim, J. Y. H., Woo, H. M., Jin, E. S., Min, B. K., and Sim, S. J. (2016). Synergistic effect of multiple stress conditions for improving microalgal lipid production. Algal Res. 19, 215–224. doi:10.1016/J.ALGAL.2016.09.003
Lackner, K. S. (2003). A guide to CO2 sequestration. Science 300, 1677–1678. doi:10.1126/science.1079033
Larkum, A. W. D., Ross, I. L., Kruse, O., and Hankamer, B. (2012). Selection, breeding and engineering of microalgae for bioenergy and biofuel production. Trends Biotechnol. 30, 198–205. doi:10.1016/j.tibtech.2011.11.003
Lee, O. K., Kim, A. L., Seong, D. H., Lee, C. G., Jung, Y. T., Lee, J. W., et al. (2013). Chemo-enzymatic saccharification and bioethanol fermentation of lipid-extracted residual biomass of the microalga, Dunaliella tertiolecta. Bioresour. Technol. 132, 197–201. doi:10.1016/J.BIORTECH.2013.01.007
Lehr, F., and Posten, C. (2009). Closed photo-bioreactors as tools for biofuel production. Curr. Opin. Biotechnol. 20, 280–285. doi:10.1016/J.COPBIO.2009.04.004
Li, Y., Ghasemi Naghdi, F., Garg, S., Adarme-Vega, T. C., Thurecht, K. J., Ghafor, W. A., et al. (2014). A comparative study: The impact of different lipid extraction methods on current microalgal lipid research. Microb. Cell Fact. 13, 14–19. doi:10.1186/1475-2859-13-14
Lim, D. K. Y., Schenk, P. M., Lim, D. K. Y., and Schenk, P. M. (2017). Microalgae selection and improvement as oil crops: GM vs non-GM strain engineering. AIMS Bioeng. 2017, 151–161. doi:10.3934/BIOENG.2017.1.151
Lin, W. R., Lai, Y. C., Sung, P. K., Tan, S. I., Chang, C. H., Chen, C. Y., et al. (2018). Enhancing carbon capture and lipid accumulation by genetic carbonic anhydrase in microalgae. J. Taiwan Inst. Chem. Eng. 93, 131–141. doi:10.1016/J.JTICE.2018.10.010
Lin-Lan, Z., Jing-Han, W., and Hong-Ying, H. (2018). Differences between attached and suspended microalgal cells in ssPBR from the perspective of physiological properties. J. Photochem. Photobiol. B Biol. 181, 164–169. doi:10.1016/j.jphotobiol.2018.03.014
Liu, X., Saydah, B., Eranki, P., Colosi, L. M., Greg Mitchell, B., Rhodes, J., et al. (2013). Pilot-scale data provide enhanced estimates of the life cycle energy and emissions profile of algae biofuels produced via hydrothermal liquefaction. Bioresour. Technol. 148, 163–171. doi:10.1016/j.biortech.2013.08.112
Lorente, E., Hapońska, M., Clavero, E., Torras, C., and Salvadó, J. (2018). Steam explosion and vibrating membrane filtration to improve the processing cost of microalgae cell disruption and fractionation. Processes 6(4). doi:10.3390/pr6040028
Lowrey, J., Brooks, M. S., and Armenta, R. E. (2016). Nutrient recycling of lipid-extracted waste in the production of an oleaginous thraustochytrid. Appl. Microbiol. Biotechnol. 100, 4711–4721. doi:10.1007/S00253-016-7463-2
Ma, X., Zheng, H., Huang, H., Liu, Y., and Ruan, R. (2014). Effects of temperature and substrate concentration on lipid production by Chlorella vulgaris from enzymatic hydrolysates of lipid-extracted microalgal biomass residues (LMBRs). Appl. Biochem. Biotechnol. 174, 1631–1650. doi:10.1007/S12010-014-1134-5
Madeira, M. S., Cardoso, C., Lopes, P. A., Coelho, D., Afonso, C., Bandarra, N. M., et al. (2017). Microalgae as feed ingredients for livestock production and meat quality: A review. Livest. Sci. 205, 111–121. doi:10.1016/J.LIVSCI.2017.09.020
Mata, T. M., Martins, A. A., and Caetane, N. S. (2012). Microalgae processing for biodiesel production. Adv. Biodiesel Prod. Process. Technol., 204–231. doi:10.1016/B978-0-85709-117-8.50009-5
Maurya, R., Chokshi, K., Ghosh, T., Trivedi, K., Pancha, I., Kubavat, D., et al. (2016a). Lipid extracted microalgal biomass residue as a fertilizer substitute for Zea mays L. Front. Plant Sci. 6, 1266. doi:10.3389/fpls.2015.01266
Maurya, R., Paliwal, C., Chokshi, K., Pancha, I., Ghosh, T., Satpati, G. G., et al. (2016b). Hydrolysate of lipid extracted microalgal biomass residue: An algal growth promoter and enhancer. Bioresour. Technol. 207, 197–204. doi:10.1016/J.BIORTECH.2016.02.018
McMillan, J. R., Watson, I. A., Ali, M., and Jaafar, W. (2013). Evaluation and comparison of algal cell disruption methods: Microwave, waterbath, blender, ultrasonic and laser treatment. Appl. Energy 103, 128–134. doi:10.1016/J.APENERGY.2012.09.020
Mercer, P., and Armenta, R. E. (2011). Developments in oil extraction from microalgae. Eur. J. Lipid Sci. Technol. 113, 539–547. doi:10.1002/EJLT.201000455
Milledge, J. J. (2011). Commercial application of microalgae other than as biofuels: A brief review. Rev. Environ. Sci. Biotechnol. 10, 31–41. doi:10.1007/s11157-010-9214-7
Mirzaei, S. Z., Ahmadi Somaghian, S., Lashgarian, H. E., Karkhane, M., Cheraghipour, K., and Marzban, A. (2021). Phyco-fabrication of bimetallic nanoparticles (zinc–selenium) using aqueous extract of Gracilaria corticata and its biological activity potentials. Ceram. Int. 47, 5580–5586. doi:10.1016/J.CERAMINT.2020.10.142
Mishra, S., and Mohanty, K. (2019). Comprehensive characterization of microalgal isolates and lipid-extracted biomass as zero-waste bioenergy feedstock: An integrated bioremediation and biorefinery approach. Bioresour. Technol. 273, 177–184. doi:10.1016/J.BIORTECH.2018.11.012
Mohammadi, M., Sedighi, M., Natarajan, R., Hassan, S. H. A., and Ghasemi, M. (2021). Microbial fuel cell for oilfield produced water treatment and reuse: Modelling and process optimization. Korean J. Chem. Engin. 38 (1), 72–80. doi:10.1007/S11814-020-0674-3
Molina Grima, E., Belarbi, E. H., Acién Fernández, F. G., Robles Medina, A., and Chisti, Y. (2003). Recovery of microalgal biomass and metabolites: Process options and economics. Biotechnol. Adv. 20, 491–515. doi:10.1016/S0734-9750(02)00050-2
Mondal, M., Goswami, S., Ghosh, A., Oinam, G., Tiwari, O. N., Das, P., et al. (2017). Production of biodiesel from microalgae through biological carbon capture: A review. 3 Biotech. 7, 99–21. doi:10.1007/s13205-017-0727-4
Moon, M., Kim, C. W., Farooq, W., Suh, W. I., Shrivastav, A., Park, M. S., et al. (2014). Utilization of lipid extracted algal biomass and sugar factory wastewater for algal growth and lipid enhancement of Ettlia sp. Bioresour. Technol. 163, 180–185. doi:10.1016/J.BIORTECH.2014.04.033
Morales, M., Aflalo, C., and Bernard, O. (2021). Microalgal lipids: A review of lipids potential and quantification for 95 phytoplankton species. Biomass Bioenergy 150, 106108. doi:10.1016/J.BIOMBIOE.2021.106108
Morschett, H., Wiechert, W., and Oldiges, M. (2016). Automation of a Nile red staining assay enables high throughput quantification of microalgal lipid production. Microb. Cell Fact. 15, 34–11. doi:10.1186/s12934-016-0433-7
Mota, G. C. P., Moraes, L. B. S. de, Oliveira, C. Y. B., Oliveira, D. W. S., Abreu, J. L. de, Dantas, D. M. M., et al. (2022). Astaxanthin from Haematococcus pluvialis : Processes, applications, and market. Prep. Biochem. Biotechnol. 52, 598–609. doi:10.1080/10826068.2021.1966802
Muñoz, C. F., Weusthuis, R. A., D’Adamo, S., and Wijffels, R. H. (2019). Effect of single and combined expression of lysophosphatidic acid acyltransferase, glycerol-3-phosphate acyltransferase, and diacylglycerol acyltransferase on lipid accumulation and composition in Neochloris oleoabundans. Front. Plant Sci. 10, 1573. doi:10.3389/FPLS.2019.01573
Mutanda, T., Ramesh, D., Karthikeyan, S., Kumari, S., Anandraj, A., and Bux, F. (2011). Bioprospecting for hyper-lipid producing microalgal strains for sustainable biofuel production. Bioresour. Technol. 102, 57–70. doi:10.1016/J.BIORTECH.2010.06.077
Nagappan, S., Devendran, S., Tsai, P. C., Dahms, H. U., and Ponnusamy, V. K. (2019). Potential of two-stage cultivation in microalgae biofuel production. Fuel 252, 339–349. doi:10.1016/j.fuel.2019.04.138
Nandhini, R., Berslin, D., Sivaprakash, B., Rajamohan, N., and Vo, D. V. N. (2022). Thermochemical conversion of municipal solid waste into energy and hydrogen: a review. Environ. Chem. Let. 20 (3), 1645–1669. doi:10.1007/S10311-022-01410-3
Ng, I. S., Tan, S. I., Kao, P. H., Chang, Y. K., and Chang, J. S. (2017). Recent developments on genetic engineering of microalgae for biofuels and bio-based chemicals. Biotechnol. J. 12, 1600644. doi:10.1002/BIOT.201600644
Nguyen, T. H. T., Park, S., Jeong, J., Shin, Y. S., Sim, S. J., and Jin, E. S. (2020). Enhancing lipid productivity by modulating lipid catabolism using the CRISPR-Cas9 system in Chlamydomonas. J. Appl. Phycol. 32, 2829–2840. doi:10.1007/S10811-020-02172-7
Nigam, H., Malik, A., and Singh, V. (2021). A novel nanoemulsion-based microalgal growth medium for enhanced biomass production. Biotechnol. Biofuels 14, 111–118. doi:10.1186/s13068-021-01960-8
Park, H., Jung, D., Lee, J., Kim, P., Cho, Y., Jung, I., et al. (2018). Improvement of biomass and fatty acid productivity in ocean cultivation of Tetraselmis sp. using hypersaline medium. J. Appl. Phycol. 30, 2725–2735. doi:10.1007/S10811-018-1388-3
Parsaeimehr, A., Mancera-Andrade, E. I., Robledo-Padilla, F., Iqbal, H. M. N., and Parra-Saldivar, R. (2017). A chemical approach to manipulate the algal growth, lipid content and high-value alpha-linolenic acid for biodiesel production. Algal Res. 26, 312–322. doi:10.1016/j.algal.2017.08.016
Pavithra, K. G., Kumar, P. S., Jaikumar, V., Vardhan, K. H., and SundarRajan, P. S. (2020). Microalgae for biofuel production and removal of heavy metals: A review. Environ. Chem. Lett. 18, 1905–1923. doi:10.1007/S10311-020-01046-1
Peng, L., Fu, D., Chu, H., Wang, Z., and Qi, H. (2020). Biofuel production from microalgae: A review. Environ. Chem. Lett. 18, 285–297. doi:10.1007/S10311-019-00939-0
Popp, J., Lakner, Z., Harangi-Rákos, M., and Fári, M. (2014). The effect of bioenergy expansion: Food, energy, and environment. Renew. Sustain. Energy Rev. 32, 559–578. doi:10.1016/J.RSER.2014.01.056
Purton, S., Szaub, J. B., Wannathong, T., Young, R., and Economou, C. K. (2013). Genetic engineering of algal chloroplasts: Progress and prospects. Russ. J. Plant Physiol. 60, 491–499. doi:10.1134/S1021443713040146
Qiu, R., Gao, S., Lopez, P. A., and Ogden, K. L. (2017). Effects of pH on cell growth, lipid production and CO2 addition of microalgae Chlorella sorokiniana. Algal Res. 28, 192–199. doi:10.1016/j.algal.2017.11.004
Quader, M. A., and Ahmed, S. (2017). Bioenergy with carbon capture and storage (BECCS): Future prospects of carbon-negative technologies. Clean Energy for Sustainable Development: Comparisons and Contrasts of New Approaches Amsterdam, Netherlands: Elsevier Inc, 91–140. doi:10.1016/B978-0-12-805423-9.00004-1
Quan, Y., Pehkonen, S. O., and Ray, M. B. (2004). Evaluation of three different lamp emission models using novel application of potassium ferrioxalate actinometry. Ind. Eng. Chem. Res. 43, 948–955. doi:10.1021/ie0304210
Rahman, A., Kumar, S., and Nawaz, T. (2020). Biosynthesis of nanomaterials using algae. Microalgae Cultiv. Biofuels Prod., 265–279. doi:10.1016/B978-0-12-817536-1.00017-5
Rajeswari, S., Baskaran, D., Saravanan, P., Rajasimman, M., Rajamohan, N., and Vasseghian, Y. (2022). Production of ethanol from biomass–Recent research, scientometric review and future perspectives. Fuel 317, 123448. doi:10.1016/J.FUEL.2022.123448
Raven, J. A., and Beardall, J. (2003). Carbohydrate metabolism and respiration in algae, 205–224. doi:10.1007/978-94-007-1038-2_10
Remmers, I. M., Wijffels, R. H., Barbosa, M. J., and Lamers, P. P. (2018). Can we approach theoretical lipid yields in microalgae? Trends Biotechnol. 36, 265–276. doi:10.1016/J.TIBTECH.2017.10.020
Rengel, R., Smith, R. T., Haslam, R. P., Sayanova, O., Vila, M., and León, R. (2018). Overexpression of acetyl-CoA synthetase (ACS) enhances the biosynthesis of neutral lipids and starch in the green microalga Chlamydomonas reinhardtii. Algal Res. 31, 183–193. doi:10.1016/j.algal.2018.02.009
Renuka, N., Guldhe, A., Singh, P., Ansari, F. A., Rawat, I., and Bux, F. (2017). Evaluating the potential of cytokinins for biomass and lipid enhancement in microalga Acutodesmus obliquus under nitrogen stress. Energy Convers. Manag. 140, 14–23. doi:10.1016/J.ENCONMAN.2017.02.065
Richmond, A. (2004). Principles for attaining maximal microalgal productivity in photobioreactors: An overview. Hydrobiologia 512 (Table 1), 33–37. doi:10.1023/B:HYDR.0000020365.06145.36
Romel Malapascua, J. (2012). Development of an indirect method of microalgal lipid quantification using a lysochrome dye, Nile red. Afr. J. Biotechnol. 11, 13518–13527. doi:10.5897/ajb12.1142
Rosa, G. M., Morais, M. G., and Costa, J. A. V. (2019). Fed-batch cultivation with CO2 and monoethanolamine: Influence on Chlorella fusca LEB 111 cultivation, carbon biofixation and biomolecules production. Bioresour. Technol. 273, 627–633. doi:10.1016/J.BIORTECH.2018.11.010
Rosales-Mendoza, S., Paz-Maldonado, L. M. T., and Soria-Guerra, R. E. (2012). Chlamydomonas reinhardtii as a viable platform for the production of recombinant proteins: Current status and perspectives. Plant Cell Rep. 31, 479–494. doi:10.1007/s00299-011-1186-8
Salama, E. S., Kabra, A. N., Ji, M. K., Kim, J. R., Min, B., and Jeon, B. H. (2014). Enhancement of microalgae growth and fatty acid content under the influence of phytohormones. Bioresour. Technol. 172, 97–103. doi:10.1016/J.BIORTECH.2014.09.002
Sathishkumar, R. S., Sundaramanickam, A., Srinath, R., Ramesh, T., Saranya, K., Meena, M., et al. (2019). Green synthesis of silver nanoparticles by bloom forming marine microalgae Trichodesmium erythraeum and its applications in antioxidant, drug-resistant bacteria, and cytotoxicity activity. J. Saudi Chem. Soc. 23, 1180–1191. doi:10.1016/J.JSCS.2019.07.008
Sati, H., Mitra, M., Mishra, S., and Baredar, P. (2019). Microalgal lipid extraction strategies for biodiesel production: A review. Algal Res. 38, 101413. doi:10.1016/j.algal.2019.101413
Shahid, A., Rehman, A., Usman, M., Ashraf, M. U. F., Javed, M. R., Khan, A. Z., et al. (2020). Engineering the metabolic pathways of lipid biosynthesis to develop robust microalgal strains for biodiesel production. Biotechnol. Appl. Biochem. 67, 41–51. doi:10.1002/BAB.1812
Sharma, K. K., Schuhmann, H., and Schenk, P. M. (2012). High lipid induction in microalgae for biodiesel production. Energies 5 (5), 15321532–15531553. doi:10.3390/EN5051532
Sharma, P. K., Goud, V. V., Yamamoto, Y., and Sahoo, L. (2021). Efficient Agrobacterium tumefaciens-mediated stable genetic transformation of green microalgae, Chlorella sorokiniana. 3 Biotech. 311, 196–207. doi:10.1007/s13205-021-02750-7
Shen, G., Schluchter, W. M., and Bryant, D. A. (2008). Biogenesis of phycobiliproteins: I. cpcS-I and cpcU mutants of the cyanobacterium synechococcus sp. pcc 7002 define a heterodimeric phyococyanobilin lyase specific for β-phycocyanin and allophycocyanin subunits. J. Biol. Chem. 283, 7503–7512. doi:10.1074/JBC.M708164200
Shokravi, Z., Shokravi, H., Chyuan, O. H., Lau, W. J., Koloor, S. S. R., Petrů, M., et al. (2020). Improving ‘lipid productivity’ in microalgae by bilateral enhancement of biomass and lipid contents: A review. Sustainability 12, 9083. doi:10.3390/SU12219083
Sivagnanam, S. P., Tilahun Getachew, A., Choi, J. H., Park, Y. B., Woo, H. C., and Chun, B. S. (2017). Green synthesis of silver nanoparticles from deoiled Brown algal extract via Box-Behnken based design and their antimicrobial and sensing properties. Green Process. Synthesis 6, 147–160. doi:10.1515/GPS-2016-0052/ASSET/GRAPHIC/J_GPS-2016-0052_FIG_009.JPG
Soltani, S., Saadatmand, S., Khavarinejad, R., and Nejadsattari, T. (2011). Antioxidant and antibacterial activities of cladophora glomerata (L.) kütz. In caspian sea coast, Iran. Afr. J. Biotechnol. 10, 7684–7689. doi:10.5897/AJB11.491
Stephens, E., Ross, I. L., Mussgnug, J. H., Wagner, L. D., Borowitzka, M. A., Posten, C., et al. (2010). Future prospects of microalgal biofuel production systems. Trends Plant Sci. 15 (10), 554–564. doi:10.1016/J.TPLANTS.2010.06.003
Suali, E., and Sarbatly, R. (2012). Conversion of microalgae to biofuel. Renew. Sustain. Energy Rev. 16, 4316–4342. doi:10.1016/j.rser.2012.03.047
Sun, X. M., Ren, L. J., Zhao, Q. Y., Ji, X. J., and Huang, H. (2019). Enhancement of lipid accumulation in microalgae by metabolic engineering. Biochimica Biophysica Acta (BBA) - Mol. Cell Biol. Lipids 1864, 552–566. doi:10.1016/J.BBALIP.2018.10.004
Suarez Garcia, E., Lo, C., Eppink, M. H. M., Wijffels, R. H., and van den Berg, C. (2019). Understanding mild cell disintegration of microalgae in bead mills for the release of biomolecules. Chem. Eng. Sci. 203, 380–390. doi:10.1016/J.CES.2019.04.008
Taher, H., Al-Zuhair, S., Al-Marzouqi, A. H., Haik, Y., and Farid, M. M. (2011). A review of enzymatic transesterification of microalgal oil-based biodiesel using supercritical technology. Enzyme Res., 1–25. doi:10.4061/2011/468292
Tang, D. Y. Y., Yew, G. Y., Koyande, A. K., Chew, K. W., Vo, D. V. N., and Show, P. L. (2020). Green technology for the industrial production of biofuels and bioproducts from microalgae: A review. Environ. Chem. Lett. 18, 1967. doi:10.1007/S10311-020-01052-3
Tran, D. T., Lee, H. R., Jung, S., Park, M. S., and Yang, J. W. (2018). Lipid-extracted algal biomass based biocomposites fabrication with poly(vinyl alcohol). Algal Res. 31, 525–533. doi:10.1016/J.ALGAL.2016.08.016
Trentacoste, E. M., Shrestha, R. P., Smith, S. R., Glé, C., Hartmann, A. C., Hildebrand, M., et al. (2013). Metabolic engineering of lipid catabolism increases microalgal lipid accumulation without compromising growth. Proc. Natl. Acad. Sci. U. S. A. 110, 19748–19753. doi:10.1073/PNAS.1309299110
Vasilieva, S. G., Lobakova, E. S., Lukyanov, A. A., and Solovchenko, A. E. (2016). Immobilized microalgae in biotechnology. Mosc. Univ. Biol. Sci. Bull. 71, 170–176. doi:10.3103/S0096392516030135
Venkata Subhash, G., Rajvanshi, M., Navish Kumar, B., Govindachary, S., Prasad, V., and Dasgupta, S. (2017). Carbon streaming in microalgae: extraction and analysis methods for high value compounds. Biores. Technol. 244, 1304–1316. doi:10.1016/J.BIORTECH.2017.07.024
Vorobiev, E., Lebovka, N. I., Redondo, L. M., Pataro, G., Carullo, D., Donsì, F., et al. (2020). Pulsed electric fields-assisted extraction of valuable compounds from Arthrospira Platensis: Effect of pulse polarity and mild heating Front. Bioeng. Biotechnol. 8, 551272. doi:10.3389/fbioe.2020.551272
Wang, B., Li, Y., Wu, N., and Lan, C. Q. (2008). CO2 bio-mitigation using microalgae. Appl. Microbiol. Biotechnol. 79, 707–718. doi:10.1007/s00253-008-1518-y
Wang, C., Guo, L., Li, Y., and Wang, Z. (2012). Systematic comparison of C3 and C4 plants based on metabolic network analysis. BMC Syst. Biol. 6, S9. doi:10.1186/1752-0509-6-S2-S9
Wang, J., Liu, J., and Liu, T. (2015). The difference in effective light penetration may explain the superiority in photosynthetic efficiency of attached cultivation over the conventional open pond for microalgae. Biotechnol. Biofuels 8, 49–12. doi:10.1186/s13068-015-0240-0
Watanabe, H., Li, D., Nakagawa, Y., Tomishige, K., and Watanabe, M. M. (2015). Catalytic gasification of oil-extracted residue biomass of Botryococcus braunii. Bioresour. Technol. 191, 452–459. doi:10.1016/J.BIORTECH.2015.03.034
White, D. A., Pagarette, A., Rooks, P., and Ali, S. T. (2013). The effect of sodium bicarbonate supplementation on growth and biochemical composition of marine microalgae cultures. J. Appl. Phycol. 25, 153–165. doi:10.1007/s10811-012-9849-6
Win, T. T., Barone, G. D., Secundo, F., and Fu, P. (2018). Algal biofertilizers and plant growth stimulants for sustainable agriculture. Ind. Biotechnol. 14, 203–211. doi:10.1089/ind.2018.0010
Wu, P., Ata-Ul-Karim, S. T., Singh, B. P., Wang, H., Wu, T., Liu, C., et al. (2019). A scientometric review of biochar research in the past 20 years (1998–2018). Biochar 1, 23–43. doi:10.1007/s42773-019-00002-9
Xia, H., Riaz, M., Zhang, M., Liu, B., El-Desouki, Z., and Jiang, C. (2020). Biochar increases nitrogen use efficiency of maize by relieving aluminum toxicity and improving soil quality in acidic soil. Ecotoxicol. Environ. Saf. 196, 110531. doi:10.1016/J.ECOENV.2020.110531
Xia, L., Ge, H., Zhou, X., Zhang, D., and Hu, C. (2013). Photoautotrophic outdoor two-stage cultivation for oleaginous microalgae Scenedesmus obtusus XJ-15. Bioresour. Technol. 144, 261–267. doi:10.1016/J.BIORTECH.2013.06.112
Xue, J., Niu, Y. F., Huang, T., Yang, W. D., Liu, J. S., and Li, H. Y. (2015). Genetic improvement of the microalga Phaeodactylum tricornutum for boosting neutral lipid accumulation. Metab. Eng. 27, 1–9. doi:10.1016/J.YMBEN.2014.10.002
Yaashikaa, P. R., Keerthana Devi, M., Senthil Kumar, P., and Pandian, E. (2022). A review on biodiesel production by algal biomass: Outlook on lifecycle assessment and techno-economic analysis. Fuel 324, 124774. doi:10.1016/J.FUEL.2022.124774
Yamaguchi, K. (1996). Recent advances in microalgal bioscience in Japan, with special reference to utilization of biomass and metabolites: A review. J. Appl. Phycol. 8, 487–502. doi:10.1007/BF02186327
Yang, H., He, Q., Rong, J., Xia, L., and Hu, C. (2014). Rapid neutral lipid accumulation of the alkali-resistant oleaginous Monoraphidium dybowskii LB50 by NaCl induction. Bioresour. Technol. 172, 131–137. doi:10.1016/j.biortech.2014.08.066
Yap, J. K., Sankaran, R., Chew, K. W., Halimatul Munawaroh, H. S., Ho, S. H., Rajesh Banu, J., et al. (2021). Advancement of green technologies: A comprehensive review on the potential application of microalgae biomass. Chemosphere 281, 130886. doi:10.1016/J.CHEMOSPHERE.2021.130886
Yu, K. L., Lau, B. F., Show, P. L., Ong, H. C., Ling, T. C., Chen, W. H., et al. (2017). Recent developments on algal biochar production and characterization. Bioresour. Technol. 246, 2–11. doi:10.1016/J.BIORTECH.2017.08.009
Yukesh Kannah, R., Kavitha, S., Parthiba Karthikeyan, O., Rene, E. R., Kumar, G., Rajesh Banu, J., et al. (2021). A review on anaerobic digestion of energy and cost effective microalgae pretreatment for biogas production. Bioresour. Technol. 332, 125055. doi:10.1016/J.BIORTECH.2021.125055
Zhang, C., Xiao, Y., Ma, Y., Li, B., Liu, Z., Lu, C., et al. (2017). Algae biomass as a precursor for synthesis of nitrogen-and sulfur-co-doped carbon dots: A better probe in Arabidopsis guard cells and root tissues. J. Photochem. Photobiol. B Biol. 174, 315–322. doi:10.1016/J.JPHOTOBIOL.2017.06.024
Zhang, Y., Pan, Y., Ding, W., Hu, H., and Liu, J. (2021). Lipid production is more than doubled by manipulating a diacylglycerol acyltransferase in algae. GCB Bioenergy 13, 185–200. doi:10.1111/GCBB.12771
Zhao, B., Ma, J., Zhao, Q., Laurens, L., Jarvis, E., Chen, S., et al. (2014). Efficient anaerobic digestion of whole microalgae and lipid-extracted microalgae residues for methane energy production. Bioresour. Technol. 161, 423–430. doi:10.1016/J.BIORTECH.2014.03.079
Zheng, H., Gao, Z., Yin, F., Ji, X., and Huang, H. (2012). Effect of CO2 supply conditions on lipid production of Chlorella vulgaris from enzymatic hydrolysates of lipid-extracted microalgal biomass residues. Bioresour. Technol. 126, 24–30. doi:10.1016/J.BIORTECH.2012.09.048
Zheng, H., Ma, X., Gao, Z., Wan, Y., Min, M., Zhou, W., et al. (2015). Lipid production of heterotrophic Chlorella sp. from hydrolysate mixtures of lipid-extracted microalgal biomass residues and molasses. Appl. Biochem. Biotechnol. 177, 662–674. doi:10.1007/S12010-015-1770-4
Keywords: biodiesel, lipid productivity, biorefinery, genetic engineering, algae, carbon dioxide sequestration
Citation: Kumar L, Mohan L, Anand R, Joshi V, Chugh M and Bharadvaja N (2022) A review on unit operations, challenges, opportunities, and strategies to improve algal based biodiesel and biorefinery. Front. Chem. Eng. 4:998289. doi: 10.3389/fceng.2022.998289
Received: 19 July 2022; Accepted: 23 September 2022;
Published: 06 October 2022.
Edited by:
Kavitha S, Anna University Regional Campus Tirunelveli, IndiaReviewed by:
Vinay Kumar Tyagi, National Institute of Hydrology, IndiaCopyright © 2022 Kumar, Mohan, Anand, Joshi, Chugh and Bharadvaja. This is an open-access article distributed under the terms of the Creative Commons Attribution License (CC BY). The use, distribution or reproduction in other forums is permitted, provided the original author(s) and the copyright owner(s) are credited and that the original publication in this journal is cited, in accordance with accepted academic practice. No use, distribution or reproduction is permitted which does not comply with these terms.
*Correspondence: Lakhan Kumar, YWRhcnNoLmxha2hhbkBnbWFpbC5jb20=; Navneeta Bharadvaja, bmF2bmVldGFiQGRjZS5hYy5pbg==
Disclaimer: All claims expressed in this article are solely those of the authors and do not necessarily represent those of their affiliated organizations, or those of the publisher, the editors and the reviewers. Any product that may be evaluated in this article or claim that may be made by its manufacturer is not guaranteed or endorsed by the publisher.
Research integrity at Frontiers
Learn more about the work of our research integrity team to safeguard the quality of each article we publish.