- 1Clinical Translational Center for Targeted Drug, Department of Pharmacology, School of Medicine, Jinan University, Guangzhou, China
- 2Institution of Laboratory Animal, Jinan University, Guangzhou, China
- 3School of Food Science and Engineering, South China University of Technology, Guangzhou, China
- 4Enantiotech Corp., Ltd., Zhongshan Torch Hi-Tech, Industrial Development Zone, Zhongshan, China
Antibacterial resistance is by far one of the greatest challenges to global health. Many pharmaceutical or material strategies have been explored to overcome this dilemma. Of these, silver nanoparticles (AgNPs) are known to have a non-specific antibacterial mechanism that renders it difficult to engender silver-resistant bacteria, enabling them to be more powerful antibacterial agents than conventional antibiotics. AgNPs have shown promising antibacterial effects in both Gram-positive and Gram-negative bacteria. The aim of this review is to summarize the green synthesis of AgNPs as antibacterial agents, while other AgNPs-related insights (e.g., antibacterial mechanisms, potential toxicity, and medical applications) are also reviewed.
1 Introduction
Along with the long-term use of antibiotics for bacterial treatment, bacteria evolve in order to survive, leading to bacterial drug resistance (McManus, 1997; Munita et al., 2015). Bacterial resistance is a symptom of bacteria becoming resistant to previous effective antibiotics. Bacterial resistance is a growing threat to global public health since patients with drug-resistant bacterial infections have worse clinical outcomes, face a higher risk of death (Antoniadou et al., 2007), and consume more healthcare resources than patients with non-drug-resistant bacterial infections. For example, Staphylococcus aureus (MRSA) is a common cause of serious infections in health facilities and communities (David and Daum, 2010), whereas resistance to the first-line drugs used to treat these infections is universal. Estimated survival rates for patients with methicillin-resistant MRSA infections are 64% lower than for uninfected patients (Sabbagh et al., 2019). Whilst the emergence of bacterial resistance is a natural phenomenon, other main reasons for speeding up the spread of bacterial resistance including the lack of hygienic measures to prevent and control infections, the excessive and inappropriate use of antibiotics.
As a result, many proactive strategies for bacterial resistance have been proposed, including calling for rational use of antibiotics (Solomon and Oliver, 2014), strengthening health systems and regulatory capacity (Courtenay et al., 2019), and tapping into new antibiotics and other antibacterial drugs (World Health, 2012). AgNPs are currently gaining widespread attention as antibacterial agents (Alt et al., 2004; Silver et al., 2006) as it is very difficult for AgNPs to generate bacterial resistance. Also, it is crucial to manufacture nanomaterials in a safe, environmentally friendly and economical manner for further clinical translational applications. Among the many preparation methods for AgNPs, green synthesis with environmentally friendly catch our attention. Here, we overviewed the green preparation methods of AgNPs, including saccharide-based, irradiation-reduction and biosynthesis methods, and gave a systematic comparison of the advantages and disadvantages of these three methods. We also introduced the antibacterial mechanisms and potential side effects of AgNPs for balancing the efficacy and toxic effects of AgNPs in antibacterial therapy. Lastly, a summary of their medical applications is presented, reflecting their potential medical applications.
2 Preparation of AgNPs by green synthesis method
2.1 Saccharide-based method
Compared with traditional methods for the preparation of AgNPs, the glycosylation method as the first emerging green preparation strategy demonstrates many advantages. Water is used as environmentally friendly solvent throughout the synthesis process, and sugars are used as reducing and stabilizing agents, which fully embodies the concept of green chemistry. In addition, the weak binding interaction of release of silver and is suitable for biomedical applications.
Raveendran and others (Raveendran et al., 2003; Raveendran et al., 2006) first reported a green method for preparing AgNPs (Figure 1A). Silver nitrate (AgNO3) and starch were dissolved in water, and β-d-glucose was added and the reaction was stirred at 40°C for 20 h. Starch and β-d-glucose functioned as stabilizing and reducing agent, respectively. The reaction conditions were mild and no organic solvents or toxic substances were involved. The mixture turned light yellow indicating the formation of AgNPs. The absorption maximum was at 419 nm (Figure 1B) due to the surface plasmon resonance of AgNPs and the size of AgNPs was around 10 nm (Figures 1C,D). In addition, many saccharide-based methods were then developed to synthesize AgNPs, including exploiting different polysaccharides, optimizing the concentration of silver salts and polysaccharides and optimizing the reaction conditions. For example, Many different polysaccharides including heparin (Huang and Yang, 2004), sucrose (Lee S. H. et al., 2014), corn starch (Valodkar et al., 2010) and cellulose (Mohammad et al., 2022) were also explored as reducing and stabilizing agents for the synthesis of AgNPs (Table 1). Vigneshwaran et al. (Vigneshwaran et al., 2006) used starch to obtain stable AgNPs by optimizing the resugars with AgNPs facilitates the action conditions. The mixture was incubated in an autoclave at a pressure of 15 psi and a temperature of 121°C for 5 min. Tai et al. (Tai et al., 2008) obtained small and homogeneous AgNPs using a rotating disc reactor. Subsequently, higher quality AgNPs were gradually obtained by adjusting the starch concentration, AgNO3 concentration and reactor parameters. Besides, several studies have developed the usage of polymers as stabilizers, such as poly (ethylene glycol) (PEG) (Luo et al., 2005; Shameli et al., 2012). The chain length of the polymer affected the reaction rate and the size of AgNPs, specifically, longer polymer chains (e.g., PEG 2000) have higher reactivity than PEG 200 or ethylene glycol. Meanwhile, the large amount of oxygen in the longer PEG chain provided the coordination saturation of the dangling bonds on the surface of the AgNPs, helping to prevent the agglomeration of AgNPs and obtain stable AgNPs (Luo et al., 2005).
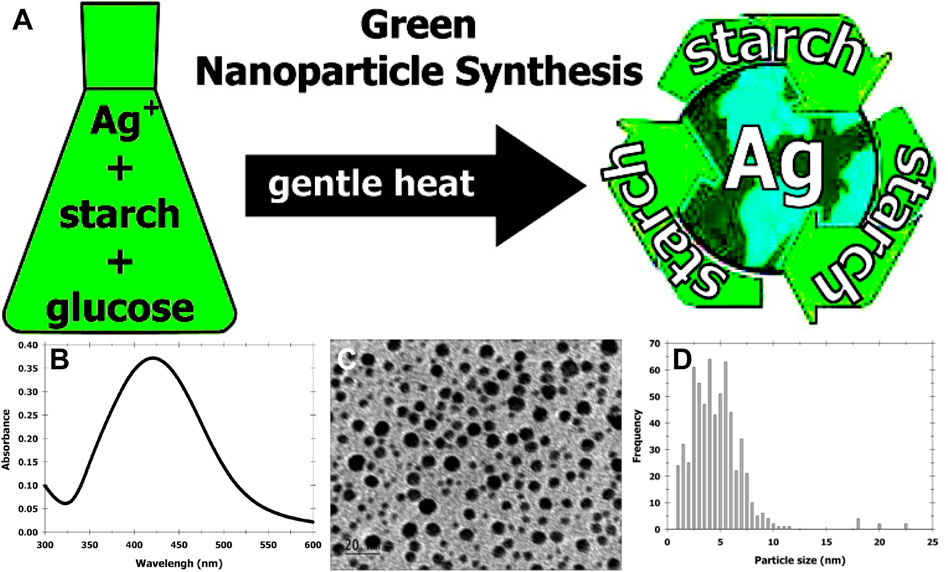
FIGURE 1. (A) Schematic of completely “green” synthesis of AgNPs. (B) The surface-plasmon absorbance spectrum of AgNPs formed in the aqueous starch dispersion (λmax = 419 nm). (C) Typical TEM image of starched AgNPs. The scale bar corrresponds to 20 nm. (D) Histogram showing the size distribution of the AgNPs (the average particle size = 5.3 nm and σ = 2.6 nm). The total number of particles counted for the histogram is 624. Reproduced with permission from ref (Vigneshwaran et al., 2006). Copyright 2003 American Chemical Society.
2.2 Irradiation-reduction method
Irradiation reduction is another method for green preparation of AgNPs. This method does not require additional reducing agents, and the reaction rate induced by irradiation can be clearly defined, which facilitates the control of the reaction process. At the same time, complete and homogeneous AgNPs can be obtained when prepared by this method (Zhang et al., 2003), avoiding the cumbersome post-treatment to remove unreacted silver ions.
The main mechanism for the preparation of AgNPs by irradiation reduction is that water is decomposed by irradiation to produce hydrated electrons, which subsequently reduce silver ions to silver and thus promote the formation of silver clusters (Karim et al., 2007; Long et al., 2007). Therefore, this method generally requires the addition of a cluster stabilizer to prevent silver agglomeration caused by direct irradiation reduction (Shin et al., 2004). The main process for synthesis of AgNPs by irradiation reduction was first to dissolve a certain amount of AgNO3 and biocompatible macromolecules as stabilizer in water, such as amphiphilic polymers (Zhou et al., 1999; Zhang et al., 2003) or natural polysaccharides (Chen et al., 2007). Subsequently, the mixed solution was degassed with nitrogen bubbling for about 30 min, sealed and irradiated at a certain dose at room temperature. Related studies focus on the optimization of radioactive sources and the selection of stabilizers. A variety of radioactive sources can be used to prepare AgNPs, including ultraviolet light (Zhou et al., 1999), visible light (Zhang et al., 2003; Zhang et al., 2010), microwaves (Seku et al., 2018), and high-energy rays (Karim et al., 2007; Lee et al., 2007) (Table 2). Among them, microwaves as a radiation source can greatly reduce the synthesis time because microwaves provide uniform nucleation and growth conditions for nanoparticles (Chen et al., 2008; Hu et al., 2008). In addition, light emitting diode (LED) as radiation sources can control the size, morphology and optical properties of AgNPs. Stamplecoskie et al. (Stamplecoskie and Scaiano, 2010) explored the differences in the size, morphology and optical properties of AgNPs obtained for radiation at wavelength of 405 nm, 455 nm, 627 nm and 720 nm (Figure 2). Under irradiation with 405 nm LED, the particle size of AgNPs gradually increased with irradiation time (Figure 2B). Irradiation of AgNP seeds with a 455 nm LED induced spectral changes, and subsequently transformed their morphology into dodecahedra with narrower polydispersity (Figure 2C). In contrast, AgNPs with a larger distribution of nanoplates and nanorods were obtained with 627 and 720 nm light irradiation (Figures 2D,E). Further, the radiation dose also affects the physicochemical properties of AgNPs. Chen et al. discussed the effect of radiation dose on the size distribution (Chen et al., 2007). They used chitosan as a stabilizer, which degraded into small fragments upon γ-irradiation, and then its interaction with silver through amino chelation prevented silver agglomeration. They found that a slightly lower irradiation dose (∼27 kGy) produced AgNPs with a narrower particle size distribution, while a slightly higher irradiation dose (∼75 kGy) produced AgNPs with a wider particle size distribution (Chen et al., 2007). Liu et al. proposed the concept of AgNPs with “clean” surfaces (no surfactant or polymer contamination) and obtained the desired clean AgNPs by adjusting the γ-irradiation dose (Liu et al., 2007). This would be a great advantage for no other reagents involved during the preparation, which complying with the concept of green chemistry.
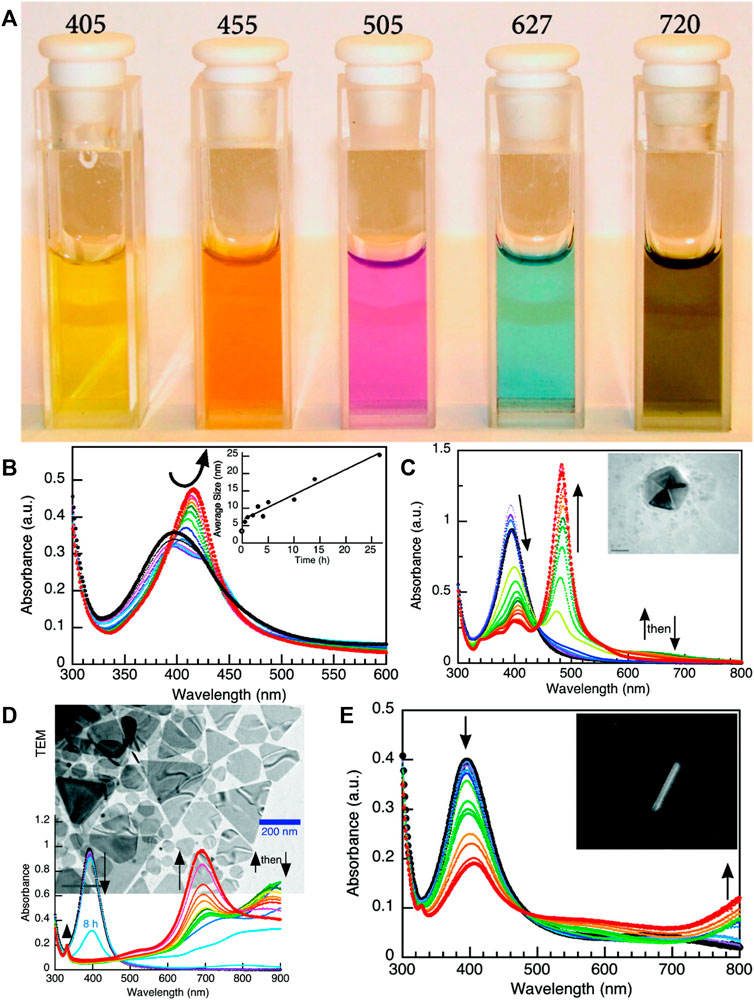
FIGURE 2. (A) Image of the various colloidal solutions produced under the corresponding LED irradiation at the wavelength indicated (in nm) at the top of each cuvette. (B) UV-vis spectral change upon 405 nm irradiation (initial in black, final in red) and (inset) change in particle size (determined by scanning electron microscopy (SEM)) against irradiation time. (C) UV-vis spectral change during 455 nm LED excitation (initial in black, final in red); note that at ∼650 nm the maximum absorbance increases and then decreases at intermediate times (green) during conversion. The inset shows TEM image for a representative particle (size bar = 20 nm). (D) UV-vis spectral change and TEM image (background) during 627 nm LED excitation of AgNP seeds with ∼900 nm absorption increase and then decrease during the overall conversion. (E) UV-vis spectral change during 720 nm LED irradiation as well as an exceptionally large aspect ratio nanorod (inset). Reproduced with permission from ref (Stamplecoskie and Scaiano, 2010). Copyright 2010 American Chemical Society.
2.3 Biosynthesis method
Biosynthesis of AgNPs has been extensively studied as an emerging preparation strategy (Table 3). Biosynthesis is a very environmentally friendly process because it does not involve high temperature, high pressure, energy consumption and toxic chemicals, which gives it a great advantage over conventional synthesis methods. The general process of biosynthesis is to isolate the desired raw material from a natural resource and boil it, after which the bioactive components are extracted and then incubated with a silver ion solution to produce AgNPs.
2.3.1 Microorganism
Microorganisms are commonly used for the biosynthesis of AgNPs, including bacteria (Lateef et al., 2015; Wang et al., 2016; Saravanan et al., 2018; Ameen et al., 2020), fungi (Guilger-Casagrande et al., 2019; Hamad, 2019; Hu et al., 2019), and algae (Sinha et al., 2015; Muthusamy et al., 2017; Massironi et al., 2019). Microbial-based biosynthesis strategies are generally classified into intracellular and extracellular synthesis. In principle, AgNPs with more uniform size and shape distribution can be obtained by intracellular synthesis, but the collection and post-processing of the products are relatively cumbersome and expensive. Therefore, most studies on the biosynthesis of AgNPs have focused on the extracellular pattern.
Silver-resistant bacteria are the main microorganisms for microbial-based synthesis of AgNPs. A bacterial strain-Weissella oryzae DC6, isolated from mountain ginseng, has been first used for green and convenient synthesis of AgNPs, the secreted proteins and enzymes are responsible for the reduction of silver ions (Singh et al., 2016). Gandhi and others (Gandhi and Khan, 2016) also synthesized AgNPs via Escherichia coli, incubation of silver ions in the supernatant of Escherichia coli leaded to the extracellular reduction of metal ions and the formation of AgNPs (Figure 3). In addition, synthesis of AgNPs in fungi offers many advantages, as fungi grows rapidly and can secrete large amounts of enzymes, which producing abundant raw material for the synthesis. Furthermore, fungi can withstand the agitation and flow pressures of bioreactor. Laryssa et al. first reported that AgNPs were synthesized extracellularly using nematophagous fungus Duddingtonia flagrans. They obtained the cell-free fungal filtrates from Duddingtonia flagrans, and analyzed the total protein content and chitinase activity in the filtrates, which could act as a reducing agent for the synthesis of AgNPs. This method produced high yield of AgNPs with good stability (Costa Silva et al., 2017). Singh et al. prepared AgNPs on the endophytic fungus Alternaria sp. Isolated from healthy leaves of Raphanus sativus, which showed effective antibacterial effect against human pathogenic bacteria (Singh et al., 2017).
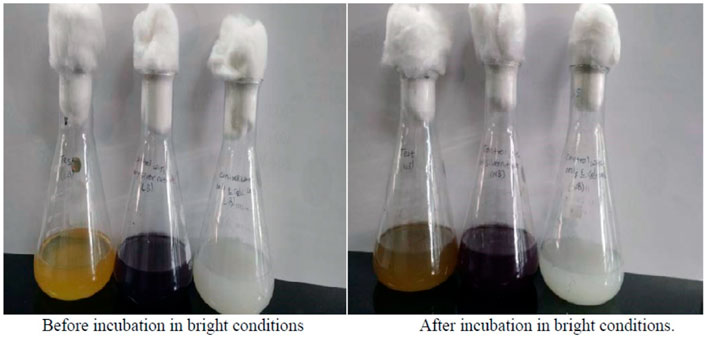
FIGURE 3. Color change from yellow to brown after incubation with Escherichia coli indicates presence of silver nanoparticles. Reproduced with permission from ref (Gandhi and Khan, 2016). Copyright 2016 Elsevier.
Microbial-based method for the preparation of AgNPs has many advantages, as it is a complete green reaction process without using industrial chemical reagents, and simple operations with low energy consumption. In addition, the prepared AgNPs are naturally coated with proteins secreted by biomass, showing high stability and excellent biocompatibility for further applications (Chowdhury et al., 2014).
2.3.2 Plants
Plant-mediated reduction systems have also been widely investigated due to their simplicity, eco-friendly and the potential medicinal value of the plants themselves (Sengottaiyan et al., 2016a; Sengottaiyan et al., 2016b; Escárcega-González et al., 2018; Wang et al., 2018; S.S et al., 2019). A major advantage of using plant extracts for synthesis of AgNPs is that complex cell culture processes can be avoided, facilitating the post-processing of the products and their further industrial application (Sudhakar et al., 2015). The biomolecules in plant extracts can act as both reducing agents and stabilizer during AgNPs formation and can even exert their own antibacterial effect (Ghorbani et al., 2015).
Wang et al. synthesized AgNPs using aqueous extracts from Psidium guajava L. They demonstrated that AgNPs could be formed in 10 min after the mix of AgNO3 and extracts, and the reaction was basically completed after 2 h (Figures 4A,B) (Wang et al., 2018). The reduction rate of plant-based synthesis was significantly improved compared to biosynthetic methods based on fungi, bacteria, etc., which require about 24 h to obtain large amounts of AgNPs. Also, they found that the resulting AgNPs had excellent antibacterial effects against both common Gram-positive and Gram-negative bacteria (Figure 4C) (Wang et al., 2018). Selvam et al. used Tinospora cordifolia (Thunb.) Miers for eco-friendly synthesis of AgNPs. They studied the influence factors (AgNO3, leaf, incubation time, and pH) by response surface methodology of Box--Behnken design (BBD) to optimize synthesis conditions. Under optimal conditions, the silver ions were reduced to AgNPs within 30 min by heating (60 °C) of T. cordifolia extract mixed with silver ions (Selvam et al., 2017). In addition to plant leaves, flowers have also been studied for the biosynthesis of AgNPs (Aravinthan et al., 2015; Chinnappan et al., 2018; Ameen et al., 2019), bioactive components extracted from flowers can also exert antimicrobial effects. Aravinthan and others reported a rapid green synthesis of AgNPs using an aqueous extract of Helianthus tuberosus (sunroot tuber). The ability of the biomolecules extracted from tuber reducing Ag+ in solution was confirmed by the stretching vibrations of amines and alkaloids observed by fourier transform infrared spectroscopy (FTIR). They also investigated the antibacterial activity of AgNPs synthesized from tuber extracts against phytopathogenic bacteria, namely, R. solanacearum and X. axonopodis, and the results showed that the tuber extracts synergistically enhanced the antibacterial properties of AgNPs against the phytopathogenic bacteria (Aravinthan et al., 2015). Mango flower extract was also used as a bio-reducing agent for the synthesis of AgNPs. The obtained AgNPs were effective against Gram-negative bacteria Klebsiella sp., P. agglomerans, and Rahnella sp. At 10 mM of AgNPs (Ameen et al., 2019). In addition, other abundant seaweed extracts have also been developed for the synthesis of AgNPs, such as Spyridia filamentosa (Valarmathi et al., 2020), Caulerpa racemose (Kathiraven et al., 2015) and Gracilaria birdiae (de Aragão et al., 2019). The wide source of plants and their easy availability, and some plants possess antibacterial activity, all these contribute to the beneficial prospect of this method.
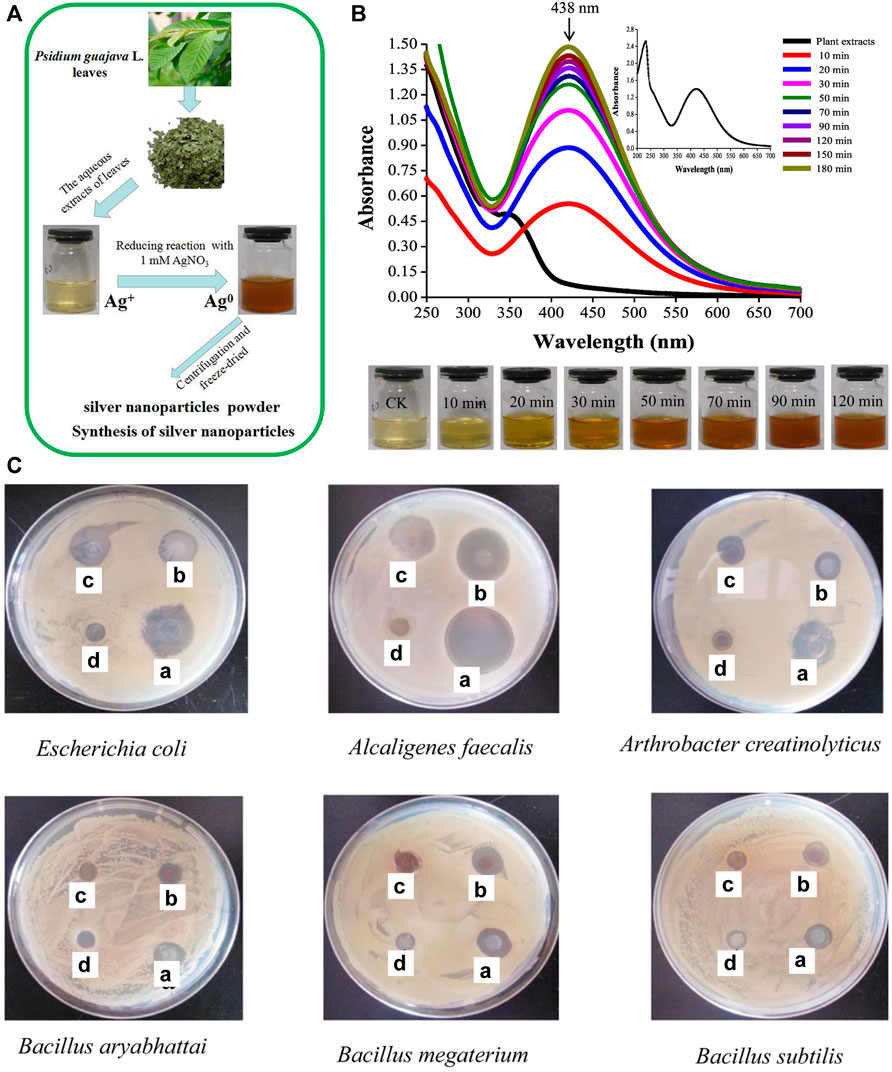
FIGURE 4. (A) Schematic of synthesis of AgNPs using P. guajava L. leaf extracts. (B) UV-vis spectra of the bioreduction kinetics in the range of 200–700 nm for a colloidal AgNO3 solution with P. guajava L. leaf extracts; the inset upper right is the UV-vis spectra of P. guajava L. leaf extracts and the inset below shows the solution color changes over time. CK: the aqueous extracts of P. guajava L. leaf. (C) Activity of P-AgNPs formed by the reduction of AgNO3 with aqueous extracts from P. guajava L. leaves against selected bacterials depicting zones of inhibition of (a) positive control-ampicillin, (b) P-AgNPs, (c) AgNO3 control, (d) P. guajava leaf aqueous extracts. Reproduced with permission from ref (Wang et al., 2018). Copyright 2018 Elsevier.
2.3.3 Food and agricultural waste
The development of food and agricultural waste for the synthesis of AgNPs provides a sustainable way to effectively utilize the waste. In recent years, cow milk (Williams et al., 2022), coffee extracts (Chien et al., 2019), vegetable oilcake (Singhal and Gupta, 2019), peels (Soto et al., 2019), wood (Xue et al., 2018) and other agricultural industrial wastes (Mythili et al., 2018) have been widely developed for the synthesis of AgNPs.
Economical and readily available milk was reported to synthesize AgNPs, and the presence of proteins in milk may be responsible for the reduction of Ag+. TEM results showed that AgNPs mainly existed in the form of aggregates, which might be caused by the presence of lipids in milk (Lee et al., 2013). However, this problem could be controlled by changing the reaction parameters, such as pH, temperature and reactant concentration (Nguyen et al., 2013). Due to reducing active ingredient chlorogenic acid (CGA), green coffee bean extracts have also been developed for the synthesis of AgNPs (Wang et al., 2017). Govarthanan et al. (Govarthanan et al., 2014) used a traditional Indian agricultural formulation panchakavya, a mixture of microorganisms, to synthesize AgNPs without any contamination. Coconut (Cocos nucifera) oil cake (COC) is a by-product that extracts oil from the dried copra. It contains starch, soluble sugar, protein, lipid and trace nitrogen, the reducing components of which can also be used for the synthesis of AgNPs (Govarthanan et al., 2016). And plant waste Sal deoiled seed cake (DOC) can also be used to extract AgNPs from discarded X-ray sheets (Singhal and Gupta, 2019). In addition, the synthesis of AgNPs using vegetable waste extracts from the market has also been reported (Mythili et al., 2018). The raw materials for this method are all waste, representing a promising sustainable route. This synthetic route is “green” in that: 1) waste is used as a resource for the synthesis of AgNPs, 2) non-critical environmental synthesis conditions make it energy-efficient and cost-effective, and 3) no organic solvents are involved, making it environmentally friendly and economical (Devadiga et al., 2015).
In short, several environmentally friendly green methods for the synthesis of AgNPs are presented in this section, including saccharide-based method, irradiation reduction method and biosynthesis method. The saccharide-based method as the first emerged green synthesis does not involve environmentally unfriendly materials in the whole process. However, as a preliminary attempt, there are some disadvantages, such as the need for high temperature and pressure, and the unclean surface of the obtained AgNPs, requiring suitable post-treatment for their further applications. While irradiation-reduction method shows some advantages, such as the high controllability of the reaction process. It allows the obtained AgNPs to be controlled in size and morphology, and even to achieve a completely clean surface, which is great beneficial for further applications. But it has special requirements for the equipment and the reaction process is more tedious. Specifically, the reducing and stabilizing agents used in the biosynthesis process come from nature, which are widely available and easily accessible for mass production. Among them, plant-mediated synthesis could significantly increase the reaction rate, and own medicinal value of extracted plants might be synergistic with AgNPs for efficient antibacterial purposes. While the food and agricultural waste method reflects the concept of economic benefits of waste utilization and sustainable development, which is very compatible with the concept of green synthesis. Altogether, each of these green synthesis methods has its own advantages and can be chosen specifically according to the purpose of application of AgNPs.
3 Antibacterial mechanisms of AgNPs
The resistance of bacteria to antibiotics is based on three general mechanisms (Kumarasamy et al., 2010; Wilson, 2014; Blair et al., 2015): 1) production of enzymes that degrade drugs, 2) alteration of drug targets, and 3) reduction of the permeability of bacterial cell membranes to drugs. Unlike the antibacterial mechanism of traditional antibiotics, the unique antibacterial mechanism of AgNPs effectively avoid the occurrence of bacterial resistance.
3.1 Release of silver ions from AgNPs
Many hypotheses have been proposed for the antibacterial mechanism of AgNPs, presenting that AgNPs are transformed into silver ions after entering bacterial cells and exert antibacterial effects by interacting with various intracellular biomolecules. For example, silver ions can bind to sulfhydryl enzymes within bacteria, thereby denaturing the enzymes, which are necessary for the normal metabolism of antibacterial drugs (Liau et al., 1997; McDonnell and Russell, 1999). Silver ions can also bind to the DNA of bacteria, changing the conformation of DNA, causing dysfunctional DNA and exerting antibacterial effects (Feng et al., 2000; Arakawa et al., 2001). In addition, silver ions can mediate the release of potassium ions from microbial plasma (Russell and Hugo, 1994b; Holt and Bard, 2005). It has also been reported that silver ions are associated with elevated intracellular ROS levels (Park et al., 2009). The interference of silver ions with the respiratory chain of bacteria increases the production of ROS and exhibits efficient bactericidal activity.
3.2 AgNPs-mediated destructive effect on bacterial membranes
Apart from releasing silver ions for antibacterial activity, AgNPs can also perform antibacterial functions by directly disrupting bacterial membranes and then penetrating to microorganisms, as evidenced by the forming “pits” on the membrane surface after treating with AgNPs (Sondi and Salopek-Sondi, 2004; Choi et al., 2008). Nevertheless, the size and shape of AgNPs have a significant effect on their ability to bind to bacterial membranes. AgNPs with {111} facets had been reported to interact directly with the bacterial surface (Morones et al., 2005), while the truncated triangular AgNPs with {111} lattice plane showed stronger bactericidal effects than other shape structures, such as spheres (Pal et al., 2007).
3.3 Other antibacterial mechanisms of AgNPs
Additional antibacterial mechanisms of AgNPs have been reported. For exsample, Kalishwaralal et al. (Kalishwaralal et al., 2010) explored the potential anti-biofilm activity of AgNPs with Pseudomonas aeruginosa and Staphylococcus epidermidis, which were the source of many chronic bacterial infections. More than 95% of biofilm formation were inhibited by treating these bacteria with AgNPs, resulting in inhibition of bacterial growth. The other well mentioned antibacterial mechanism is that AgNPs may induce an apoptosis-like response with bacteria (Lee W. et al., 2014), including phosphatidylserine externalization (early apoptosis) and DNA damage (late apoptosis) (Bao et al., 2015).
Taken together, AgNPs are different from conventional antibiotics that can trigger bacterial resistance. AgNPs are acting in a new antibacterial paradigm that contribute to breaking the dilemma of antibiotic-induced bacterial resistance.
4 Potential toxicity
AgNPs-containing products are widely used in daily life. Human being may be exposed to AgNPs-containing products in different ways (inhalation, skin contact and ingestion), thus unconsciously taking in heavy metal compounds and causing potential harm to the body. Ji et al. (Ji et al., 2007) exposed rats to certain concentrations of silver and showed no significant changes in lung tissue after 28 days, according to the current American Conference of Governmental Industrial Hygienists (ACGIH) silver dust limit (100 μg/m3). Park et al. (Park et al., 2007) studied the cytotoxicity of AgNPs in alveolar epithelial cells and found that even at high concentrations of AgNPs (200 μg/ml), the apoptosis rate was less than 12% and the degree of DNA fragmentation was less than 2%, confirming the relatively low toxicity of AgNPs to the lung. From these works, it can be concluded that the effect of AgNPs on the lung is negligible, probably due to the high atomic mass of Ag. The effect of AgNPs on the skin has also been investigated. The cytotoxicity of AgNPs-containing antimicrobial wound dressings was evaluated with human epidermal keratin-forming cells and human fibroblasts, and it was found that AgNPs could not distinguish between healthy cells and pathogenic bacteria involved in wound healing and had a certain degree of cytotoxicity (Lam et al., 2004; Poon and Burd, 2004; Paddle-Ledinek et al., 2006; Arora et al., 2008; Samberg et al., 2010).
The liver and kidneys are the main organs that take up and metabolize nanoparticles, so it is crucial to assess the effects of AgNPs on these organs. To that end, the BRL 3A immortal rat liver cells were incubated with AgNPs for 24 h. Hepatocytes showed increased leakage of lactate dehydrogenase (LDH) and mitochondrial dysfunction, displaying marked cytotoxicity (Figure 5) (Hussain et al., 2005). And the depletion of reduced glutathione (GSH) in hepatocytes suggests that hepatotoxicity is related to oxidative stress, which is one of the antibacterial mechanisms of AgNPs. Cytotoxicity of AgNPs to HepG2 human hepatoma cells at high concentrations (>1 mg/L), but no apparent cytotoxicity below that concentration (Kawata et al., 2009). Moreover, gender differences in renal silver accumulation have been reported (Kim et al., 2008). Female rats showed large accumulation of AgNPs in all regions of the kidney (cortex, outer medulla and inner medulla), and the accumulation of AgNPs in cortical glomeruli was obviously higher in females than in males (Kim et al., 2009). These relevant studies illustrate the tendency of silver to accumulate in the liver and kidneys with toxic effects. There are also studies reported that AgNPs could accumulate in the brain and exhibit neurotoxicity. Tang et al. found that AgNPs could cross the blood-brain barrier. Long-term exposure to AgNPs might lead to neuronal lesions and necrosis (Tang et al., 2008). Lee et al. (Lee et al., 2010) investigated the effect of AgNPs on gene expression in the mouse brain using affymetrix mouse genome arrays and found that 468 genes in the brain and 952 genes in the cerebellum were sensitive to AgNPs. Given the potential hazards of AgNPs to humans, we need to take a critical view of the antibacterial activity and potential toxicity of AgNPs. It should prescribe appropriate doses for administration according to different therapeutic purposes. Importantly, the size and morphology of AgNPs can be modulated, their surface can be optimized modification to reduce cytotoxicity and enhance therapeutic effects.
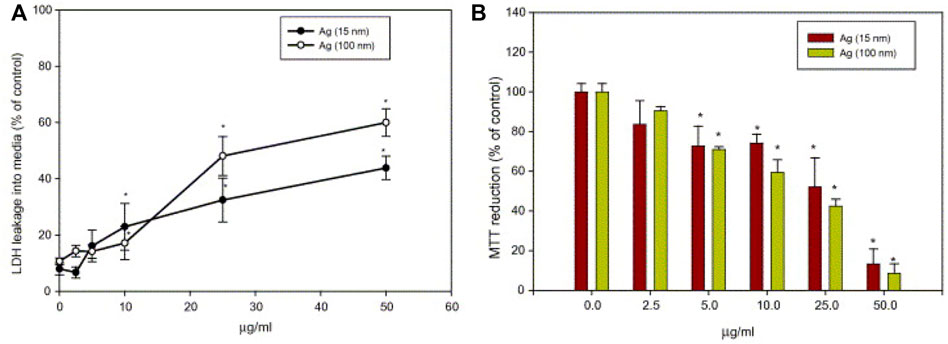
FIGURE 5. (A) Effect of AgNPs on LDH leakage in rat liver cells BRL 3A cells. (B) Effect of nanoparticles on mitochondrial function in rat liver cells (BRL 3A cells). Cells were treated with different concentrations of AgNPs for 24 h. Reproduced with permission from ref (Hussain et al., 2005). Copyright 2005 Elsevier.
5 Medical application of AgNPs
As described above, it is difficult for AgNPs to develop resistance to antimicrobial therapy because silver resistance requires a generation of bacteria to undergo three independent mutations in three different bacterial systems (Alt et al., 2004; Silver et al., 2006). Owing to the unique advantages, AgNPs are often employed as antimicrobial agents in medical applications to ward off infections (Table 4).
5.1 Wound dressing
Blisters repeatedly appear during wound healing after deep burns, which are prone to ulcerate and infect to form residual wounds (Huang et al., 2007). Additionally, local wounds are classified into acute and chronic wounds according to their nature and recovery time. Chronic trauma is a fertile ground for biofilm formation, which is one of the causes of bacterial resistance (Sacco et al., 2015). Over the past decades, AgNPs have been extensively studied in wound healing, and although silver is relatively inert and difficult to absorb by mammalian or bacterial cells, it is readily ionized by wound fluid or other secretions. When bound to proteins and cell membranes, ionized silver becomes highly active, inhibiting biofilm formation and wound infection (Atiyeh et al., 2007). Silver sulfadiazine has been considered the gold standard for the treatment of local burns, but subsequent studies have found that it delays the wound healing process and is accompanied by severe cytotoxicity (Russell and Hugo, 1994a; Atiyeh et al., 2007). Actually, ideal wound dressing should meet the following requirements: good mechanical strength and breathability, excellent exudate absorption, blood and cell compatibility, etc. AgNPs-containing antibacterial wound dressings prepared by electrostatic spinning and in situ reduction of surface silver ions using biocompatible macromolecules such as polymers (Hong et al., 2006; GhavamiNejad et al., 2015; GhavamiNejad et al., 2016; Unnithan et al., 2016; Augustine et al., 2018) and biomacromolecules (Lu et al., 2008; Madhumathi et al., 2010; Singh and Singh, 2014; Biswas et al., 2018; Wu et al., 2018) as substrates can achieve good therapeutic effects. For example, a wound dressing consisting of AgNPs and chitosan was prepared by self-assembly, which passed sterility and pyrogenic safety evaluations in tests with deeper thick wound Sprague-Dawley rat model (Lu et al., 2008). Further, MADO-AgNPs prepared by coating AgNPs on a novel electrospun nanofiber material, poly (methyl methacrylate-dopamine methacrylamide, MADO), exhibited good antibacterial activity in vitro and good wound healing ability in vivo (Figure 6) (GhavamiNejad et al., 2015).
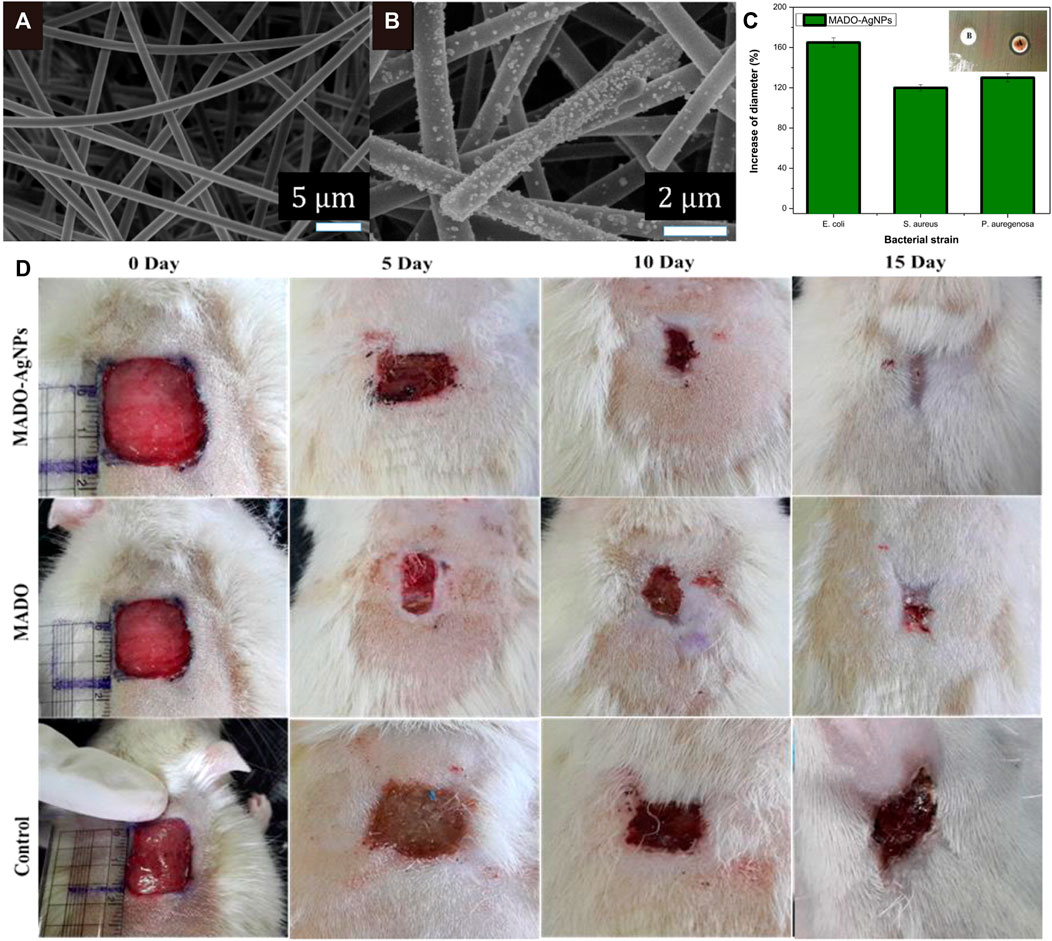
FIGURE 6. (A) and (B) Field emission scanning electron microscopy (FESEM) images of MADO nanofibers and MADO-AgNPs nanofibers. (C) Results of the antibacterial activity of MADO-AgNPs electrospun membranes against Pseudomonas aeruginosa, Staphylococcus aureus, and Escherichia coli. The inset shows a comparison of (A) MADO-AgNPs nanofiber and (B)MADO nanofiber on a Lysogeny broth (LB)-agar plate covered with Pseudomonas aeruginosa. (D) Wound appearance at 0, 5, 10, and 15 days after grafting with MADO-AgNPs, MADO nanofiber, and bare. Reproduced with permission from ref (GhavamiNejad et al., 2015). Copyright 2015 American Chemical Society.
5.2 Implants
Implants are widely used in clinical treatment, but since they are exogenous materials, they tend to trigger an immune response in the body and expose patients to infections. Bacterial infections of implants are usually caused by Staphylococci, as the bacteria tend to adhere to the surface of the implants, forming biofilm and inducing infection (van de Belt et al., 2001). The use of high-dose antibiotics to prevent implant infection during transplantation has been attempted in the clinic, but the action duration is limited (Oliveira et al., 2018). Therefore, it is an urgent need to develop implants that are resistant to bacteria. A potential strategy is to deposit antimicrobial substances on the surface of the implant. AgNPs are excellent antibacterial agents with drug-resistant Staphylococci, widely deposited on the implant surface to avoid infection. To date, implants surface deposited with AgNPs have focused on various medical devices, such as heart valves (Grunkemeier et al., 2006; Ghanbari et al., 2009; de Mel et al., 2012), bone graft devices (Zheng et al., 2010; Travan et al., 2011; Zhao et al., 2011; DeVasConCellos et al., 2012; van Hengel et al., 2020) and orthopedic implants (Liu et al., 2012; Fordham et al., 2014; Huang et al., 2017). For example, Andara et al. developed a multi-component target pulse laser deposition process to prepare a diamond-like carbon-silver composites and validated their promising hemocompatibility as a coating for cardiovascular implants (Andara et al., 2006). Liu et al. reported AgNPs/poly (DL-lactic-co-glycolic acid)-coated stainless steel alloy (SNPSA) as a potential antibacterial implant material that exhibited strong antibacterial activity in vitro and in vivo without interfering with bone morphogenetic protein 2 (BMP-2) for bone formation (Figure 7) (Liu et al., 2012).
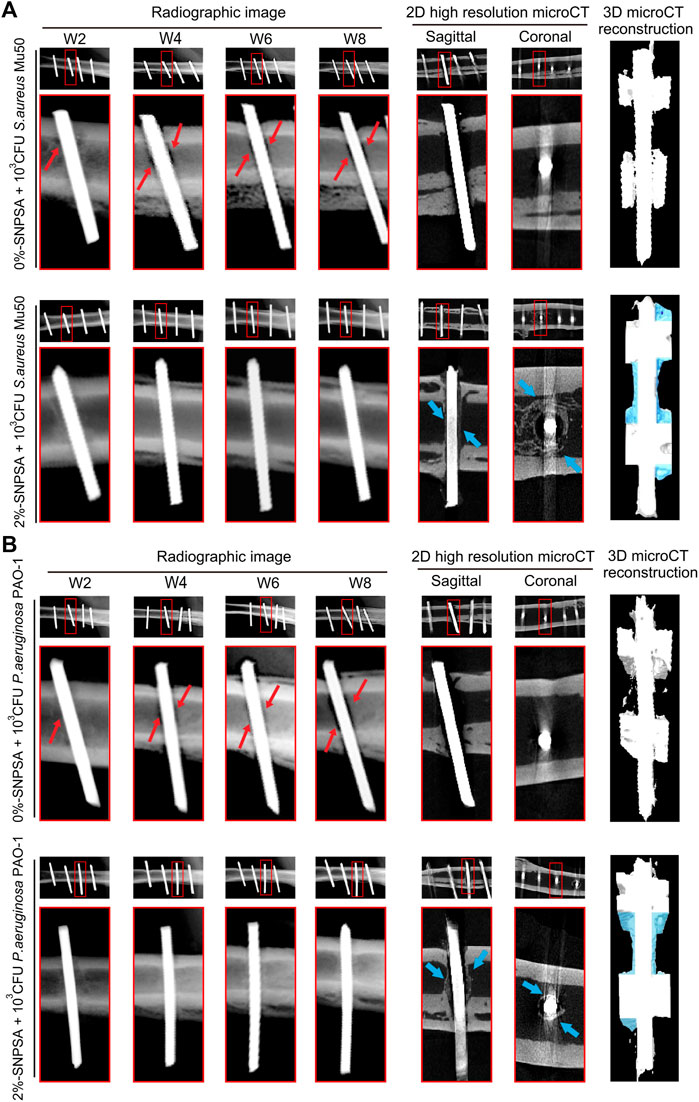
FIGURE 7. Radiographic images of contaminated 0 %- and 2 %-SNPSA implants in rat femoral canal (FC) model. 103 CFU S. aureus Mu50 (A) or P. aeruginosa PAO-1 (B) in 10 μL PBS (105 CFU/ml) was pipetted into the canal before implantation for bacterial invasion. Radiographic evidence of osseous destruction (red arrows), without any obvious signs of bone formation up to 8 weeks post-surgery, was detected in the contaminated 0%-SNPSA group. In contrast, significant bone formation surrounding 2%-SNPSAs implanted in rat FCs at week eight post-implantation (shown as blue arrows in 2D resolution micro-computed tomography (microCT) images), without significant osteolysis, was detected. Newly formed bone around 2%-SNPSA implants was highlighted in 3D microCT reconstruction images (blue shading). Reproduced with permission from ref (Liu et al., 2012). Copyright 2012 Elsevier.
5.3 Medical catheters
The risk of chronic catheterization-related infections is extremely high, such as catheter-associated urinary tract infections (Thokala et al., 2018), intravascular infections (Hsu et al., 2010; Paladini et al., 2013) and cerebrospinal fluid infections (Lackner et al., 2008). AgNPs could also be utilized to coat catheters destined for clinical care. Roe et al. demonstrated that surfactant-modified AgNPs coated on the surface of catheters can reduce the risk of infectious complications in patients with indwelling catheters by continuously releasing sterilized silver at the implantation site (Roe et al., 2008). Besides, Zhang et al. developed a silver-tetrafluoroethylene nanocomposite coating with catheters by simple wet chemical method, which was able to decrease biofilm coverage up to 97.4% compared to commercial silicone tubes (Zhang et al., 2019). The colonization of fucose-functionalized silver nanoparticles (FNPs) on urinary catheters revealed superior biofilm resistance and antibacterial effect on silicone rubber compared to citrate-encapsulated silver nanoparticles (CNPs), attributed to their strong attachment capacity with bacterial and penetrating into bacterial cells (Figure 8) (Bhargava et al., 2018).
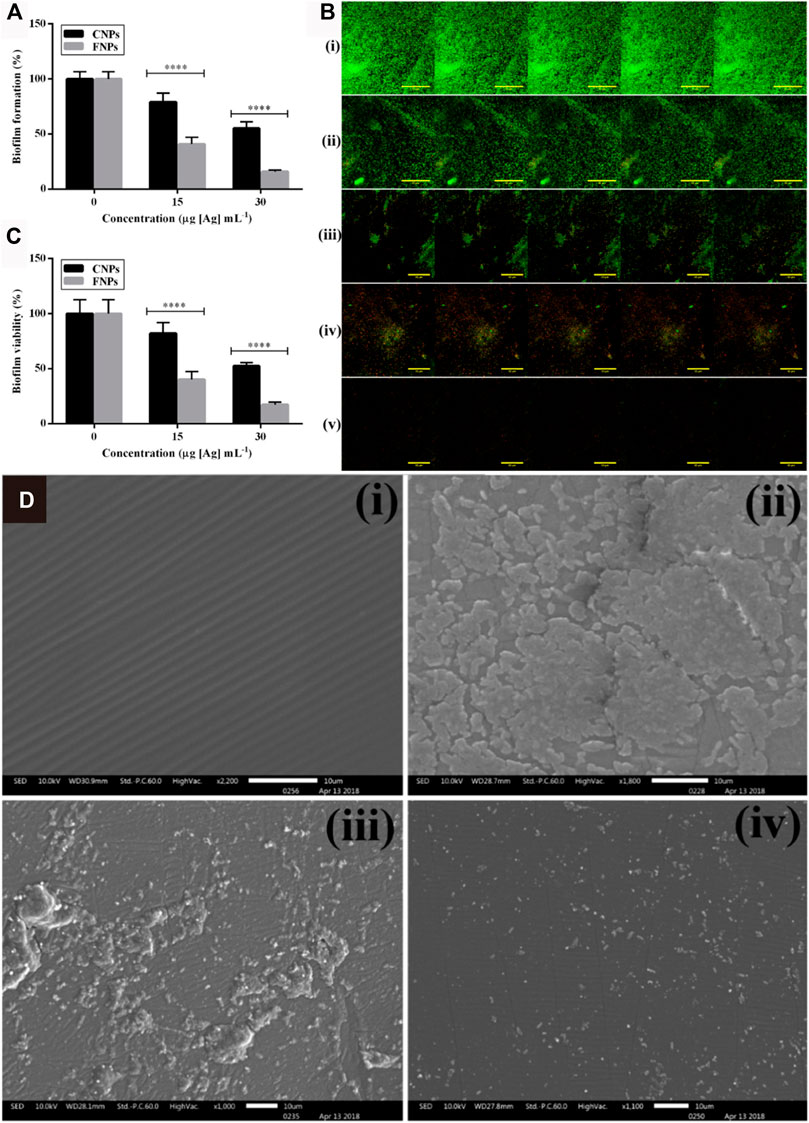
FIGURE 8. (A) Biofilm formation by bacterial cells exposed to CNPs and FNPs assessed by Crystal violet (CV) staining assay (n = 8). (B) Composite confocal laser scanning microscopy (CLSM)-stacked image of live/dead stained biofilm formed by cells exposed to CNPs and FNPs: i) Untreated/control, ii) 15 μg [Ag] mL−1 CNPs, iii) 15 μg [Ag] mL−1 FNPs, iv) 30 μg [Ag] mL−1 CNPs, and v) 30 μg [Ag] mL−1 FNPs. (C) Effect of CNPs and FNPs on the bacterial viability in established biofilm. (D) SEM imaging of the silicone rubber disc: i) blank, ii) control, iii) CNP- and (iv) FNP-loaded discs at a concentration of 80 μg [Ag] g−1. Reproduced with permission from ref (Bhargava et al., 2018). Copyright 2018 American Chemical Society.
5.4 Dental composites
Streptococcus mutans is the main microorganism that causes tooth decay. Initial adhesion of specific oral bacteria to the tooth surface or artificial dental matrix is a prerequisite for the formation of pathogenic biofilms (Magalhães et al., 2012). Dental modification materials should preferentially manifest antimicrobial properties at an early stage in order to inhibit the adhesion and proliferation of pathogens. For this purpose, the incorporation of AgNPs into dental bone cements or silver plating on their surface can generate an antibacterial effect (Akhavan et al., 2013; Chladek et al., 2013; Ai et al., 2017; Chambers et al., 2017). A report evaluated the antibacterial activity of three AgNPs-modified dental bone cements (Sealapex, RelyX ARC and Vitrebond) and noticed that the antibacterial activity of Vitrebond was enhanced by the addition of AgNPs (Magalhães et al., 2012). With the aim of improving biocompatibility, a surface modification of AgNPs-coated titanium implants with hydroxyapatite was developed, showing optimum antimicrobial capacity and favorable biosafety (Salaie et al., 2020).
Evidently, AgNPs are currently widely used in clinical applications due to their unique antibacterial properties. Not only can they be used for antibacterial treatment of traumatic surfaces, but they can even be applied to materials such as implants and medical devices for the prevention of bacterial infections.
6 Conclusion
At a time when antibiotic resistance is rampant around the world, AgNPs are being extensively invented for their antimicrobial effects. Here, we systematically state the green method to prepare AgNPs for a sustainable development concept, including saccharide-based method, irradiation-reduction reduction method and biosynthesis method. Each of these methods has advantages in practical application for the preparation of AgNPs. Overall, the wide source of materials, the simplicity of operation, and the stability of the products are greatly in line with the principles of green chemistry and are instrumental in promoting AgNPs as antibacterial alternative therapeutics. Unlike conventional antibiotics, it combines multiple antibacterial effects which is effective for bacteria that have evolved resistance to antibiotics. Furthermore, AgNPs are equipped with the activity of inhibiting biofilm, which showing beneficial effect to the antibiotic-induced biofilm formation. However, it must take into account that synthesize AgNPs with batch-to-batch reproducibility and scale-up for the following pharmaceutical application. Finaly, the function behavior of AgNP should be reasonably designed to balance the therapeutic outcome and potential toxicity to normal cells and tissues, resulting from the heavy ion effect of metals.
Author contributions
XX, CQ, LX, and QG wrote the manuscript together.
Funding
This work was supported by the startup funding from Jinan University, the Fundamental Research Funds for the Central Universities (No. 11618337), National Natural Science Foundation of China (No. 81903546).
Conflict of interest
Author LX was employed by the company Enantiotech Corp., Ltd.
The remaining authors declare that the research was conducted in the absence of any commercial or financial relationships that could be construed as a potential conflict of interest.
Publisher’s note
All claims expressed in this article are solely those of the authors and do not necessarily represent those of their affiliated organizations, or those of the publisher, the editors and the reviewers. Any product that may be evaluated in this article, or claim that may be made by its manufacturer, is not guaranteed or endorsed by the publisher.
References
Ai, M., Du, Z., Zhu, S., Geng, H., Zhang, X., Cai, Q., et al. (2017). Composite resin reinforced with silver nanoparticles–laden hydroxyapatite nanowires for dental application. Dent. Mat. 33, 12–22. doi:10.1016/j.dental.2016.09.038
Akhavan, A., Sodagar, A., Mojtahedzadeh, F., and Sodagar, K. (2013). Investigating the effect of incorporating nanosilver/nanohydroxyapatite particles on the shear bond strength of orthodontic adhesives. Acta Odontol. Scand. 71, 1038–1042. doi:10.3109/00016357.2012.741699
Alt, V., Bechert, T., Steinrücke, P., Wagener, M., Seidel, P., Dingeldein, E., et al. (2004). An in vitro assessment of the antibacterial properties and cytotoxicity of nanoparticulate silver bone cement. Biomaterials 25, 4383–4391. doi:10.1016/j.biomaterials.2003.10.078
Ameen, F., AlYahya, S., Govarthanan, M., Aljahdali, N., Al-Enazi, N., Alsamhary, K., et al. (2020). Soil bacteria Cupriavidus sp. mediates the extracellular synthesis of antibacterial silver nanoparticles. J. Mol. Struct. 1202, 127233. doi:10.1016/j.molstruc.2019.127233
Ameen, F., Srinivasan, P., Selvankumar, T., Kamala-Kannan, S., Al Nadhari, S., Almansob, A., et al. (2019). Phytosynthesis of silver nanoparticles using Mangifera indica flower extract as bioreductant and their broad-spectrum antibacterial activity. Bioorg. Chem. 88, 102970. doi:10.1016/j.bioorg.2019.102970
Andara, M., Agarwal, A., Scholvin, D., Gerhardt, R. A., Doraiswamy, A., Jin, C., et al. (2006). Hemocompatibility of diamondlike carbon–metal composite thin films. Diam. Relat. Mat. 15, 1941–1948. doi:10.1016/j.diamond.2006.05.013
Antoniadou, A., Kontopidou, F., Poulakou, G., Koratzanis, E., Galani, I., Papadomichelakis, E., et al. (2007). Colistin-resistant isolates of Klebsiella pneumoniae emerging in intensive care unit patients: First report of a multiclonal cluster. J. Antimicrob. Chemother. 59, 786–790. doi:10.1093/jac/dkl562
Arakawa, H., Neault, J. F., and Tajmir-Riahi, H. A. (2001). Silver(I) complexes with DNA and RNA studied by fourier transform infrared spectroscopy and capillary electrophoresis. Biophys. J. 81, 1580–1587. doi:10.1016/S0006-3495(01)75812-2
Aravinthan, A., Govarthanan, M., Selvam, K., Praburaman, L., Selvankumar, T., Balamurugan, R., et al. (2015). Sunroot mediated synthesis and characterization of silver nanoparticles and evaluation of its antibacterial and rat splenocyte cytotoxic effects. Int. J. Nanomedicine 10, 1977–1983. doi:10.2147/IJN.S79106
Arora, S., Jain, J., Rajwade, J. M., and Paknikar, K. M. (2008). Cellular responses induced by silver nanoparticles: In vitro studies. Toxicol. Lett. 179, 93–100. doi:10.1016/j.toxlet.2008.04.009
Atiyeh, B. S., Costagliola, M., Hayek, S. N., and Dibo, S. A. (2007). Effect of silver on burn wound infection control and healing: Review of the literature. Burns 33, 139–148. doi:10.1016/j.burns.2006.06.010
Augustine, R., Hasan, A., Yadu Nath, V. K., Thomas, J., Augustine, A., Kalarikkal, N., et al. (2018). Electrospun polyvinyl alcohol membranes incorporated with green synthesized silver nanoparticles for wound dressing applications. J. Mat. Sci. Mat. Med. 29, 163. doi:10.1007/s10856-018-6169-7
Bao, H. J., Yu, X. X., Xu, C., Li, X., Li, Z. Y., Wei, D. J., et al. (2015). New toxicity mechanism of silver nanoparticles: Promoting apoptosis and inhibiting proliferation. Plos One 10, ARTN e0122535. doi:10.1371/journal.pone.0122535
Bhargava, A., Pareek, V., Roy Choudhury, S., Panwar, J., and Karmakar, S. (2018). Superior bactericidal efficacy of fucose-functionalized silver nanoparticles against Pseudomonas aeruginosa PAO1 and prevention of its colonization on urinary catheters. ACS Appl. Mat. Interfaces 10, 29325–29337. doi:10.1021/acsami.8b09475
Biswas, D. P., O'Brien-Simpson, N. M., Reynolds, E. C., O'Connor, A. J., and Tran, P. A. (2018). Comparative study of novel in situ decorated porous chitosan-selenium scaffolds and porous chitosan-silver scaffolds towards antimicrobial wound dressing application. J. Colloid Interface Sci. 515, 78–91. doi:10.1016/j.jcis.2018.01.007
Blair, J. M. A., Webber, M. A., Baylay, A. J., Ogbolu, D. O., and Piddock, L. J. V. (2015). Molecular mechanisms of antibiotic resistance. Nat. Rev. Microbiol. 13, 42–51. doi:10.1038/nrmicro3380
Chambers, C., Stewart, S. B., Su, B., Jenkinson, H. F., Sandy, J. R., Ireland, A. J., et al. (2017). Silver doped titanium dioxide nanoparticles as antimicrobial additives to dental polymers. Dent. Mat. 33, e115–e123. doi:10.1016/j.dental.2016.11.008
Chen, J., Wang, J., Zhang, X., and Jin, Y. (2008). Microwave-assisted green synthesis of silver nanoparticles by carboxymethyl cellulose sodium and silver nitrate. Mat. Chem. Phys. 108, 421–424. doi:10.1016/j.matchemphys.2007.10.019
Chen, P., Song, L., Liu, Y., and Fang, Y.-e. (2007). Synthesis of silver nanoparticles by γ-ray irradiation in acetic water solution containing chitosan. Radiat. Phys. Chem. Oxf. Engl. 76, 1165–1168. 1993. doi:10.1016/j.radphyschem.2006.11.012
Chien, H.-W., Kuo, C.-J., Kao, L.-H., Lin, G.-Y., and Chen, P.-Y. (2019). Polysaccharidic spent coffee grounds for silver nanoparticle immobilization as a green and highly efficient biocide. Int. J. Biol. Macromol. 140, 168–176. doi:10.1016/j.ijbiomac.2019.08.131
Chinnappan, S., Kandasamy, S., Arumugam, S., Seralathan, K.-K., Thangaswamy, S., Muthusamy, G., et al. (2018). Biomimetic synthesis of silver nanoparticles using flower extract of Bauhinia purpurea and its antibacterial activity against clinical pathogens. Environ. Sci. Pollut. Res. 25, 963–969. doi:10.1007/s11356-017-0841-1
Chladek, G., Kasperski, J., Barszczewska-Rybarek, I., and Żmudzki, J. (2013). Sorption, solubility, bond strength and hardness of denture soft lining incorporated with silver nanoparticles. Int. J. Mol. Sci. 14, 563–574. doi:10.3390/ijms14010563
Choi, O., Deng, K. K., Kim, N.-J., Ross, L., Surampalli, R. Y., Hu, Z., et al. (2008). The inhibitory effects of silver nanoparticles, silver ions, and silver chloride colloids on microbial growth. Water Res. 42, 3066–3074. doi:10.1016/j.watres.2008.02.021
Chowdhury, S., Basu, A., and Kundu, S. (2014). Green synthesis of protein capped silver nanoparticles from phytopathogenic fungus Macrophomina phaseolina (Tassi) Goid with antimicrobial properties against multidrug-resistant bacteria. Nanoscale Res. Lett. 9, 365. doi:10.1186/1556-276X-9-365
Costa Silva, L. P., Oliveira, J. P., Keijok, W. J., da Silva, A. R., Aguiar, A. R., Guimarães, M. C. C., et al. (2017). Extracellular biosynthesis of silver nanoparticles using the cell-free filtrate of nematophagous fungus Duddingtonia flagrans. Int. J. Nanomedicine 12, 6373–6381. doi:10.2147/IJN.S137703
Courtenay, M., Castro-Sanchez, E., Fitzpatrick, M., Gallagher, R., Lim, R., Morris, G., et al. (2019). Tackling antimicrobial resistance 2019–2024 – the UK's five-year national action plan. J. Hosp. Infect. 101, 426–427. doi:10.1016/j.jhin.2019.02.019
Dakshayani, S. S., Marulasiddeshwara, M. B., Sharath Kumar, M. N., Golla, R., Raghavendra, K., Devaraja, S., et al. (2019). Antimicrobial, anticoagulant and antiplatelet activities of green synthesized silver nanoparticles using Selaginella (Sanjeevini) plant extract. Int. J. Biol. Macromol. 131, 787–797. doi:10.1016/j.ijbiomac.2019.01.222
David, M. Z., and Daum, R. S. (2010). Community-associated methicillin-resistant Staphylococcus aureus: Epidemiology and clinical consequences of an emerging epidemic. Clin. Microbiol. Rev. 23, 616–687. doi:10.1128/CMR.00081-09
de Aragão, A. P., de Oliveira, T. M., Quelemes, P. V., Perfeito, M. L. G., Araújo, M. C., Santiago, J. d. A. S., et al. (2019). Green synthesis of silver nanoparticles using the seaweed Gracilaria birdiae and their antibacterial activity. Arab. J. Chem. 12, 4182–4188. doi:10.1016/j.arabjc.2016.04.014
de Mel, A., Chaloupka, K., Malam, Y., Darbyshire, A., Cousins, B., Seifalian, A. M., et al. (2012). A silver nanocomposite biomaterial for blood-contacting implants. J. Biomed. Mat. Res. A 100A, 2348–2357. doi:10.1002/jbm.a.34177
Devadiga, A., Shetty, K. V., and Saidutta, M. B. (2015). Timber industry waste-teak (Tectona grandis Linn.) leaf extract mediated synthesis of antibacterial silver nanoparticles. Int. Nano Lett. 5, 205–214. doi:10.1007/s40089-015-0157-4
DeVasConCellos, P., Bose, S., Beyenal, H., Bandyopadhyay, A., and Zirkle, L. G. (2012). Antimicrobial particulate silver coatings on stainless steel implants for fracture management. Mater. Sci. Eng. C 32, 1112–1120. doi:10.1016/j.msec.2012.02.020
Escárcega-González, C. E., Garza-Cervantes, J. A., Vázquez-Rodríguez, A., Montelongo-Peralta, L. Z., Treviño-González, M. T., Díaz Barriga Castro, E., et al. (2018). In vivo antimicrobial activity of silver nanoparticles produced via a green chemistry synthesis using Acacia rigidula as a reducing and capping agent. Int. J. Nanomedicine 13, 2349–2363. doi:10.2147/IJN.S160605
Feng, Q. L., Wu, J., Chen, G. Q., Cui, F. Z., Kim, T. N., and Kim, J. O. (2000). A mechanistic study of the antibacterial effect of silver ions on Escherichia coli and Staphylococcus aureus. J. Biomed. Mat. Res. 52, 662–668. doi:10.1002/1097-4636(20001215)52:4<662:AID-JBM10>3.0.CO;2-3
Fordham, W. R., Redmond, S., Westerland, A., Cortes, E. G., Walker, C., Gallagher, C., et al. (2014). Silver as a bactericidal coating for biomedical implants. Surf. Coat. Technol. 253, 52–57. doi:10.1016/j.surfcoat.2014.05.013
Gandhi, H., and Khan, S. (2016). Biological synthesis of silver nanoparticles and its antibacterial activity. J. Nanomed. Nanotechnol. 07. doi:10.4172/2157-7439.1000366
Ghanbari, H., Viatge, H., Kidane, A. G., Burriesci, G., Tavakoli, M., Seifalian, A. M., et al. (2009). Polymeric heart valves: New materials, emerging hopes. Trends Biotechnol. 27, 359–367. doi:10.1016/j.tibtech.2009.03.002
GhavamiNejad, A., Park, C. H., and Kim, C. S. (2016). In situ synthesis of antimicrobial silver nanoparticles within antifouling zwitterionic hydrogels by catecholic redox chemistry for wound healing application. Biomacromolecules 17, 1213–1223. doi:10.1021/acs.biomac.6b00039
GhavamiNejad, A., Rajan Unnithan, A., Ramachandra Kurup Sasikala, A., Samarikhalaj, M., Thomas, R. G., Jeong, Y. Y., et al. (2015). Mussel-inspired electrospun nanofibers functionalized with size-controlled silver nanoparticles for wound dressing application. ACS Appl. Mat. Interfaces 7, 12176–12183. doi:10.1021/acsami.5b02542
Ghorbani, P., Soltani, M., Homayouni-Tabrizi, M., Namvar, F., Azizi, S., Mohammad, R., et al. (2015). Sumac silver novel biodegradable nano composite for bio-medical application: Antibacterial activity. Molecules 20, 12946–12958. doi:10.3390/molecules200712946
Govarthanan, M., Selvankumar, T., Manoharan, K., Rathika, R., Shanthi, K., Lee, K.-J., et al. (2014). Biosynthesis and characterization of silver nanoparticles using panchakavya, an Indian traditional farming formulating agent. Int. J. Nanomedicine 9, 1593–1599. doi:10.2147/IJN.S58932
Govarthanan, M., Seo, Y.-S., Lee, K.-J., Jung, I.-B., Ju, H.-J., Kim, J. S., et al. (2016). Low-cost and eco-friendly synthesis of silver nanoparticles using coconut (Cocos nucifera) oil cake extract and its antibacterial activity. Artif. Cells, Nanomedicine, Biotechnol. 44, 1878–1882. doi:10.3109/21691401.2015.1111230
Grunkemeier, G. L., Jin, R., and Starr, A. (2006). Prosthetic heart valves: Objective performance criteria versus randomized clinical trial. Ann. Thorac. Surg. 82, 776–780. doi:10.1016/j.athoracsur.2006.06.037
Guilger-Casagrande, M., Germano-Costa, T., Pasquoto-Stigliani, T., Fraceto, L. F., and Lima, R. d. (2019). Biosynthesis of silver nanoparticles employing Trichoderma harzianum with enzymatic stimulation for the control of Sclerotinia sclerotiorum. Sci. Rep. 9, 14351. doi:10.1038/s41598-019-50871-0
Hamad, M. T. (2019). Biosynthesis of silver nanoparticles by fungi and their antibacterial activity. Int. J. Environ. Sci. Technol. (Tehran). 16, 1015–1024. doi:10.1007/s13762-018-1814-8
Holt, K. B., and Bard, A. J. (2005). Interaction of silver(I) ions with the respiratory chain of Escherichia coli: An electrochemical and scanning electrochemical microscopy study of the antimicrobial mechanism of micromolar Ag+. Biochemistry 44, 13214–13223. doi:10.1021/bi0508542
Hong, K. H., Park, J. L., Sul, I. H., Youk, J. H., and Kang, T. J. (2006). Preparation of antimicrobial poly(vinyl alcohol) nanofibers containing silver nanoparticles. J. Polym. Sci. B. Polym. Phys. 44, 2468–2474. doi:10.1002/polb.20913
Hsu, S.-h., Tseng, H.-J., and Lin, Y.-C. (2010). The biocompatibility and antibacterial properties of waterborne polyurethane-silver nanocomposites. Biomaterials 31, 6796–6808. doi:10.1016/j.biomaterials.2010.05.015
Hu, B., Wang, S.-B., Wang, K., Zhang, M., and Yu, S.-H. (2008). Microwave-Assisted rapid facile “green” synthesis of uniform silver nanoparticles: Self-assembly into multilayered films and their optical properties. J. Phys. Chem. C 112, 11169–11174. doi:10.1021/jp801267j
Hu, X., Saravanakumar, K., Jin, T., and Wang, M.-H. (2019). Mycosynthesis, characterization, anticancer and antibacterial activity of silver nanoparticles from endophytic fungus <em>Talaromyces purpureogenus</em>. Int. J. Nanomedicine 14, 3427–3438. doi:10.2147/IJN.S200817
Huang, H., and Yang, X. (2004). Synthesis of polysaccharide-stabilized gold and silver nanoparticles: A green method. Carbohydr. Res. 339, 2627–2631. doi:10.1016/j.carres.2004.08.005
Huang, Y., Li, X., Liao, Z., Zhang, G., Liu, Q., Tang, J., et al. (2007). A randomized comparative trial between Acticoat and SD-Ag in the treatment of residual burn wounds, including safety analysis. Burns 33, 161–166. doi:10.1016/j.burns.2006.06.020
Huang, Y., Xu, Z., Zhang, X., Chang, X., Zhang, X., Li, Y., et al. (2017). Nanotube-formed Ti substrates coated with silicate/silver co-doped hydroxyapatite as prospective materials for bone implants. J. Alloys Compd. 697, 182–199. doi:10.1016/j.jallcom.2016.12.139
Hussain, S. M., Hess, K. L., Gearhart, J. M., Geiss, K. T., and Schlager, J. J. (2005). In vitro toxicity of nanoparticles in BRL 3A rat liver cells. Toxicol. Vitro 19, 975–983. doi:10.1016/j.tiv.2005.06.034
Ji, J. H., Jung, J. H., Kim, S. S., Yoon, J.-U., Park, J. D., Choi, B. S., et al. (2007). Twenty-eight-day inhalation toxicity study of silver nanoparticles in sprague-dawley rats. Inhal. Toxicol. 19, 857–871. doi:10.1080/08958370701432108
Kalishwaralal, K., BarathManiKanth, S., Pandian, S. R. K., Deepak, V., and Gurunathan, S. (2010). Silver nanoparticles impede the biofilm formation by Pseudomonas aeruginosa and Staphylococcus epidermidis. Colloids Surfaces B Biointerfaces 79, 340–344. doi:10.1016/j.colsurfb.2010.04.014
Karim, M. R., Lim, K. T., Lee, C. J., Bhuiyan, M. T. I., Kim, H. J., Park, L.-S., et al. (2007). Synthesis of core-shell silver–polyaniline nanocomposites by gamma radiolysis method. J. Polym. Sci. A. Polym. Chem. 45, 5741–5747. doi:10.1002/pola.22323
Kathiraven, T., Sundaramanickam, A., Shanmugam, N., and Balasubramanian, T. (2015). Green synthesis of silver nanoparticles using marine algae Caulerpa racemosa and their antibacterial activity against some human pathogens. Appl. Nanosci. 5, 499–504. doi:10.1007/s13204-014-0341-2
Kawata, K., Osawa, M., and Okabe, S. (2009). In vitro toxicity of silver nanoparticles at noncytotoxic doses to HepG2 human hepatoma cells. Environ. Sci. Technol. 43, 6046–6051. doi:10.1021/es900754q
Kim, W.-Y., Kim, J., Park, J. D., Ryu, H. Y., and Yu, I. J. (2009). Histological study of gender differences in accumulation of silver nanoparticles in kidneys of fischer 344 rats. J. Toxicol. Environ. Health A 72, 1279–1284. doi:10.1080/15287390903212287
Kim, Y. S., Kim, J. S., Cho, H. S., Rha, D. S., Kim, J. M., Park, J. D., et al. (2008). Twenty-eight-day oral toxicity, genotoxicity, and gender-related tissue distribution of silver nanoparticles in sprague-dawley rats. Inhal. Toxicol. 20, 575–583. doi:10.1080/08958370701874663
Kumarasamy, K. K., Toleman, M. A., Walsh, T. R., Bagaria, J., Butt, F., Balakrishnan, R., et al. (2010). Emergence of a new antibiotic resistance mechanism in India, Pakistan, and the UK: A molecular, biological, and epidemiological study. Lancet Infect. Dis. 10, 597–602. doi:10.1016/S1473-3099(10)70143-2
Lackner, P., Beer, R., Broessner, G., Helbok, R., Galiano, K., Pleifer, C., et al. (2008). Efficacy of silver nanoparticles-impregnated external ventricular drain catheters in patients with acute occlusive hydrocephalus. Neurocrit. Care 8, 360–365. doi:10.1007/s12028-008-9071-1
Lam, P. K., Chan, E. S. Y., Ho, W. S., and Liew, C. T. (2004). In vitro cytotoxicity testing of a nanocrystalline silver dressing (Acticoat) on cultured keratinocytes. Br. J. Biomed. Sci. 61, 125–127. doi:10.1080/09674845.2004.11732656
Lateef, A., Adelere, I. A., Gueguim-Kana, E. B., Asafa, T. B., and Beukes, L. S. (2015). Green synthesis of silver nanoparticles using keratinase obtained from a strain of Bacillus safensis LAU 13. Int. Nano Lett. 5, 29–35. doi:10.1007/s40089-014-0133-4
Lee, C. J., Karim, M. R., and Lee, M. S. (2007). Synthesis and characterization of silver/thiophene nanocomposites by UV-irradiation method. Mat. Lett. 61, 2675–2678. doi:10.1016/j.matlet.2006.10.021
Lee, H.-Y., Choi, Y.-J., Jung, E.-J., Yin, H.-Q., Kwon, J.-T., Kim, J.-E., et al. (2010). Genomics-based screening of differentially expressed genes in the brains of mice exposed to silver nanoparticles via inhalation. J. Nanopart. Res. 12, 1567–1578. doi:10.1007/s11051-009-9666-2
Lee, K.-J., Park, S.-H., Govarthanan, M., Hwang, P.-H., Seo, Y.-S., Cho, M., et al. (2013). Synthesis of silver nanoparticles using cow milk and their antifungal activity against phytopathogens. Mat. Lett. 105, 128–131. doi:10.1016/j.matlet.2013.04.076
Lee, S. H., Salunke, B. K., and Kim, B. S. (2014a). Sucrose density gradient centrifugation separation of gold and silver nanoparticles synthesized using Magnolia kobus plant leaf extracts. Biotechnol. Bioprocess Eng. 19, 169–174. doi:10.1007/s12257-013-0561-4
Lee, W., Kim, K.-J., and Lee, D. G. (2014b). A novel mechanism for the antibacterial effect of silver nanoparticles on Escherichia coli. BioMetals 27, 1191–1201. doi:10.1007/s10534-014-9782-z
Liau, S. Y., Read, D. C., Pugh, W. J., Furr, J. R., and Russell, A. D. (1997). Interaction of silver nitrate with readily identifiable groups: Relationship to the antibacterialaction of silver ions. Lett. Appl. Microbiol. 25, 279–283. doi:10.1046/j.1472-765X.1997.00219.x
Liu, F.-K., Hsu, Y.-C., Tsai, M.-H., and Chu, T.-C. (2007). Using γ-irradiation to synthesize Ag nanoparticles. Mat. Lett. 61, 2402–2405. doi:10.1016/j.matlet.2006.07.193
Liu, Y., Zheng, Z., Zara, J. N., Hsu, C., Soofer, D. E., Lee, K. S., et al. (2012). The antimicrobial and osteoinductive properties of silver nanoparticle/poly (dl-lactic-co-glycolic acid)-coated stainless steel. Biomaterials 33, 8745–8756. doi:10.1016/j.biomaterials.2012.08.010
Long, D., Wu, G., and Chen, S. (2007). Preparation of oligochitosan stabilized silver nanoparticles by gamma irradiation. Radiat. Phys. Chem. Oxf. Engl. 76, 1126–1131. 1993. doi:10.1016/j.radphyschem.2006.11.001
Lu, S., Gao, W., and Gu, H. Y. (2008). Construction, application and biosafety of silver nanocrystalline chitosan wound dressing. Burns 34, 623–628. doi:10.1016/j.burns.2007.08.020
Luo, C., Zhang, Y., Zeng, X., Zeng, Y., and Wang, Y. (2005). The role of poly(ethylene glycol) in the formation of silver nanoparticles. J. Colloid Interface Sci. 288, 444–448. doi:10.1016/j.jcis.2005.03.005
Madhumathi, K., Sudheesh Kumar, P. T., Abhilash, S., Sreeja, V., Tamura, H., Manzoor, K., et al. (2010). Development of novel chitin/nanosilver composite scaffolds for wound dressing applications. J. Mat. Sci. Mat. Med. 21, 807–813. doi:10.1007/s10856-009-3877-z
Magalhães, A. P. R., Santos, L. B., Lopes, L. G., Estrela, C. R. d. A., Estrela, C., Torres, É. M., et al. (2012). Nanosilver application in dental cements. ISRN Nanotechnol. 2012, 1–6. doi:10.5402/2012/365438
Massironi, A., Morelli, A., Grassi, L., Puppi, D., Braccini, S., Maisetta, G., et al. (2019). Ulvan as novel reducing and stabilizing agent from renewable algal biomass: Application to green synthesis of silver nanoparticles. Carbohydr. Polym. 203, 310–321. doi:10.1016/j.carbpol.2018.09.066
McDonnell, G., and Russell, A. D. (1999). Antiseptics and disinfectants: Activity, action, and resistance. Clin. Microbiol. Rev. 12, 147–179. doi:10.1128/CMR.12.1.147
McManus, M. C. (1997). Mechanisms of bacterial resistance to antimicrobial agents. Am. J. Health. Syst. Pharm. 54, 1420–1433. quiz 1444-6. doi:10.1093/ajhp/54.12.1420
Mohammad, N. H., El-Sherbiny, G. M., Hammad, A. A., Askar, A. A., and El- Nour, S. A. A. (2022). Gamma-ray and sunlight-induced synthesis of silver nanoparticles using bacterial cellulose and cell-free filtrate produced by Komagataeibacter rhaeticus N1 MW322708 strain. Cellulose 29, 1791–1805. doi:10.1007/s10570-021-04356-x
Morones, J. R., Elechiguerra, J. L., Camacho, A., Holt, K., Kouri, J. B., Ramírez, J. T., et al. (2005). The bactericidal effect of silver nanoparticles. Nanotechnology 16, 2346–2353. doi:10.1088/0957-4484/16/10/059
Munita, J. M., Bayer, A. S., and Arias, C. A. (2015). Evolving resistance among Gram-positive pathogens. Clin. Infect. Dis. 61 (Suppl. 2), S48–S57. doi:10.1093/cid/civ523
Muthusamy, G., Thangasamy, S., Raja, M., Chinnappan, S., and Kandasamy, S. (2017). Biosynthesis of silver nanoparticles from Spirulina microalgae and its antibacterial activity. Environ. Sci. Pollut. Res. 24, 19459–19464. doi:10.1007/s11356-017-9772-0
Mythili, R., Selvankumar, T., Kamala-Kannan, S., Sudhakar, C., Ameen, F., Al-Sabri, A., et al. (2018). Utilization of market vegetable waste for silver nanoparticle synthesis and its antibacterial activity. Mat. Lett. 225, 101–104. doi:10.1016/j.matlet.2018.04.111
Nguyen, V. Q., Ishihara, M., Mori, Y., Nakamura, S., Kishimoto, S., Hattori, H., et al. (2013). Preparation of size-controlled silver nanoparticles and chitin-based composites and their antimicrobial activities. J. Nanomater. 2013, 1–7. doi:10.1155/2013/693486
Oliveira, W. F., Silva, P. M. S., Silva, R. C. S., Silva, G. M. M., Machado, G., Coelho, L. C. B. B., et al. (2018). Staphylococcus aureus and Staphylococcus epidermidis infections on implants. J. Hosp. Infect. 98, 111–117. doi:10.1016/j.jhin.2017.11.008
Paddle-Ledinek, J. E., Nasa, Z., and Cleland, H. J. (2006). Effect of different wound dressings on cell viability and proliferation. Plastic Reconstr. Surg. 117, 110S–118S. discussion 119S-120S. doi:10.1097/01.prs.0000225439.39352.ce
Pal, S., Tak, Y. K., and Song, J. M. (2007). Does the antibacterial activity of silver nanoparticles depend on the shape of the nanoparticle? A study of the gram-negative bacterium Escherichia coli. Appl. Environ. Microbiol. 73, 1712–1720. doi:10.1128/AEM.02218-06
Paladini, F., Pollini, M., Deponti, D., Di Giancamillo, A., Peretti, G., Sannino, A., et al. (2013). Effect of silver nanocoatings on catheters for haemodialysis in terms of cell viability, proliferation, morphology and antibacterial activity. J. Mat. Sci. Mat. Med. 24, 1105–1112. doi:10.1007/s10856-013-4870-0
Park, H.-J., Kim, J. Y., Kim, J., Lee, J.-H., Hahn, J.-S., Gu, M. B., et al. (2009). Silver-ion-mediated reactive oxygen species generation affecting bactericidal activity. Water Res. 43, 1027–1032. doi:10.1016/j.watres.2008.12.002
Park, S., Lee, Y. K., Jung, M., Kim, K. H., Chung, N., Ahn, E.-K., et al. (2007). Cellular toxicity of various inhalable metal nanoparticles on human alveolar epithelial cells. Inhal. Toxicol. 19, 59–65. doi:10.1080/08958370701493282
Poon, V. K. M., and Burd, A. (2004). In vitro cytotoxity of silver: Implication for clinical wound care. Burns 30, 140–147. doi:10.1016/j.burns.2003.09.030
Raveendran, P., Fu, J., and Wallen, S. (2006). A simple and “green” method for the synthesis of Au, Ag, and Au–Ag alloy nanoparticles. Green Chem. 8, 34–38. doi:10.1039/b512540e
Raveendran, P., Fu, J., and Wallen, S. L. (2003). Completely “green” synthesis and stabilization of metal nanoparticles. J. Am. Chem. Soc. 125, 13940–13941. doi:10.1021/ja029267j
Roe, D., Karandikar, B., Bonn-Savage, N., Gibbins, B., and Roullet, J.-B. (2008). Antimicrobial surface functionalization of plastic catheters by silver nanoparticles. J. Antimicrob. Chemother. 61, 869–876. doi:10.1093/jac/dkn034
Russell, A. D., and Hugo, W. B. (1994a). 7 antimicrobial activity and action of silver. In "Progress in medicinal chemistry" (G. P. Ellis, and D. K. Luscombe, eds.), Vol. 31, pp. 351–370. Elsevier.
Russell, A. D., and Hugo, W. B. (1994b). Antimicrobial activity and action of silver. Prog. Med. Chem. 31, 351–370. doi:10.1016/s0079-6468(08)70024-9
Sabbagh, P., Riahi, S. M., Gamble, H. R., and Rostami, A. (2019). The global and regional prevalence, burden, and risk factors for methicillin-resistant Staphylococcus aureus colonization in HIV-infected people: A systematic review and meta-analysis. Am. J. Infect. Control 47, 323–333. doi:10.1016/j.ajic.2018.06.023
Sacco, P., Travan, A., Borgogna, M., Paoletti, S., and Marsich, E. (2015). Silver-containing antimicrobial membrane based on chitosan-TPP hydrogel for the treatment of wounds. J. Mat. Sci. Mat. Med. 26, 128. doi:10.1007/s10856-015-5474-7
Salaie, R. N., Besinis, A., Le, H., Tredwin, C., and Handy, R. D. (2020). The biocompatibility of silver and nanohydroxyapatite coatings on titanium dental implants with human primary osteoblast cells. Mater. Sci. Eng. C 107, 110210. doi:10.1016/j.msec.2019.110210
Samberg, M. E., Oldenburg, S. J., and Monteiro-Riviere, N. A. (2010). Evaluation of silver nanoparticle toxicity in skin in vivo and keratinocytes in vitro. Environ. Health Perspect. 118, 407–413. doi:10.1289/ehp.0901398
Saravanan, M., Barik, S. K., MubarakAli, D., Prakash, P., and Pugazhendhi, A. (2018). Synthesis of silver nanoparticles from Bacillus brevis (NCIM 2533) and their antibacterial activity against pathogenic bacteria. Microb. Pathog. 116, 221–226. doi:10.1016/j.micpath.2018.01.038
Seku, K., Gangapuram, B. R., Pejjai, B., Kadimpati, K. K., and Golla, N. (2018). Microwave-assisted synthesis of silver nanoparticles and their application in catalytic, antibacterial and antioxidant activities. J. Nanostructure Chem. 8, 179–188. doi:10.1007/s40097-018-0264-7
Selvam, K., Sudhakar, C., Govarthanan, M., Thiyagarajan, P., Sengottaiyan, A., Senthilkumar, B., et al. (2017). Eco-friendly biosynthesis and characterization of silver nanoparticles using Tinospora cordifolia (Thunb.) Miers and evaluate its antibacterial, antioxidant potential. J. Radiat. Res. Appl. Sci. 10, 6–12. doi:10.1016/j.jrras.2016.02.005
Sengottaiyan, A., Aravinthan, A., Sudhakar, C., Selvam, K., Srinivasan, P., Govarthanan, M., et al. (2016a). Synthesis and characterization of Solanum nigrum-mediated silver nanoparticles and its protective effect on alloxan-induced diabetic rats. J. Nanostructure Chem. 6, 41–48. doi:10.1007/s40097-015-0178-6
Sengottaiyan, A., Mythili, R., Selvankumar, T., Aravinthan, A., Kamala-Kannan, S., Manoharan, K., et al. (2016b). Green synthesis of silver nanoparticles using Solanum indicum L. and their antibacterial, splenocyte cytotoxic potentials. Res. Chem. Intermed. 42, 3095–3103. doi:10.1007/s11164-015-2199-7
Shameli, K., Ahmad, M. B., Jazayeri, S. D., Shabanzadeh, P., Sangpour, P., Jahangirian, H., et al. (2012). Investigation of antibacterial properties silver nanoparticles prepared via green method. Chem. Cent. J. 6, 73. doi:10.1186/1752-153X-6-73
Shin, H. S., Yang, H. J., Kim, S. B., and Lee, M. S. (2004). Mechanism of growth of colloidal silver nanoparticles stabilized by polyvinyl pyrrolidone in γ-irradiated silver nitrate solution. J. Colloid Interface Sci. 274, 89–94. doi:10.1016/j.jcis.2004.02.084
Silver, S., Phung, L. T., and Silver, G. (2006). Silver as biocides in burn and wound dressings and bacterial resistance to silver compounds. J. Ind. Microbiol. Biotechnol. 33, 627–634. doi:10.1007/s10295-006-0139-7
Singh, P., Kim, Y. J., Wang, C., Mathiyalagan, R., and Yang, D. C. (2016). Weissella oryzae DC6-facilitated green synthesis of silver nanoparticles and their antimicrobial potential. Artif. Cells Nanomed. Biotechnol. 44, 1569–1575. doi:10.3109/21691401.2015.1064937
Singh, R., and Singh, D. (2014). Chitin membranes containing silver nanoparticles for wound dressing application. Int. Wound J. 11, 264–268. doi:10.1111/j.1742-481X.2012.01084.x
Singh, T., Jyoti, K., Patnaik, A., Singh, A., Chauhan, R., Chandel, S. S., et al. (2017). Biosynthesis, characterization and antibacterial activity of silver nanoparticles using an endophytic fungal supernatant of Raphanus sativus. J. Genet. Eng. Biotechnol. 15, 31–39. doi:10.1016/j.jgeb.2017.04.005
Singhal, A., and Gupta, A. (2019). Sustainable synthesis of silver nanoparticles using exposed X-ray sheets and forest-industrial waste biomass: Assessment of kinetic and catalytic properties for degradation of toxic dyes mixture. J. Environ. Manage. 247, 698–711. doi:10.1016/j.jenvman.2019.06.078
Sinha, S. N., Paul, D., Halder, N., Sengupta, D., and Patra, S. K. (2015). Green synthesis of silver nanoparticles using fresh water green alga Pithophora oedogonia (Mont.) Wittrock and evaluation of their antibacterial activity. Appl. Nanosci. 5, 703–709. doi:10.1007/s13204-014-0366-6
Solomon, S. L., and Oliver, K. B. (2014). Antibiotic resistance threats in the United States: Stepping back from the brink. Am. Fam. Physician 89, 938–941.
Sondi, I., and Salopek-Sondi, B. (2004). Silver nanoparticles as antimicrobial agent: A case study on E. coli as a model for gram-negative bacteria. J. Colloid Interface Sci. 275, 177–182. doi:10.1016/j.jcis.2004.02.012
Soto, K. M., Quezada-Cervantes, C. T., Hernández-Iturriaga, M., Luna-Bárcenas, G., Vazquez-Duhalt, R., Mendoza, S., et al. (2019). Fruit peels waste for the green synthesis of silver nanoparticles with antimicrobial activity against foodborne pathogens. LWT 103, 293–300. doi:10.1016/j.lwt.2019.01.023
Stamplecoskie, K. G., and Scaiano, J. C. (2010). Light emitting diode irradiation can control the morphology and optical properties of silver nanoparticles. J. Am. Chem. Soc. 132, 1825–1827. doi:10.1021/ja910010b
Sudhakar, C., Selvam, K., Govarthanan, M., Senthilkumar, B., Sengottaiyan, A., Stalin, M., et al. (2015). Acorus calamus rhizome extract mediated biosynthesis of silver nanoparticles and their bactericidal activity against human pathogens. J. Genet. Eng. Biotechnol. 13, 93–99. doi:10.1016/j.jgeb.2015.10.003
Tai, C. Y., Wang, Y.-H., and Liu, H.-S. (2008). A green process for preparing silver nanoparticles using spinning disk reactor. AIChE J. 54, 445–452. doi:10.1002/aic.11396
Tang, J., Xiong, L., Wang, S., Wang, J., Liu, L., Li, J., et al. (2008). Influence of silver nanoparticles on neurons and blood-brain barrier via subcutaneous injection in rats. Appl. Surf. Sci. 255, 502–504. doi:10.1016/j.apsusc.2008.06.058
Thokala, N., Kealey, D. C., Kennedy, D. J., Brady, D. D. B., and Farrell, D. J. (2018). Comparative activity of silver-based antimicrobial composites for urinary catheters. Int. J. Antimicrob. Agents 52, 166–171. doi:10.1016/j.ijantimicag.2018.03.015
Travan, A., Marsich, E., Donati, I., Benincasa, M., Giazzon, M., Felisari, L., et al. (2011). Silver–polysaccharide nanocomposite antimicrobial coatings for methacrylic thermosets. Acta Biomater. 7, 337–346. doi:10.1016/j.actbio.2010.07.024
Unnithan, A. R., Ghavami Nejad, A., Sasikala, A. R. K., Thomas, R. G., Jeong, Y. Y., Murugesan, P., et al. (2016). Electrospun zwitterionic nanofibers with in situ decelerated epithelialization property for non-adherent and easy removable wound dressing application. Chem. Eng. J. 287, 640–648. doi:10.1016/j.cej.2015.11.086
Valarmathi, N., Ameen, F., Almansob, A., Kumar, P., Arunprakash, S., Govarthanan, M., et al. (2020). Utilization of marine seaweed Spyridia filamentosa for silver nanoparticles synthesis and its clinical applications. Mat. Lett. 263, 127244. doi:10.1016/j.matlet.2019.127244
Valodkar, M., Bhadoria, A., Pohnerkar, J., Mohan, M., and Thakore, S. (2010). Morphology and antibacterial activity of carbohydrate-stabilized silver nanoparticles. Carbohydr. Res. 345, 1767–1773. doi:10.1016/j.carres.2010.05.005
van de Belt, H., Neut, D., Schenk, W., van Horn, J. R., van der Mei, H. C., Busscher, H. J., et al. (2001). Infection of orthopedic implants and the use of antibiotic-loaded bone cements - a review. Acta Orthop. Scand. 72, 557–571. doi:10.1080/000164701317268978
van Hengel, I. A. J., Tierolf, M. W. A. M., Valerio, V. P. M., Minneboo, M., Fluit, A. C., Fratila-Apachitei, L. E., et al. (2020). Self-defending additively manufactured bone implants bearing silver and copper nanoparticles. J. Mat. Chem. B 8, 1589–1602. doi:10.1039/C9TB02434D
Vigneshwaran, N., Nachane, R. P., Balasubramanya, R. H., and Varadarajan, P. V. (2006). A novel one-pot 'green' synthesis of stable silver nanoparticles using soluble starch. Carbohydr. Res. 341, 2012–2018. doi:10.1016/j.carres.2006.04.042
Wang, C., Kim, Y. J., Singh, P., Mathiyalagan, R., Jin, Y., Yang, D. C., et al. (2016). Green synthesis of silver nanoparticles by Bacillus methylotrophicus, and their antimicrobial activity. Artif. Cells Nanomed. Biotechnol. 44, 1127–1132. doi:10.3109/21691401.2015.1011805
Wang, L., Wu, Y., Xie, J., Wu, S., and Wu, Z. (2018). Characterization, antioxidant and antimicrobial activities of green synthesized silver nanoparticles from Psidium guajava L. leaf aqueous extracts. Mater. Sci. Eng. C 86, 1–8. doi:10.1016/j.msec.2018.01.003
Wang, M., Zhang, W., Zheng, X., and Zhu, P. (2017). Antibacterial and catalytic activities of biosynthesized silver nanoparticles prepared by using an aqueous extract of green coffee bean as a reducing agent. RSC Adv. 7, 12144–12149. doi:10.1039/C6RA27706C
Williams, B., Gautham, I., Grady, T. L., and Fernando, H. (2022). Redox properties and temperature dependence of silver nanoparticles synthesized using pasteurized cow and goat milk. Green Chem. Lett. Rev. 15, 71–82. doi:10.1080/17518253.2021.2018507
Wilson, D. N. (2014). Ribosome-targeting antibiotics and mechanisms of bacterial resistance. Nat. Rev. Microbiol. 12, 35–48. doi:10.1038/nrmicro3155
World Health Organization, (2020). Antimicrobial resistance. Available at: https://www.who.int/news-room/fact-sheets/detail/antimicrobial-resistance (Accessed June 23, 2020).
World Health, O. (2012). The evolving threat of antimicrobial resistance : Options for action. Geneva: World Health Organization.
Wu, C.-N., Fuh, S.-C., Lin, S.-P., Lin, Y.-Y., Chen, H.-Y., Liu, J.-M., et al. (2018). TEMPO-oxidized bacterial cellulose pellicle with silver nanoparticles for wound dressing. Biomacromolecules 19, 544–554. doi:10.1021/acs.biomac.7b01660
Xue, Y., Qiu, X., Liu, Z., and Li, Y. (2018). Facile and efficient synthesis of silver nanoparticles based on biorefinery wood lignin and its application as the optical sensor. ACS Sustain. Chem. Eng. 6, 7695–7703. doi:10.1021/acssuschemeng.8b00578
Zhang, L.-S., Wong, K.-H., Yip, H.-Y., Hu, C., Yu, J. C., Chan, C.-Y., et al. (2010). Effective photocatalytic disinfection of E. coli K-12 using AgBr−Ag−Bi2WO6 nanojunction system irradiated by visible light: The role of diffusing hydroxyl radicals. Environ. Sci. Technol. 44, 1392–1398. doi:10.1021/es903087w
Zhang, L., Yu, J. C., Yip, H. Y., Li, Q., Kwong, K. W., Xu, A.-W., et al. (2003). Ambient light reduction strategy to synthesize silver nanoparticles and silver-coated TiO2 with enhanced photocatalytic and bactericidal activities. Langmuir 19, 10372–10380. doi:10.1021/la035330m
Zhang, S., Wang, L., Liang, X., Vorstius, J., Keatch, R., Corner, G., et al. (2019). Enhanced antibacterial and antiadhesive activities of silver-PTFE nanocomposite coating for urinary catheters. ACS Biomater. Sci. Eng. 5, 2804–2814. doi:10.1021/acsbiomaterials.9b00071
Zhao, L., Wang, H., Huo, K., Cui, L., Zhang, W., Ni, H., et al. (2011). Antibacterial nano-structured titania coating incorporated with silver nanoparticles. Biomaterials 32, 5706–5716. doi:10.1016/j.biomaterials.2011.04.040
Zheng, Z., Yin, W., Zara, J. N., Li, W., Kwak, J., Mamidi, R., et al. (2010). The use of BMP-2 coupled – nanosilver-PLGA composite grafts to induce bone repair in grossly infected segmental defects. Biomaterials 31, 9293–9300. doi:10.1016/j.biomaterials.2010.08.041
Zhou, Y., Yu, S. H., Wang, C. Y., Li, X. G., Zhu, Y. R., Chen, Z. Y., et al. (1999). A novel ultraviolet irradiation photoreduction technique for the preparation of single-crystal Ag nanorods and Ag dendrites. Adv. Mat. 11, 850–852. doi:10.1002/(SICI)1521-4095(199907)11:10<850:AID-ADMA850>3.0.CO;2-Z
Keywords: antibacterial resistance, silver nanoparticles, green synthesis, antibacterial activity, sustainability
Citation: Xin X, Qi C, Xu L, Gao Q and Liu X (2022) Green synthesis of silver nanoparticles and their antibacterial effects. Front. Chem. Eng. 4:941240. doi: 10.3389/fceng.2022.941240
Received: 11 May 2022; Accepted: 05 July 2022;
Published: 08 August 2022.
Edited by:
Filipa A. Vicente, National Institute of Chemistry, SloveniaReviewed by:
Ashish Bohre, National Institute of Chemistry, SloveniaGamal Mohamed El-Said El-Sherbiny, Al-Azhar University, Egypt
Ricardo João Borges Pinto, University of Aveiro, Portugal
Copyright © 2022 Xin, Qi, Xu, Gao and Liu. This is an open-access article distributed under the terms of the Creative Commons Attribution License (CC BY). The use, distribution or reproduction in other forums is permitted, provided the original author(s) and the copyright owner(s) are credited and that the original publication in this journal is cited, in accordance with accepted academic practice. No use, distribution or reproduction is permitted which does not comply with these terms.
*Correspondence: Xiaowen Liu, xwliu231@jnu.edu.cn
†These authors have contributed equally to this work