- School of Mechanical Engineering, Shanghai Jiao Tong University, Shanghai, China
Lignin valorization via thermochemical approaches has the potential to produce renewable fuels and value-added chemicals, which are of great significance to the sustainable development of human beings. During the thermochemical depolymerization which involves acid-catalyzed, alkali-catalyzed, oxidative, reductive, pyrolytic, and other reactions, the lignin structure will undergo a series of bond cleavage, condensation, and functional group changes, while the mechanism is still unclear. To improve the efficiency, the analysis of the evolution of intermediates during depolymerization is very important, among which soft ionization mass spectrometry plays a vital role. This review aims to summarize the research progress of process analysis of lignin depolymerization in both gas-phase, typically thermal and catalytic pyrolysis, and liquid-phase via online mass spectrometry. The challenges and our insights into the future development of the lignin valorization as well as soft ionization mass spectrometry methods are also discussed.
Introduction
Under the dual pressure of energy and environmental crisis, lignocellulosic biomass, as the most abundant non-food biomass on Earth, is considered to be the only carbon-based renewable energy source that may partially replace fossil fuels (Ragauskas et al., 2006; Zhou et al., 2011; Caspeta et al., 2013). It is mainly composed of cellulose, hemicellulose, and lignin, among which lignin is the only renewable aromatic resource in nature, and has a carbon-hydrogen ratio similar to that of petroleum, and thus has a very broad prospect for utilization (Laskar et al., 2013; Ragauskas et al., 2014).
Lignin is composed of various phenylpropane units and connected by a series of C-C (β-β, β-5, β-1, 5–5′, etc.) and C-O bonds (β-O-4, α-O-4, 4-O-5, etc.) in a nonlinear and random pattern to form a variety of complex spatial structures in different plants (Figure 1A) (Li et al., 2015; Cao et al., 2020; Wang et al., 2021). Lignin valorization usually requires conversion to monomers first, and further transforming into desired chemical compounds. Through depolymerization, lignin macromolecules with a molecular weight of tens of thousands Da can be degraded into lignin monomers, dimers and a few oligomers, which involves acid-catalyzed, alkali-catalyzed, oxidative, reductive, pyrolytic, and other reactions (Schutyser et al., 2018; Sun et al., 2018; Zhou et al., 2022). While the lignin structure undergoes a series of bond cleavage and functional group changes, the formed active intermediates are prone to repolymerization to form strong non-native C-C bonds, which poses a great challenge to improving the monomer yield (Shuai and Saha, 2017; Wang and Wang, 2019). In recent years, a series of catalytic and protection-group chemical methods have been proposed to stabilize the active lignin intermediates or inhibit their formation, thereby inhibiting the occurrence of condensation reactions and improving the depolymerization efficiency (Rinaldi et al., 2016; Questell-Santiago et al., 2020; Abu-Omar et al., 2021). However, so far, researchers have only a limited understanding of the lignin depolymerization process, and there is still much room for improvement in avoiding unfavorable side reactions and improving product selectivity (Wang et al., 2019).
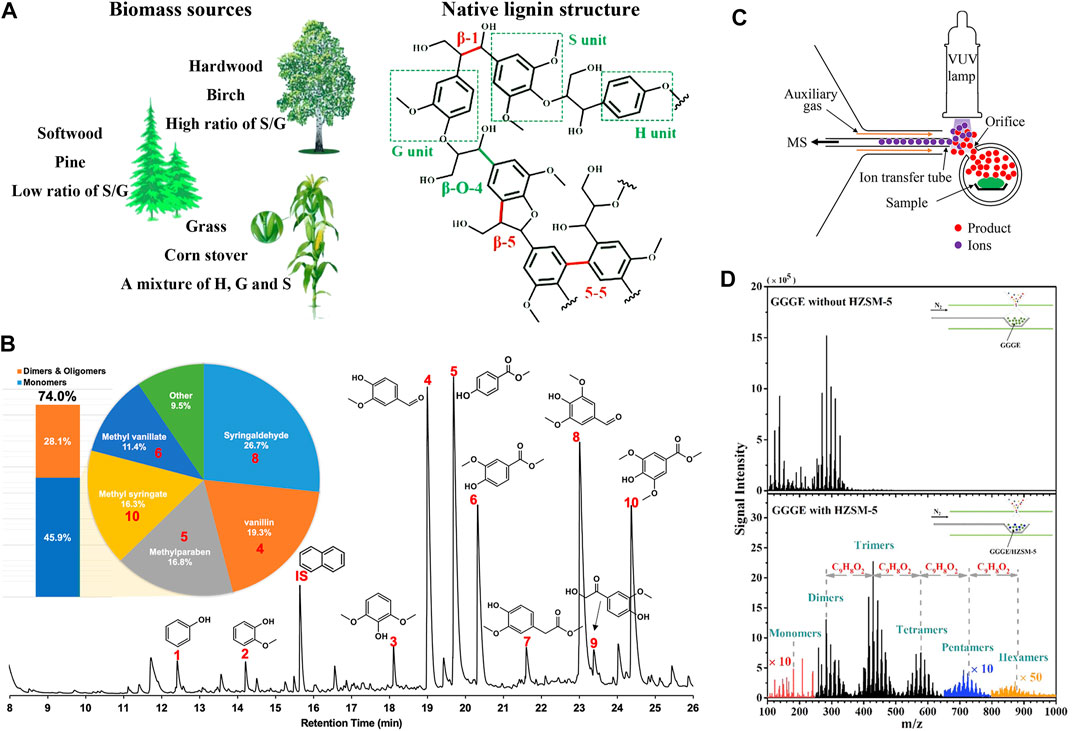
FIGURE 1. (A) Different sources and a representative structural fragment of native lignin, where H, G, and S units represent p-hydroxyphenyl, guaiacyl, and syringyl units, respectively. Reprinted from Cao et al. (2020), with permission from American Chemical Society (Copyright 2020). (B) Chromatogram of lignin depolymerization products which were identified by GC/MS and quantified by GC-FID, and yield of products with different degrees of polymerization. Reprinted from Du et al. (2021), with permission from American Chemical Society (Copyright 2021). (C) Experimental setup of in situ APPI MS method. Reprinted from Chen et al. (2020), with permission from American Chemical Society (Copyright 2019). (D) The comparison of mass spectra of guaiacylglycerol-β-guaiacyl ether (GGGE) pyrolysis with/without catalyst. Reprinted from Liu C. et al. (2020), with permission from Wiley-VCH GmbH (Copyright 2020).
The comprehensive assessment of the process products is the premise to grasp the reaction mechanism and further improve the conversion scheme, for which researchers have applied multiple analytical techniques. Chromatography is the preferred qualitative and quantitative method for gas and liquid products (Figure 1B) (Du et al., 2021). Typical separation methods such as gas chromatography (GC), liquid chromatography, and gel chromatography, combined with mass spectrometry (MS) and/or other detectors can conduct a detailed analysis of the products, giving the content, molecular formula, and partial structure information of each component (Kozliak et al., 2016; Letourneau and Volmer, 2020; Su et al., 2022). Through derivatization treatment and two-dimensional GC, complicated products can be better separated, and the qualitative and semi-quantitative identification of lignin monomers, dimers and trimers can be realized (Thi et al., 2022). Nuclear magnetic resonance and Fourier transform infrared spectroscopies are commonly employed in the structural characterization of liquid products and solid residues, which can provide information on chemical bonds and functionalities (Kozliak et al., 2016). However, these methods only allow for discontinuous analysis which takes quite a long time, and the information is either limited by molecular weight or lack of spectral resolution and sensitivity (Lupoi et al., 2015; Kozliak et al., 2016; Michailof et al., 2016). In the past decade, advanced mass spectrometry (MS) has gained much attention, for it can obtain the chemical formula of multiple products with molecular weight ranging from tens to tens of thousands Da simultaneously and intuitively, and monitor the evolution of reactive intermediates during the reaction with its fast detection speed, high sensitivity, and high resolution, as well as the development of soft ionization technology (Kubatova et al., 2020; Sun et al., 2022), which ensures the fragmentation controllable and reduces the difficulty of data interpretation.
Since the lignin depolymerization involves a series of complex reactions and generates various products including numerous aromatic monomers, dimers, and possibly cycloalkanes, furans, and oligomers with different chain lengths (Kozliak et al., 2016), whose states are transforming rapidly, conventional offline analyses are difficult to detect transient intermediates of the reaction, let alone the continuous changes in the composition and structure of lignin derivatives during the conversion process. To reveal the process of lignin depolymerization, online MS detection plays a vital role, and researchers have developed many online monitoring methods by innovating reactors, sampling methods, and ionization techniques for different conversion systems (Rueger et al., 2021; Sun et al., 2022). In the following, recent progress of process analysis of lignin depolymerization via online MS will be delivered separately from pyrolysis and liquefaction.
Thermal and catalytic pyrolysis of lignin
Pyrolysis is the thermal degradation of feedstocks into fuels and platform chemicals under an inert atmosphere, which acts as the first reaction of multiple thermal conversions, and is also a widely used thermal depolymerization method (Wang et al., 2017; Hoang et al., 2021). Lignin will transform into gaseous products, bio-oil, and char through pyrolysis, among which bio-oil is considered to be the main depolymerization product. The performance of depolymerization is closely related to the parameters such as pyrolysis temperature, heating rate, feedstock size, and residence time, as well as the choice of the catalytic system (Chen et al., 2019; Hoang et al., 2021).
Since lignin contains a rich diversity of linkages, its thermal decomposition covers a wide temperature range (Shen et al., 2015; Zhou et al., 2017). By directly connecting various reactors (including thermogravimetric (Zhou et al., 2017), fixed bed reactor (Le Brech et al., 2016), fluidized bed reactor (Jia et al., 2015), etc.) to photoionization (PI) MS via molecular beam nozzle or heated transfer lines, or integrating different furnaces into the ionization source (Dufour et al., 2013; Hurt et al., 2013; Zhu et al., 2020), researchers have realized online MS detection of pyrolysis volatiles, and thus observed the variation of the characteristic products with time and temperature intuitively. In general, lignin pyrolysis can be divided into two stages: 1) When the temperature is low, feedstocks will melt into liquid form, and the weaker ether bonds such as β-O-4 and α-O-4 will undergo heterolytic cleavage to form lignin monomers. 2) When the temperature increases, numerous C-O and C-C will be broken to generate more monomers and further reactions such as demethylation and demethoxylation will occur to form various phenols (Le Brech et al., 2016; Chen et al., 2019). Meanwhile, plenty of free radicals will be generated via homolysis of chemical bonds, which will further undergo rearrangement and repolymerization to form oligomers and even coke (Li and Takkellapati, 2018; Zhu et al., 2020).
Discussions on the primary pyrolysis mechanism of lignin have mainly focused on the radical mechanism and the concerted mechanism, which may vary with feedstock structure and reaction conditions and stages (Jarvis et al., 2011; Chen et al., 2019). Model compounds are frequently used to simplify the reaction system, with a concentration on the cleavage mechanism of β-O-4 and α-O-4. Pyrolysis of 2-phenethyl phenyl ether was conducted in a hyperthermal nozzle and analyzed via PIMS in real-time (Jarvis et al., 2011). The mass spectra obtained at different temperatures indicated that Maccoll and/or retro-ene eliminations were the dominant reaction pathways for the cleavage of β-O-4 at temperatures below 1000°C, which was consistent with subsequent experiments using in situ synchrotron vacuum ultraviolet (SVUV) PIMS (He et al., 2016) and pyroprobe-integrated atmospheric pressure chemical ionization MS (Sheng et al., 2017). While at higher temperatures and longer reaction time, the homolysis reaction to generate free radicals will gradually dominate, which will lead to the growth of aromatic hydrocarbons and the formation of coke (Zhu et al., 2020). In the pyrolysis experiment of α-O-4 model compounds, benzyl radical and phenoxy radical were detected by SVUV PIMS, which proved that the homolysis of Cα-O led to the cleavage of α-O-4 (He et al., 2016; Dai et al., 2019). Further, since the primary pyrolysis of lignin mainly produces monomers such as guaiacol, the online analysis of guaiacol pyrolysis was used to study the mechanism of the second-stage lignin pyrolysis reaction (Liu et al., 2018; Dai et al., 2019). The pathways for the removal of methyl, methoxyl, and other groups were revealed, and the formation of polycyclic aromatic hydrocarbons was detected, which has guiding significance for improving product selectivity and inhibiting repolymerization.
Due to the poor selectivity of thermal pyrolysis and the occurrence of repolymerization, the pyrolytic oil usually has a complicated composition with a low monomer yield (Schutyser et al., 2018). Therefore, a suitable catalytic system is vital for the lignin pyrolysis, among which zeolites have received the most extensive attention, for their unique pore structure and shape selectivity can stabilize the reactive intermediates and further convert the depolymerized monomers into target products (such as aromatics) (Jae et al., 2011; Schutyser et al., 2018; Chen et al., 2019). Recently, an online MS method coupled with in situ atmospheric pressure photoionization (APPI) was designed for monitoring the primary products of biomass pyrolysis (Figure 1C) (Chen et al., 2020), and further applied to the investigation of pyrolysis of guaiacylglycerol-β-guaiacyl ether catalyzed by HZSM-5 (Liu C. et al., 2021). A series of heavy phenolic oligomers were successfully detected (Figure 1D), elucidating the phenolic pool mechanism proposed previously (Stanton et al., 2018). In an online study of catalytic fast pyrolysis of biomass using a micro-fluidized bed, hierarchical zeolites were confirmed to have high selectivity for aromatics (Jia et al., 2017). To deepen the understanding of the mechanism, a temporal analysis of products reactor coupled with photoelectron photoion coincidence spectroscopy with SVUV was employed for isomer-selective detection of intermediates in the catalytic pyrolysis of guaiacol, and the core species in the reaction network, fulvenone ketene, was discovered for the first time (Hemberger et al., 2017). In addition, metal oxides, carbon-based catalysts, etc. also have the ability to improve lignin pyrolysis (Chen et al., 2019). For example, Pd/NC-catalyzed hydrodeoxygenation of guaiacol was proved to have high benzene selectivity (yield up to 85.1%) and stability (Liu C. J. et al., 2021).
Liquid-phase depolymerization of lignin
Liquid-phase depolymerization or liquefaction of lignin refers to the decomposition of lignin into lignin monomers, dimers, phenols, etc. in an aqueous or protic solvent environment, usually with specific catalysts, which requires a milder condition (200–400°C, 5–28 MPa) than lignin pyrolysis to achieve better depolymerization effect (Kozliak et al., 2016; Li and Takkellapati, 2018). Commonly used solvents include water, organic solvents like methanol, ethanol, acetone, tetralin, formic acid, and combinations of the above solvents (Kozliak et al., 2016; Schutyser et al., 2018), which are usually in a subcritical or even supercritical state under such reaction conditions, with excellent flow and transport properties, strengthening their interaction with solutes and providing great assistance for lignin depolymerization (Gillet et al., 2017). Monomer yields and product selectivity achieved by solvolytic depolymerization without catalysts tend to be low, and thus a suitable catalytic system, including acidic and basic catalysts, noble metals, and zeolites, is required (Kozliak et al., 2016; Schutyser et al., 2018). The depolymerization process is also greatly affected by the atmosphere of the system, commonly used are reductive and oxidative atmospheres. Reductive depolymerization often requires harsher conditions and may saturate aromatic rings, while oxidative depolymerization can cleave the inter-unit linkages of lignin under milder conditions and retain the aromatic rings to obtain phenolic products, but is not stable enough under oxidative conditions. An alternative method is a two-step strategy, in which β-O-4 is first weakened by selective oxidation, followed by reductive depolymerization under mild conditions (Schutyser et al., 2018; Liu et al., 2019). Moreover, the proposal of the lignin-first strategy has attracted extensive attention for reductive catalytic fractionation or direct hydrogenolysis of biomass using batch or flow-through reactors, which considerably inhibits the repolymerization and increases the monomer yields (Anderson et al., 2017; Liu et al., 2020; Abu-Omar et al., 2021).
However, since the systems of lignin liquefaction involve a variety of complicated feedstocks, require unconventional conditions and contain numerous side reactions, the current understanding of the overall reaction process is far from enough, and the theoretical maximum monomer yield is hard to reach. To better control the undesirable repolymerization of the reactive intermediates and the formation of tough C-C linkages, it is crucial to grasp the formation and consumption processes of key intermediates, which requires online monitoring during lignin liquefaction (Cui et al., 2022). For homogeneous catalytic reactions, soluble reactants, catalysts, and intermediates can be sampled simultaneously for MS analysis, whose online detection has been reported by several articles (Vikse et al., 2011; Yan et al., 2014). However, for the lignin conversion system, in order to facilitate the recovery of the catalyst and the separation of the products, heterogeneous catalytic reactions are usually preferred (Oregui-Bengoechea et al., 2019). Some researchers have used inductive ESI (Yan et al., 2014) and desorption ESI (Brown et al., 2015) methods, combined with pressured sample infusion coupling ambient MS to achieve online detection of heterogeneous catalytic reactions near atmospheric pressure. However, for the extreme conditions (high temperature and high pressure) required for lignin conversion, these methods are not applicable.
The greatest challenge in performing an online analysis of lignin thermal liquefaction is the undisturbed and stable sampling from the heterogeneous reaction zone under extreme conditions, which is a slurry mixture of catalysts, reactants, and products. Capillary sampling from the reaction system and transmission to the MS detector is a feasible approach, while the sampling area must be filtered to avoid capillary blockage, and the inner diameter and length of the capillary should be carefully selected to balance the pressure difference between the reaction area and the ionization zone. This idea was applied in the online investigation of catalytic hydrotreatment of guaiacol by coupling a batch reactor with PIMS and discovered the effect of hydrogen pressure on the selectivity of aromatics (Cui et al., 2021b). Most recently, an in situ reactor-integrated electrospray ionization (R-ESI) MS analysis method was proposed (Figure 2) (Cui et al., 2021a). The R-ESI source was integrated by applying high voltage to the micro-autoclave and assembling a fused-silica capillary with an inner diameter of 15 μm and a length of 60 mm to the bottom of the reactor as a sampling port, and meanwhile the spray needle. With this experimental setup, online studies on the catalytic hydrogenolysis of lignin were conducted, using methanol as a solvent, Pd/C or Ru/C as the catalyst, and the evolution of products distribution and typical monomers, dimers, and other oligomers during the reaction were discovered, with their structure elucidated by tandem MS. The progressive depolymerization of lignin and the repolymerization of active monomers were observed for the first time in experiments, and further, the lignin depolymerization mechanism was proposed (Cui et al., 2021a; Cui et al., 2022).
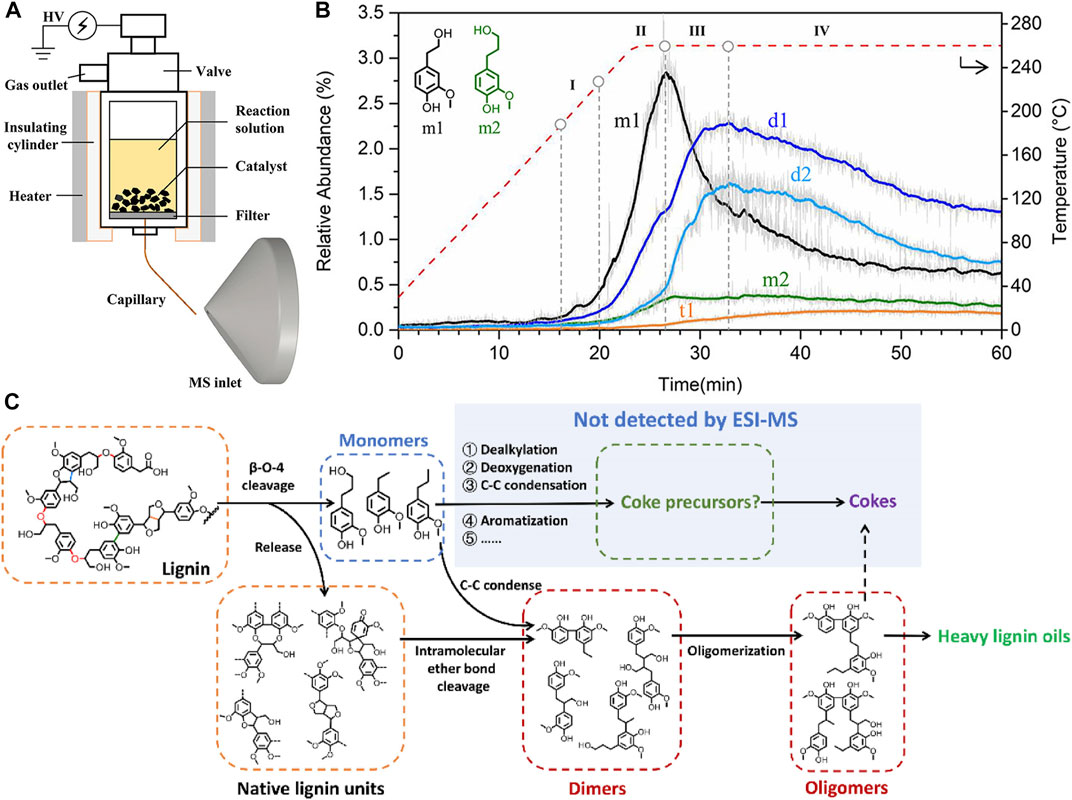
FIGURE 2. (A) A sketch of R-ESI MS. Reprinted from Cui et al. (2021a), with permission from American Chemical Society (Copyright 2021). (B) The evolution of typical phenolic products with different polymerization during the online liquid-phase detection,where m1 and m2 refer to two representative monomers, d1 and d2 are two dimers, and t1 is a trimer. Reprinted from Cui et al. (2021a), with permission from American Chemical Society (Copyright 2021). (C) General reaction pathways for lignin depolymerization. Reprinted from Cui et al. (2022), with permission from the authors (Copyright 2022).
Discussion
Thanks to the development of online soft ionization MS, complicated products of lignin pyrolysis and liquid-phase depolymerization have been successfully analyzed in real time. The detection of key intermediates, such as phenolic oligomers that cannot be detected with conventional methods, has promoted the fundamental understanding of the chemical process in the thermochemical depolymerization of lignin. Nevertheless, current knowledge is only the tip of the iceberg of the complex lignin depolymerization process. First, lignin resources are fairly extensive, including different types of virgin lignocellulose (Zhang et al., 2012; Zhou et al., 2016; Naron et al., 2019; Yuan et al., 2022) and technical lignin produced by industries such as papermaking (Gillet et al., 2017), all of which are complicated in composition and structure, without a complete description and relevant standards. Second, numerous reactions are involved in the depolymerization under harsh working conditions, including high temperature, high pressure, and heterogeneous environment, which results in the fast generation and consumption of numerous intermediates. The complexity of the reaction system poses great challenges for detection and analysis, while under the limitations of current soft ionization MS technologies, a full-molecular description of the lignin depolymerization is far from being achieved.
For qualitative analysis, probing intermediates under harsh conditions is an arduous task, and it is hard to detect all captured molecules using a single ionization method due to its certain preference for analytes (Kozliak et al., 2016; Stas et al., 2017b). Meanwhile, the structural analysis capability of the current online MS is very limited. Although different lignin units can be sequenced by MS/MS, precise matching of product formulas and structures is not possible due to the lack of a complete fragmentation database (Kiyota et al., 2012; Banoub et al., 2015). A database containing structural information of typical markers of lignin depolymerization products, especially the oligomers, is urgently needed. Moreover, due to the limitation of the resolution of the first-stage MS (usually quadrupole MS), the isolation of two closely adjacent peaks is tough to achieve. Coupled with the existence of isomerism, especially for macromolecular products that are the most concerned products of lignin depolymerization, the parent molecules with the same and/or similar molecular weights may contain a variety of structures, resulting in their fragment peaks jumbled together and difficult to interpret. For quantitative analysis, the sampling instability of online detection (Cui et al., 2021a), the difference in the response of ionization sources to analytes (Stas et al., 2017a), and the mass discrimination caused by mass analyzers (Smith et al., 2012) make MS data unable to directly serve as a quantitative standard. Due to the lack of standard samples corresponding to lignin depolymerization intermediates, especially reactive ones, accurate quantification via MS is challenging. Further, for online detection of heterogeneous systems under extreme working conditions, how to better connect the reactor with the detector and achieve in situ analysis closer to the actual process of the reaction is also an enormous challenge worth the efforts of researchers. The limitations of qualitative and quantitative analysis and online detection of MS indicate the direction of future technological development, which is not only helpful to deepen the understanding of details of lignin depolymerization, but also beneficial to the exploration of other catalytic reaction processes.
Author contributions
FQ and ZZ proposed the idea. LZ drafted the manuscript with support from CC and HL. LZ and ZZ discussed and revised the manuscript. All authors approved it for publication.
Funding
This work was supported by the National Key R&D Program of China (2019YFA0405600 and 2017YFA0402800).
Conflict of interest
The authors declare that the research was conducted in the absence of any commercial or financial relationships that could be construed as a potential conflict of interest.
Publisher’s note
All claims expressed in this article are solely those of the authors and do not necessarily represent those of their affiliated organizations, or those of the publisher, the editors and the reviewers. Any product that may be evaluated in this article, or claim that may be made by its manufacturer, is not guaranteed or endorsed by the publisher.
References
Abu-Omar, M. M., Barta, K., Beckham, G. T., Luterbacher, J. S., Ralph, J., Rinaldi, R., et al. (2021). Guidelines for performing lignin-first biorefining. Energy Environ. Sci. 14 (1), 262–292. doi:10.1039/d0ee02870c
Anderson, E. M., Stone, M. L., Katahira, R., Reed, M., Beckham, G. T., and Roman-Leshkov, Y. (2017). Flowthrough reductive catalytic fractionation of biomass. Joule 1 (3), 613–622. doi:10.1016/j.joule.2017.10.004
Banoub, J., Delmas, G. H., Joly, N., Mackenzie, G., Cachet, N., Benjelloun-Mlayah, B., et al. (2015). A critique on the structural analysis of lignins and application of novel tandem mass spectrometric strategies to determine lignin sequencing. J. Mass Spectrom. 50 (1), 5–48. doi:10.1002/jms.3541
Brown, T. A., Chen, H., and Zare, R. N. (2015). Identification of fleeting electrochemical reaction intermediates using desorption electrospray ionization mass spectrometry. J. Am. Chem. Soc. 137 (23), 7274–7277. doi:10.1021/jacs.5b03862
Cao, Y., Zhang, C., Tsang, D. C. W., Fan, J. J., Clark, J. H., and Zhang, S. C. (2020). Hydrothermal liquefaction of lignin to aromatic chemicals: Impact of lignin structure. Ind. Eng. Chem. Res. 59 (39), 16957–16969. doi:10.1021/acs.iecr.0c01617
Caspeta, L., Buijs, N. A. A., and Nielsen, J. (2013). The role of biofuels in the future energy supply. Energy Environ. Sci. 6 (4), 1077–1082. doi:10.1039/c3ee24403b
Chen, X., Che, Q. F., Li, S. J., Liu, Z. H., Yang, H. P., Chen, Y. Q., et al. (2019). Recent developments in lignocellulosic biomass catalytic fast pyrolysis: Strategies for the optimization of bio-oil quality and yield. Fuel Process. Technol. 196, 106180. doi:10.1016/j.fuproc.2019.106180
Chen, X. M., Zhu, L. Y., Cui, C. H., Zhu, Y. N., Zhou, Z. Y., and Qi, F. (2020). In situ atmospheric pressure photoionization mass spectrometric monitoring of initial pyrolysis products of biomass in real time. Anal. Chem. 92 (1), 603–606. doi:10.1021/acs.analchem.9b05200
Cui, C. H., Chen, X. M., Liu, C. J., Zhu, Y. A., Zhu, L. Y., Ouyang, J. F., et al. (2021a). In situ reactor-integrated electrospray ionization mass spectrometry for heterogeneous catalytic reactions and its application in the process analysis of high-pressure liquid-phase lignin depolymerization. Anal. Chem. 93 (38), 12987–12994. doi:10.1021/acs.analchem.1c02710
Cui, C. H., Liu, X. H., Zhou, C. Q., Liu, C. J., Zhou, Z. Y., and Qi, F. (2021b). Online study on the catalytic hydrotreatment of guaiacol in liquid phase by vacuum ultraviolet photoionization time-of-flight mass spectrometry. Energy fuels. 35 (17), 13863–13870. doi:10.1021/acs.energyfuels.1c02182
Cui, C., Zhu, L., Ouyang, J., Shen, Y., Ren, H., Yuan, W., et al. (2022). Online investigation of lignin depolymerization via reactor-integrated electrospray ionization high-resolution mass spectrometry. Appl. Energy Combust. Sci. 10, 100069. doi:10.1016/j.jaecs.2022.100069
Dai, G. X., Zhu, Y. N., Yang, J. Z., Pan, Y., Wang, G. Y., Reubroycharoen, P., et al. (2019). Mechanism study on the pyrolysis of the typical ether linkages in biomass. Fuel 249, 146–153. doi:10.1016/j.fuel.2019.03.099
Du, X., Tricker, A. W., Yang, W. S., Katahira, R., Liu, W., Kwok, T. T., et al. (2021). Oxidative catalytic fractionation and depolymerization of lignin in a one-pot single-catalyst system. ACS Sustain. Chem. Eng. 9 (23), 7719–7727. doi:10.1021/acssuschemeng.0c08448
Dufour, A., Weng, J. J., Jia, L. Y., Tang, X. F., Sirjean, B., Fournet, R., et al. (2013). Revealing the chemistry of biomass pyrolysis by means of tunable synchrotron photoionisation-mass spectrometry. RSC Adv. 3 (14), 4786–4792. doi:10.1039/c3ra40486b
Gillet, S., Aguedo, M., Petitjean, L., Morais, A. R. C., da Costa Lopes, A. M., Lukasik, R. M., et al. (2017). Lignin transformations for high value applications: Towards targeted modifications using green chemistry. Green Chem. 19 (18), 4200–4233. doi:10.1039/c7gc01479a
He, T., Zhang, Y. M., Zhu, Y. A., Wen, W., Pan, Y., Wu, J. L., et al. (2016). Pyrolysis mechanism study of lignin model compounds by synchrotron vacuum ultraviolet photoionization mass spectrometry. Energy fuels. 30 (3), 2204–2208. doi:10.1021/acs.energyfuels.5b02635
Hemberger, P., Custodis, V. B. F., Bodi, A., Gerber, T., and van Bokhoven, J. A. (2017). Understanding the mechanism of catalytic fast pyrolysis by unveiling reactive intermediates in heterogeneous catalysis. Nat. Commun. 8, 15946. doi:10.1038/ncomms15946
Hoang, A. T., Ong, H. C., Fattah, I. M. R., Chong, C. T., Cheng, C. K., Sakthivel, R., et al. (2021). Progress on the lignocellulosic biomass pyrolysis for biofuel production toward environmental sustainability. Fuel Process. Technol. 223, 106997. doi:10.1016/j.fuproc.2021.106997
Hurt, M. R., Degenstein, J. C., Gawecki, P., Borton, D. J., Vinueza, N. R., Yang, L., et al. (2013). On-line mass spectrometric methods for the determination of the primary products of fast pyrolysis of carbohydrates and for their gas-phase manipulation. Anal. Chem. 85 (22), 10927–10934. doi:10.1021/ac402380h
Jae, J., Tompsett, G. A., Foster, A. J., Hammond, K. D., Auerbach, S. M., Lobo, R. F., et al. (2011). Investigation into the shape selectivity of zeolite catalysts for biomass conversion. J. Catal. 279 (2), 257–268. doi:10.1016/j.jcat.2011.01.019
Jarvis, M. W., Daily, J. W., Carstensen, H. H., Dean, A. M., Sharma, S., Dayton, D. C., et al. (2011). Direct detection of products from the pyrolysis of 2-phenethyl phenyl ether. J. Phys. Chem. A 115 (4), 428–438. doi:10.1021/jp1076356
Jia, L. Y., Le-Brech, Y., Shrestha, B., Bente-von Frowein, M., Ehlert, S., Mauviel, G., et al. (2015). Fast pyrolysis in a microfluidized bed reactor: Effect of biomass properties and operating conditions on volatiles composition as analyzed by online single photoionization mass spectrometry. Energy fuels. 29 (11), 7364–7374. doi:10.1021/acs.energyfuels.5b01803
Jia, L. Y., Raad, M., Hamieh, S., Toufaily, J., Hamieh, T., Bettahar, M. M., et al. (2017). Catalytic fast pyrolysis of biomass: Superior selectivity of hierarchical zeolites to aromatics. Green Chem. 19 (22), 5442–5459. doi:10.1039/c7gc02309j
Kiyota, E., Mazzafera, P., and Sawaya, A. C. H. F. (2012). Analysis of soluble lignin in sugarcane by ultrahigh performance liquid chromatography-tandem mass spectrometry with a do-it-yourself oligomer database. Anal. Chem. 84 (16), 7015–7020. doi:10.1021/ac301112y
Kozliak, E. I., Kubatova, A., Artemyeva, A. A., Nagel, E., Zhang, C., Rajappagowda, R. B., et al. (2016). Thermal liquefaction of lignin to aromatics: Efficiency, selectivity, and product analysis. ACS Sustain. Chem. Eng. 4 (10), 5106–5122. doi:10.1021/acssuschemeng.6b01046
Kubatova, A., Andrianova, A. A., Hatton, J., and Kozliak, E. I. (2020). Atmospheric pressure ionization mass spectrometry as a tool for structural characterization of lignin. Rapid Commun. Mass Spectrom. 34 (14), e8813. doi:10.1002/rcm.8813
Laskar, D. D., Yang, B., Wang, H. M., and Lee, J. (2013). Pathways for biomass-derived lignin to hydrocarbon fuels. Biofuel. Bioprod. Biorefin. 7 (5), 602–626. doi:10.1002/bbb.1422
Le Brech, Y., Jia, L. Y., Cisse, S., Mauviel, G., Brosse, N., and Dufour, A. (2016). Mechanisms of biomass pyrolysis studied by combining a fixed bed reactor with advanced gas analysis. J. Anal. Appl. Pyrolysis 117, 334–346. doi:10.1016/j.jaap.2015.10.013
Letourneau, D. R., and Volmer, D. A. (2020). Mass spectrometry-based methods for the advanced characterization and structural analysis of lignin: A review. Mass Spectrom. Rev. doi:10.1002/mas.21716
Li, C. Z., Zhao, X. C., Wang, A. Q., Huber, G. W., and Zhang, T. (2015). Catalytic transformation of lignin for the production of chemicals and fuels. Chem. Rev. 115 (21), 11559–11624. doi:10.1021/acs.chemrev.5b00155
Li, T., and Takkellapati, S. (2018). The current and emerging sources of technical lignins and their applications. Biofuel. Bioprod. Biorefin. 12 (5), 756–787. doi:10.1002/bbb.1913
Liu, C., Chen, X., Liu, X., Cui, C., Zhou, Z., Jia, L., et al. (2021). Evidence of a phenolic pool as a key intermediate for zeolite-catalyzed lignin pyrolysis. Angew. Chem. Int. Ed. 60 (5), 2643–2647. doi:10.1002/anie.202011937
Liu, C. J., Ye, L. L., Yuan, W. H., Zhang, Y., Zou, J. B., Yang, J. Z., et al. (2018). Investigation on pyrolysis mechanism of guaiacol as lignin model compound at atmospheric pressure. Fuel 232, 632–638. doi:10.1016/j.fuel.2018.05.162
Liu, C. J., Zhou, C. Q., Wang, Y., Liu, X. H., Zhu, L. Y., Ma, H., et al. (2021). Gas-phase hydrodeoxygenation of bio-oil model compound over nitrogen-doped carbon-supported palladium catalyst. Proc. Combust. Inst. 38 (3), 4345–4353. doi:10.1016/j.proci.2020.06.156
Liu, C., Wu, S., Zhang, H., and Xiao, R. (2019). Catalytic oxidation of lignin to valuable biomass-based platform chemicals: A review. Fuel Process. Technol. 191, 181–201. doi:10.1016/j.fuproc.2019.04.007
Liu, X., Bouxin, F. P., Fan, J., Budarin, V. L., Hu, C., and Clark, J. H. (2020). Recent advances in the catalytic depolymerization of lignin towards phenolic chemicals: A review. Chemsuschem 13 (17), 4296–4317. doi:10.1002/cssc.202001213
Lupoi, J. S., Singh, S., Parthasarathi, R., Simmons, B. A., and Henry, R. J. (2015). Recent innovations in analytical methods for the qualitative and quantitative assessment of lignin. Renew. Sustain. Energy Rev. 49, 871–906. doi:10.1016/j.rser.2015.04.091
Michailof, C. M., Kalogiannis, K. G., Sfetsas, T., Patiaka, D. T., and Lappas, A. A. (2016). Advanced analytical techniques for bio-oil characterization. WIREs. Energy Environ. 5 (6), 614–639. doi:10.1002/wene.208
Naron, D. R., Collard, F. X., Tyhoda, L., and Gorgens, J. F. (2019). Influence of impregnated catalyst on the phenols production from pyrolysis of hardwood, softwood, and herbaceous lignins. Industrial Crops Prod. 131, 348–356. doi:10.1016/j.indcrop.2019.02.001
Oregui-Bengoechea, M., Agirre, I., Iriondo, A., Lopez-Urionabarrenechea, A., Requies, J. M., Agirrezabal-Telleria, I., et al. (2019). Heterogeneous catalyzed thermochemical conversion of lignin model compounds: An overview. Top. Curr. Chem. 377 (6), 36. doi:10.1007/s41061-019-0260-5
Questell-Santiago, Y. M., Galkin, M. V., Barta, K., and Luterbacher, J. S. (2020). Stabilization strategies in biomass depolymerization using chemical functionalization. Nat. Rev. Chem. 4 (6), 311–330. doi:10.1038/s41570-020-0187-y
Ragauskas, A. J., Beckham, G. T., Biddy, M. J., Chandra, R., Chen, F., Davis, M. F., et al. (2014). Lignin valorization: Improving lignin processing in the biorefinery. Science 344 (6185), 1246843. doi:10.1126/science.1246843
Ragauskas, A. J., Williams, C. K., Davison, B. H., Britovsek, G., Cairney, J., Eckert, C. A., et al. (2006). The path forward for biofuels and biomaterials. Science 311 (5760), 484–489. doi:10.1126/science.1114736
Rinaldi, R., Jastrzebski, R., Clough, M. T., Ralph, J., Kennema, M., Bruijnincx, P. C. A., et al. (2016). Paving the way for lignin valorisation: Recent advances in bioengineering, biorefining and catalysis. Angew. Chem. Int. Ed. 55 (29), 8164–8215. doi:10.1002/anie.201510351
Rueger, C. P., Tiemann, O., Neumann, A., Streibel, T., and Zimmermann, R. (2021). Review on evolved gas analysis mass spectrometry with soft photoionization for the chemical description of petroleum, petroleum-derived materials, and alternative feedstocks. Energy fuels. 35 (22), 18308–18332. doi:10.1021/acs.energyfuels.1c02720
Schutyser, W., Renders, T., Van den Bosch, S., Koelewijn, S. F., Beckham, G. T., and Sels, B. F. (2018). Chemicals from lignin: An interplay of lignocellulose fractionation, depolymerisation, and upgrading. Chem. Soc. Rev. 47 (3), 852–908. doi:10.1039/c7cs00566k
Shen, D. K., Liu, G. F., Zhao, J., Xue, J. T., Guan, S. P., and Xiao, R. (2015). Thermo-chemical conversion of lignin to aromatic compounds: Effect of lignin source and reaction temperature. J. Anal. Appl. Pyrolysis 112, 56–65. doi:10.1016/j.jaap.2015.02.022
Sheng, H., Murria, P., Degenstein, J. C., Tang, W., Riedeman, J. S., Hurt, M. R., et al. (2017). Initial products and reaction mechanisms for fast pyrolysis of synthetic G-lignin oligomers with beta-O-4 linkages via on-line mass spectrometry and quantum chemical calculations. Chemistryselect 2 (24), 7185–7193. doi:10.1002/slct.201700582
Shuai, L., and Saha, B. (2017). Towards high-yield lignin monomer production. Green Chem. 19 (16), 3752–3758. doi:10.1039/c7gc01676j
Smith, E. A., Park, S., Klein, A. T., and Lee, Y. J. (2012). Bio-oil analysis using negative electrospray ionization: Comparative study of high-resolution mass spectrometers and phenolic versus sugaric components. Energy fuels. 26 (6), 3796–3802. doi:10.1021/ef3003558
Stanton, A. R., Iisa, K., Mukarakate, C., and Nimlos, M. R. (2018). Role of biopolymers in the deactivation of ZSM-5 during catalytic fast pyrolysis of biomass. ACS Sustain. Chem. Eng. 6 (8), 10030–10038. doi:10.1021/acssuschemeng.8b01333
Stas, M., Chudoba, J., Auersvald, M., Kubicka, D., Conrad, S., Schulzke, T., et al. (2017a). Application of orbitrap mass spectrometry for analysis of model bio-oil compounds and fast pyrolysis bio-oils from different biomass sources. J. Anal. Appl. Pyrolysis 124, 230–238. doi:10.1016/j.jaap.2017.02.002
Stas, M., Chudoba, J., Kubicka, D., Blazek, J., and Pospisil, M. (2017b). Petroleomic characterization of pyrolysis bio-oils: A review. Energy fuels. 31 (10), 10283–10299. doi:10.1021/acs.energyfuels.7b00826
Su, S. H., Xiao, L. P., Chen, X., Wang, S. Z., Chen, X. H., Guo, Y. Z., et al. (2022). Lignin-first depolymerization of lignocellulose into monophenols over carbon nanotube-supported ruthenium: Impact of lignin sources. Chemsuschem 15, e202200365. doi:10.1002/cssc.202200365
Sun, J. H., Yin, Y. Y., Li, W. X., Jin, O. Y., and Na, N. (2022). Chemical reaction monitoring by ambient mass spectrometry. Mass Spectrom. Rev. 41 (1), 70–99. doi:10.1002/mas.21668
Sun, Z. H., Fridrich, B., de Santi, A., Elangovan, S., and Barta, K. (2018). Bright side of lignin depolymerization: Toward new platform chemicals. Chem. Rev. 118 (2), 614–678. doi:10.1021/acs.chemrev.7b00588
Thi, H. D., Van Aelst, K., Van den Bosch, S., Katahira, R., Beckham, G. T., Sels, B. F., et al. (2022). Identification and quantification of lignin monomers and oligomers from reductive catalytic fractionation of pine wood with GC × GC – FID/MS. Green Chem. 24 (1), 191–206. doi:10.1039/d1gc03822b
Vikse, K. L., Ahmadi, Z., Manning, C. C., Harrington, D. A., and McIndoe, J. S. (2011). Powerful insight into catalytic mechanisms through simultaneous monitoring of reactants, products, and intermediates. Angew. Chem. Int. Ed. 50 (36), 8304–8306. doi:10.1002/anie.201102630
Wang, H. L., Pu, Y. Q., Ragauskas, A., and Yang, B. (2019). From lignin to valuable products-strategies, challenges, and prospects. Bioresour. Technol. 271, 449–461. doi:10.1016/j.biortech.2018.09.072
Wang, M., and Wang, F. (2019). Catalytic scissoring of lignin into aryl monomers. Adv. Mat. 31 (50), 1901866. doi:10.1002/adma.201901866
Wang, S. R., Dai, G. X., Yang, H. P., and Luo, Z. Y. (2017). Lignocellulosic biomass pyrolysis mechanism: A state-of-the-art review. Prog. Energy Combust. Sci. 62, 33–86. doi:10.1016/j.pecs.2017.05.004
Wang, T. P., Li, H., Yuan, J. M., Li, W. X., Li, K., Huang, Y. B., et al. (2021). Structures and pyrolytic characteristics of organosolv lignins from typical softwood, hardwood and herbaceous biomass. Industrial Crops Prod. 171, 113912. doi:10.1016/j.indcrop.2021.113912
Yan, X., Sokol, E., Li, X., Li, G., Xu, S., and Cooks, R. G. (2014). On-line reaction monitoring and mechanistic studies by mass spectrometry: Negishi cross-coupling, hydrogenolysis, and reductive amination. Angew. Chem. Int. Ed. 53 (23), 5931–5935. doi:10.1002/anie.201310493
Yuan, J. M., Li, H., Xiao, L. P., Wang, T. P., Ren, W. F., Lu, Q., et al. (2022). Valorization of lignin into phenolic compounds via fast pyrolysis: Impact of lignin structure. Fuel 319, 123758. doi:10.1016/j.fuel.2022.123758
Zhang, M., Resende, F. L. P., Moutsoglou, A., and Raynie, D. E. (2012). Pyrolysis of lignin extracted from prairie cordgrass, aspen, and Kraft lignin by Py-GC/MS and TGA/FTIR. J. Anal. Appl. Pyrolysis 98, 65–71. doi:10.1016/j.jaap.2012.05.009
Zhou, C. H., Xia, X., Lin, C. X., Tong, D. S., and Beltramini, J. (2011). Catalytic conversion of lignocellulosic biomass to fine chemicals and fuels. Chem. Soc. Rev. 40 (11), 5588–5617. doi:10.1039/c1cs15124j
Zhou, N., Thilakarathna, W. P. D. W., He, Q. S., and Rupasinghe, H. P. V. (2022). A review: Depolymerization of lignin to generate high-value bio-products: Opportunities, challenges, and prospects. Front. Energy Res. 9, 758744. doi:10.3389/fenrg.2021.758744
Zhou, S., Xue, Y., Sharma, A., and Bai, X. L. (2016). Lignin valorization through thermochemical conversion: Comparison of hardwood, softwood and herbaceous lignin. ACS Sustain. Chem. Eng. 4 (12), 6608–6617. doi:10.1021/acssuschemeng.6b01488
Zhou, Z. Y., Jin, H. F., Zhao, L., Wang, Y. Z., Wen, W., Yang, J. Z., et al. (2017). A thermal decomposition study of pine wood under ambient pressure using thermogravimetry combined with synchrotron vacuum ultraviolet photoionization mass spectrometry. Proc. Combust. Inst. 36 (2), 2217–2224. doi:10.1016/j.proci.2016.06.081
Zhu, J. L., Yang, H., Hu, H. Q., Zhou, Y., Li, J. G., and Jin, L. J. (2020). Novel insight into pyrolysis behaviors of lignin using in-situ pyrolysis-double ionization time-of-flight mass spectrometry combined with electron paramagnetic resonance spectroscopy. Bioresour. Technol. 312, 123555. doi:10.1016/j.biortech.2020.123555
Keywords: lignin depolymerization, mass spectrometry, soft ionization, process analysis, online detection
Citation: Zhu L, Cui C, Liu H, Zhou Z and Qi F (2022) Thermochemical depolymerization of lignin: Process analysis with state-of-the-art soft ionization mass spectrometry. Front. Chem. Eng. 4:982126. doi: 10.3389/fceng.2022.982126
Received: 30 June 2022; Accepted: 25 July 2022;
Published: 16 August 2022.
Edited by:
Costas Tsouris, Oak Ridge National Laboratory (DOE), United StatesReviewed by:
Ling-Ping Xiao, Dalian Polytechnic University, ChinaCopyright © 2022 Zhu, Cui, Liu, Zhou and Qi. This is an open-access article distributed under the terms of the Creative Commons Attribution License (CC BY). The use, distribution or reproduction in other forums is permitted, provided the original author(s) and the copyright owner(s) are credited and that the original publication in this journal is cited, in accordance with accepted academic practice. No use, distribution or reproduction is permitted which does not comply with these terms.
*Correspondence: Haoran Liu, bGl1aGFvcmFuOTVAc2p0dS5lZHUuY24=; Zhongyue Zhou, Wmhvbmd5dWUuemhvdUBzanR1LmVkdS5jbg==