- Chemical Sciences and Engineering Division, Argonne National Laboratory, Lemont, IL, United States
The properties of many solid-state materials arise from critical interfaces tied to the structure, morphology, and composition of the materials under study. For many materials, identifying components that may be invisible to diffraction techniques or other bulk sensitive techniques (i.e. inductively coupled plasma (ICP)), may cause important information to be overlooked. These can include grain boundary phases, nanoscale coatings, amorphous layers, or second phases that influence the materials environment. In this short review, the use of 29Si MAS NMR as a local probe to detect silicon-containing phases in complex energy storage systems is explored with a focus is on silicon-containing materials and silicon electrodes. Examples highlighting the utility of 29Si MAS NMR include 1) examining copper diffusion into silicon as a method to create 3 dimensional electrodes, 2) using Mg(II) electrolyte additives to create in-situ nanoscale silicide coatings to inhibit low voltage parasitic side reactions and extend calendar life, and 3) studying the lithiation reactions of passivated silicon on different time scales.
Introduction
Understanding solid-state diffusion is critical to understanding the role of syntheses in areas ranging from materials processing to the creation of protective coatings (van der Ven et al., 2013). While optimizing solid state diffusion is the desired result of many materials studies, i.e. Li+ conduction in Li7La3Zr2O12 or O2- diffusion in (Y,Zr)O2-x, understanding its role in the synthetic pathway can help form specific structures or change the rate of reaction (Descemond et al., 1993; Kuhn et al., 2011). As an example, the commercial lithium-ion battery (LIB) cathode LiCoO2 exists in two forms depending on the synthesis route chosen (Gummow et al., 1993a). Using a conventional solid-state synthesis heated to high temperature (∼900°C), a hexagonal layered phase is isolated. However, it was noted by Gummow, et al., that if the mixture is heated to only 400°C for an extended period and cooled to room temperature, a three-dimensional cubic spinel structure with a distinct electrochemical cycling profile is seen rather than the high temperature hexagonal layered oxide (Gummow et al., 1993a; Gummow et al., 1993b). Later studies by Kan et al., showed that samples annealed for shorter time lengths, intermediate intergrowths in the samples were observed (Kan et al., 2014; Shi et al., 2021). Mechanistic studies highlighted that the spinel-phase is the dominant product below 620°C followed by conversion to the layered LiCoO2. In these examples, the spinel cation ordering was identified by diffraction methods, in-situ spectroscopic techniques, thermal properties, and electrochemical properties to correlate the structural differences to the electrochemical properties. In this report, the use of local-probe 29Si Solid State Magic Angle Spinning (MAS) NMR spectroscopy is reviewed for its uses as a tool to better understand the role of annealing temperature, precursor, and sample history on the properties of energy storage materials (Blanc et al., 2013; Delpuech et al., 2016; Dogan and Vaughey, 2016; Michan et al., 2016). Specific examples from the literature in the fields of solid-state electrolytes, electrode formation, and silicon-based anode materials will be used to create a better understanding of the synthetic processes involved.
29Si NMR Studies of Silicon-Based LIB Electrodes
29Si Solid State Magic Angle Spinning (MAS) NMR spectroscopy is a local structural probe that can identify the different local silicon environments in a sample and correlate them with compositions. In a study of the copper/silicon deposition process used to create an all-inorganic electrode, the local environments and phases formed after processing the copper-coated silicon powder at various temperatures were identified by 29Si MAS NMR (Dogan et al., 2013). This study, part of an effort to understand the interface between nanoscale copper and silicon, was performed in the temperature range (450–650°C) to study intermetallic formation and copper diffusion in silicon. Previous diffraction studies showed formation of the electrochemically inactive phase Cu3Si for samples heated above 700°C but the isolation of disordered (lacking long-range order) or amorphous species (i.e., nanoscale silicon oxides, surface silicon hydrides/hydroxides) was not possible using XRD methods (Joyce et al., 2012). Identifying these interfacial phases is important in understanding the electrode properties as many silicon oxides are insulating and electrochemically inactive. Globally, their presence on the particle surface has an effect on the cell impedance, irreversible capacity, and SEI stability.
Since 29Si MAS NMR spectroscopy is sensitive to the local environment of silicon, it can be used to study the formation of both ordered (crystalline) and disordered materials within the electrode at various processing temperatures. To focus the study on surface species, 29Si single pulse (SP) and 29Si{1H} cross-polarization (CP) techniques were combined so that both bulk and protonated surface silicon sites can be identified. Using 29Si{1H} CP MAS NMR allowed the selective investigation and detection of the 29Si NMR resonances from silicon atoms in close proximity to protons and surface silicon atoms.
Background
The silicon isotope 29Si has a spin I = ½ and a low natural abundance of 4.7%. Despite its low natural abundance, it is commonly used as structural characterization tool for silicon oxides and zeolite-based catalysts and more recently silicon based energy storage materials. The locations of the 29Si NMR peak shift values, reported relative to a tetramethylsilane (TMS) standard, are directly related to the shielding of the 29Si nucleus by the electronic structure in its immediate environment. Therefore, 29Si MAS NMR spectroscopy provides direct information about the structure of silicon compounds via measurements of the isotropic chemical shifts (Blanc et al., 2013; Delpuech et al., 2016; Dogan and Vaughey, 2016; Michan et al., 2016). The observed silicon chemical shift is influenced by: 1) the coordination number of the Si, 2) the nature of the nuclei in the first coordination sphere, and 3) the local symmetry of the silicon’s environment. Based on literature studies, the 29Si chemical shift of bulk silicon is a sharp resonance at −80 ppm. While the linewidth increases with increasing local environment disorder, by utilizing the Magic Angle Spinning (MAS) technique, the averaging of dipole dipole interactions and chemical shift anisotropy, reduces the peak width (Chang et al., 1996). Since the silicon linewidth in powder samples is dominated by these interactions, using MAS to examine these different silicon local environments can greatly improve the data resolution. Literature studies report a typical chemical shift value of −40 ppm for amorphous silicon which changes depending on the hydrogen content up to an experimental value of −92.5 ppm for silane (SiH4) (Pietraβ et al., 1996). Silicon oxide environments typically give rise to 29Si peaks in the range −90 to −110 ppm, depending on the local environment and coordination and are often referred to with a Qn to indicate the number of bridging bonds (-O-Si) tied to the central silicon atoms (Mackenzie and Smith, 2002; Petit et al., 1063).
For the annealed inorganic electrodes, samples were prepared using electroless Cu deposition. The literature method operates by the reduction of soluble copper cations (as CuSO4) dissolved in 70°C water at pH ≈12 by slow addition of formaldehyde. The samples were processed as reported in reference 12. Analysis of the data for the unannealed copper coated silicon powders showed a similar 29Si NMR spectrum to elemental bulk silicon supporting the conclusion that the local silicon environment remains for the most part unchanged after the copper deposition process and that formation of any silicon oxide/hydroxide/hydride species is minor and cannot be detected by MAS NMR. For comparison, X-ray powder diffraction data showed only elemental copper and silicon with small amounts of copper oxide (CuxO) for the most copper rich samples (Joyce et al., 2012; Dogan et al., 2013).
Data Analysis
After annealing under an argon atmosphere at various temperatures, several new silicon environments emerged during the 29Si NMR experiments that provided information about various silicon compounds present, notably for the high silicon content materials. The formation of these new silicon environments identified by MAS-NMR may arise from 1) higher population of copper-free silicon particles in silicon rich samples, 2) silicon being reactive (silicon oxides, hydroxides, or silicon hydrides) in the storage environment, 3) charge disproportionation of the copper cations, or 4) interfacial copper-containing grain boundary phases.
Various species identified in the study, such as the formation of silicon hydrides, are consistent with the third scenario where the deposition of copper onto a silica support and surface silicon is linked to a hydrogen spillover reaction (protons from water associated with the copper ions). Data analysis indicates that the electrode materials prepared with the lowest silicon (highest copper) content (Cu:4Si) and post treated below 600°C show only pure silicon environments, indicative of no Cu-Si reactivity, and the most stable cycling performance. Cu-Si electrode materials with higher silicon contents (Cu:6Si and Cu:8Si) but annealed at high temperatures near 700°C showed formation of multiple silicon local environments originating from both bulk and surface sites. These sites had a significant impact on the electrochemical performance of the materials as these new environments are at the critical interface in the system between the active electrode and the electrolyte. Figure 1 highlights the differences in the spectra. Analyzing the data collected, Cu-Si samples with lower amounts of silicon surface defects had superior electrochemical performance than those with more defects implying it is possible to improve the system electrochemical performance by minimizing the undesirable interfacial silicon local structures that may lead to more surface reactivity (i.e. thicker SEI formation). Analyzing the 29Si MAS NMR data and XRD data, the silicon-rich Cu:8Si compositions show formation of the phase Cu3Si, an electrochemically inactive phase, and the expected performance drop. While XRD data showed mainly crystalline phases, the MAS-NMR techniques identified several new Si environments that are assigned to various silicon oxides/hydrides as well as interfacial silicon atoms near and influenced by the copper (Joyce et al., 2012; Dogan et al., 2013).
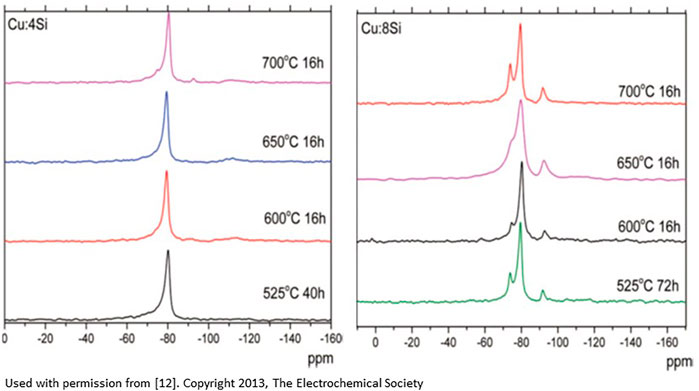
FIGURE 1. 29Si MAS NMR spectra on the effect of temperature treatment on the silicon local environment for Cu:4Si composition (left), and Cu:8Si composition (right). The extra peaks in the Cu:8Si are most likely due to the silicon within the copper-silicon matrix, interfacial silicon atoms, or silicon hydrides [From reference 12].
29Si and 7Li NMR Studies of Silicon-Based Electrodes Containing Li14MgSi4
In the previous section, 29Si MAS-NMR was used to identify poorly crystalline surface species that formed on annealing. The most common crystalline species, identified using X-ray diffraction, were elemental copper, silicon, and the bulk intermetallic phase Cu3Si. Intermediate phases, identified by MAS-NMR, included various silicon oxide, hydroxide, and hydride species that effected the cycling performance due to increased surface reactivity with the electrolyte as a function of charge. Building on the ability to study amorphous and nanoscale surface coatings, it was used to evaluate the products of an in-situ synthesis method that selectively coats the surface of an active silicon particle (BH Han et al., 2019a; BH Han et al., 2021; Li et al., 2021).
Background
For an LIB silicon electrode, a fully charged anode mainly contains the Zintl phase Li15Si4. Using Zintl-Klemm rules, the stoichiometry and properties of this phase can be understood as being composed of a combination of fully reduced four Si−4 anions, surrounded by 15 lithium cations per unit cell (Heider and Scheschkewitz, 2021). Using these rules, the phase is formally electron rich (exact charge balance would be 16 lithium cations) and is metastable at room temperature at this defect stoichiometry. On exposure to the constituents of an electrochemical cell, these electron rich phases are observed to react (formally oxidize) with the solvent and electrode binder to form various insoluble and electrolyte-soluble organic species (BH Han et al., 2019b) The exact oxidized silicon species (i.e. Li13Si4) depend on sample history and conditions. When considering the degradation mechanisms associated with full cell calendar life, this reactivity results in a parasitic current that effects the Coulombic efficiency of the cell. Under calendar life conditions that involve maintaining a specific cell voltage for extended time, the slightly oxidized silicon is reduced formally back to the electron non-precise Li15Si4 creating a redox cycle that degrades the electrolyte. To counter this cell reactivity and add stability, Mg+2 ions were added to the electrolyte. These ions move into the charged phase at low cell voltage formally to make the electrode surface electron precise by forming Li14MgSi4. The material, by Zintl-Klemm electron counting rules, is stoichiometric and (ideally) has a closed (electron) shell due to the extra electrons brought in by the Mg ions (Heider and Scheschkewitz, 2021). Creating these phases at the silicon/electrolyte interface adds stability to the cell and extends electrochemical cell cycle and calendar life (Zeilinger et al., 2013).
In a recent proof-of-concept study, Han et al., evaluated the stoichiometric Zintl phase Li2MgSi (an isostructural Mg-rich endmember of the solid solution with Li14MgSi4) against common organic cell materials (binder, solvents, salt) and observed the Si−4 anion was not obviously oxidized (as they were in the Li15Si4 study) and the enhanced stability was obtained (BH Han et al., 2021). This lack of reactivity, compared to the pure lithium silicide phases, diminishes the parasitic currents by a mechanism consistent with removing Si−3, Si−2, or Si−1 phases (as seen in the Li-Si binary system) from the reaction pathway. From the ternary Li-Mg-Si phase diagram, no equivalent intermediately charged cluster species exist as in the Li-Si system. Since elemental silicon is the next phase in the diagram, the transition requires a four-electron oxidation, which may represent a high kinetic barrier making the electrode surface more stable against the organic species in the electrochemical cell.
Data Analysis
To form a coating of these phases on the silicon in an electrochemical cell, small amounts of the salt Mg (TFSI)2 were added to the standard Gen2 electrolyte (1.2M LiPF6 EMC/EC 3:7). After cycling, TEM, EDX, MAS-NMR, and XPS studies showed surface Mg+2 at the approximate stoichiometric ratio of Li14.5Mg0.5Si4, a point in the solid solution between Li14MgSi4 and Li15Si4 that may represent a limit based on magnesium diffusion under the conditions used for the study (BH Han et al., 2019a; BH Han et al., 2021; Li et al., 2021). Spectroscopic evidence for interfacial phase formation is shown in Figure 2. With in-situ ternary salt formation at the surface, baseline Si electrode samples have extended cycle and calendar life compared to electrolytes without the additive.
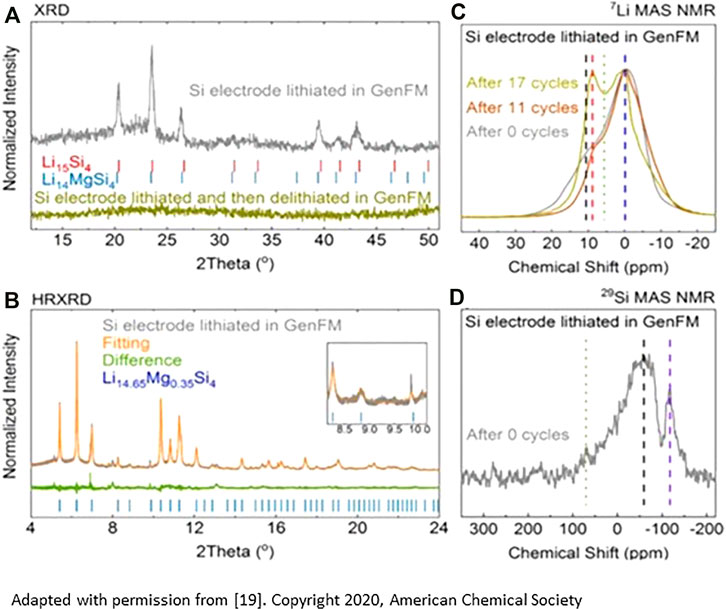
FIGURE 2. (A) Rietveld analysis from XRD data of the phases formed, (B) high resolution XRD of the cycled materials with stoichiometric refinement; (C) 7Li NMR study on cycled materials showing the growth of a second phase (Li-Mg-Si) on cycling; (D) 29Si MAS NNMR study highlighting formation of a second reduced silicon phase. Both spectra are consistent with gradual formation of a ternary Li-Mg-Si phase(s) on cycling in the Mg-containing electrolyte. [from reference 18]
The mechanism of ternary Zintl phase formation and its dynamics upon lithiation/delithiation were also studied with 29Si MAS NMR on Si electrodes harvested from cycled coin and pouch cells at various states of (de)lithiation, see Figure 3 (Li et al., 2021). The NMR data, along with other complimentary characterization techniques, reveal that lithiation of Si starts from the Si−O surface layer and progresses with heterogeneous silicon clustering with Si−4 anions at high states of lithiation. At a fully lithiated state, formation of an overlithiated Si species was also detected. At a low-voltage region (below 100 mV) and/or high lithiation levels, direct evidence for Mg-ion insertion is found, postulated by two possible mechanisms: ion exchange with fully or overlithiated binary domains (Li 3.75+xSi) and/or a co-insertion into slightly underlithiated domains (∼Li3.55Si).
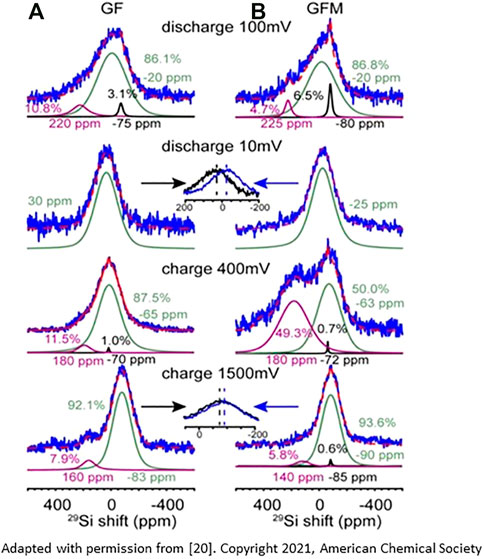
FIGURE 3. 29Si NMR spectra (solid line) and simulation/fitting (dash line) of GF (A) and GFM (B) at 100, 10, 400, and 1,500 mV. Peaks around −80 ppm are from unreacted crystalline Si, the broad resonance(s) at −15 ppm is from LixSi, whereas the peak around 220 ppm is ascribed to small Si clusters. When discharging to 100 mV, the majority of reacted Si is either in isolated Si or small Si clusters. For the fully lithiated samples, both GF (30 ppm) and GFM (−25 ppm) show one single broad peak with different chemical shifts. The shift difference is due to the presence of Li−Mg−Si phases. Upon charge to 400 mV, a significant amount (∼49.3%) of LixSi is observed in GFM cells, compared with only 11.5% residual Si in the GF cell. In the fully delithiated state, Si shifts of both GF (−83 ppm) and GFM (−90 ppm) cells shift toward lower frequencies. (Reproduced from Reference 20)
For silicon based systems, calendar life is an important performance issue as it reduces the cycle life and utility of lithium-ion cells. The use of 29Si MAS NMR was critical to identify the mechanism of Mg insertion, the role of time and diffusion, and the growth of the phase at that critical interface. Extending the calendar life of the silicon cell, by reducing the redox activity of the electrode surface, was difficult to characterize due to the nature of the coating. However the local probe aspect of MAS-NMR is capable of yielding insights many other techniques are incapable of providing to the researcher.
Silicate Reactivity
Although it does not have any appreciable redox reactivity in an electrochemical cell, the silica layer on silicon is chemically reactive with other SEI components and forms a variety of lithium silicates, ranging from the molecular species Li4SiO4 to the condensed material Li2SiO3. This chemical reaction is notable when evaluating the nanoscale Si/SiO2 composite “SiOx” as lithiation of the silica is a sink for active lithium and produces high irreversible capacity on the first cycle. Various approaches to overcome this reaction require pre-lithiation of the electrode to retain active lithium on cycling, however the active matrix produced is a relatively good lithium-ion conductor and produces an electrode with a stable cycling performance.
Background
Silicon is passivated quickly on exposure to air, water, or other oxygen containing species, to form a relatively dense silica coating that has a typical depth of 5 nm. This layer, difficult to detect with common lab XRD methods, acts to block additional reactions with an oxygen source and allows silicon to be handled and processed in air (Kale et al., 2019). Uses that require silica free materials require more stringent handling due to this oxyphilic nature of the silicon surfaces (Jiang et al., 2019; Schulze et al., 2021). In an electrochemical cell, it was noted by Edström et al., that this natural silica-based coating on silicon is lithiated early in the process forming a variety of lithium silicates, notably Li4SiO4 (Philippe et al., 2012; Philippe et al., 2013a). While no redox reactions occur, the lithiation is thought to occur as a reaction between the nanoscale silica and various species produced at the interface during the electrochemical silicon reduction (Hubaud et al., 2015). On long term cycling, these phases are not readily identifiable at the interface which may indicate reactions occur with SEI materials that form a soluble species or loss of interfacial cohesion in the cell.
Data Analysis
The Li4SiO4 was a commercially sourced powder from Alfa Aesar®. Lab XRD revealed a broad-peaked Li4SiO4 material. Small amounts of Li2SiO3 often seen in lab preps (from the oxides) could not be eliminated as a possible contaminant (i.e. from lithia loss on heating) due to similarities in the XRD spectra. NMR analysis of the as-received powder revealed a multiphase mixture, consistent with similar powders created in the lab. This sample analysis found the commercial material was a mixture of at least two silicate phases, one being relatively amorphous and thus difficult to detect in XRD experiments. Based on a mechanistic understanding of the formation of the second phase (probably Li2SiO3) it was probably nucleated on the surface phase of a crystalline Li4SiO4 (Philippe et al., 2012; Philippe et al., 2013a) Previous work has reported both Li2O and Li4SiO4 form initially when Li+ is inserted through a SiO2 native oxide on Si (Philippe et al., 2013b).
The commercial Li4SiO4 was stirred in a salt free mixture of ethylene carbonate and ethyl methyl carbonate (EC:EMC, 3:7 weight%) at room temperature for 48 h to test for solubility and reactivity. 29Si and 7Li solution NMR were performed to look for Si and Li species in the solvent after samples were filtered and spectra revealed the presence of lithium but no solubilized silicon in the electrolyte. Solid-state 29Si NMR was performed, on the as-received powder and powder that was in contact with the solvent for 2 days. The data are consistent with a decrease in the amount of Li4SiO4 and a corresponding increase in Li6Si2O7 and in Li2SiO3, indicating that the dissolving species from the electrode into the electrolyte may formally be Li2O or related (reaction product) species. The reaction mechanism is thought to be dimerization of the [SiO4]4- tetrahedra to form pyrosilicate [Si2O7]6-, then eventually the fully condensed Li2SiO3 linear chain material. Given longer exposure to the electrolyte, it is anticipated that expulsion of lithium oxide would continue depending on diffusion to the active surface, and possibly other intermediate or oligomeric species might be detected (Hubaud et al., 2015).
Conclusion
While diffusion is an important aspect of many commercial systems, methods to understand these properties at a mechanistic level are often limited to an understanding of crystalline pathways available to the diffusing species. In the examples noted, 29Si MAS NMR has been used to understand the role of amorphous or poorly crystalline species in reaction mechanisms and their evolution of these phases over time. In the examples of the active surface coatings, the diffusion of Mg+2 into Li15Si4 was critical in understanding the formation cycling issues associated with the emergence of Li14MgSi4-boundary coatings, the mechanism of Mg insertion into the bulk forming ternary Zintl phases, as well as the long-term advantages to important properties like calendar life. For the compound Li4SiO4, initially formed during the first charge for silicon containing anodes, these phases condense and eventually move into the cell environment by reaction, formally releasing lithia. Changes at this critical interface have an important role in an energy storage system, be it stabilizing the long cycle life anode SiOx, releasing of silicates gradually into the SEI layer, or tracking copper diffusion in silicon and understanding the role of minority surface species. Local probe techniques such as 29Si MAS NMR are critical tools to understand these differences in reactivity and the relationship with the electrodes performance.
Author Contributions
All authors listed have made a substantial, direct, and intellectual contribution to the work and approved it for publication.
Funding
The work at Argonne National Laboratory was supported by the U.S. Department of Energy, Office of Vehicle Technologies, under Contract No. DE-AC02-06CH11357. The submitted manuscript has been created by UChicago Argonne, LLC, Operator of Argonne National Laboratory (“Argonne”). Argonne, a U.S. Department of Energy Office of Science laboratory, is operated under Contract No. DE-AC02-06CH11357.
Conflict of Interest
The authors declare that the research was conducted in the absence of any commercial or financial relationships that could be construed as a potential conflict of interest.
Publisher’s Note
All claims expressed in this article are solely those of the authors and do not necessarily represent those of their affiliated organizations, or those of the publisher, the editors and the reviewers. Any product that may be evaluated in this article, or claim that may be made by its manufacturer, is not guaranteed or endorsed by the publisher.
Acknowledgments
The authors would like to thank Brian Cunningham, Stephen Boyd, Tien Duong, and David Howell from the Vehicle Technologies Program, at the U.S Department of Energy, Office of Energy Efficiency and Renewable Energy for their support. The Cu-Si electrode phases and the stability of silicate anions was funded under the Batteries for Advanced Transportation Technologies (BATT) Program, the work on in-situ coatings was funded as part of the Silicon Consortium Program (SCP). The electrodes in this article for the in-situ coatings work were fabricated at Argonne′s Cell Analysis, Modeling, and Prototyping (CAMP) Facility. This work used SEM and TEM in the Center for Nanoscale Materials and HRXRD in Sector 11-BM in the Advanced Photon Source, all at Argonne, which are supported by the U.S. Department of Energy, Office of Science, Office of Basic Energy Sciences, also under Contract No. DE-AC02-06CH11357. The authors thank Bob Jin Kwon, Xiang Li, Binghong Han, Christopher Joyce, and Ritesh Uppuluri at Argonne for experimental support.
References
Bh Han, C., Liao, F., Dogan, S., Trask, S., Lapidus, J., and Vaughey, B. (2019). Key “Using Mixed Salt Electrolytes to Stabilize Silicon Anodes for Lithium-Ion Batteries via In Situ Formation of Li-M-Si Ternaries (M = Mg, Zn, Al, Ca)”. ACS Appl. Mat. Interf. 11 (33), 29780–29790. doi:10.1021/acsami.9b07270
Bh Han, M. J., Piernas-Munoz, F. D., Kubal, J., Trask, S., Bloom, I., Vaughey, J., et al. (2019). “Probing the Reaction between PVDF and LiPAA vs Li7Si3: Investigation of Binder Stability for Si Anodes”. J. Electrochem. Soc. 166 (12), A2396–A2402. doi:10.1149/2.0241912jes
Bh Han, Y., Zhang, C., Liao, S., Trask, X., Li, R., Uppuluri, J., et al. (2021). Dogan “Probing the Reactivity of the Active Material of a Li-Ion Silicon Anode with Common Battery Solvents”. ACS Appl. Mat. Interf. 13 (24), 28017–28026. doi:10.1021/acsami.1c01151
Blanc, F., Leskes, M., and Grey, C. P. (2013). In Situ Solid-State NMR Spectroscopy of Electrochemical Cells: Batteries, Supercapacitors, and Fuel Cells. Acc. Chem. Res. 46 (9), 1952–1963. doi:10.1021/ar400022u
Chang, W. K., Liao, M. Y., and Gleason, K. K. (1996). J. Phys. Chem. 100, 19653. doi:10.1021/jp961921s
Delpuech, N., Dupre, N., Moreau, P., Bridel, J.-S., Gaubicher, J., Lestriez, B., et al. (2016). Mechanism of Silicon Electrode Aging upon Cycling in Full Lithium-Ion Batteries. ChemSusChem 9 (8), 841–848. doi:10.1002/cssc.201501628
Descemond, M., Brodhag, C., Thevenot, F., Durand, B., Jebrouni, M., and Roubin, M. (1993). Characteristics and Sintering Behavior of 3 Mol % Y203-Zr02 Powders Synthesized by Reaction in Molten Salts. J. Mat. Sci. 28, 2282–2288. doi:10.1007/bf01151654
Dogan, F., Joyce, C., and Vaughey, J. T. (2013). Formation of Silicon Local Environments upon Annealing for Silicon Anodes: A29Si Solid State NMR Study. J. Electrochem. Soc. 160 (2), A312–A319. doi:10.1149/2.069302jes
Dogan, F., and Vaughey, J. T. (2016). Effect of Surface Termination on Electrochemical Performance of Silicon Thin Films. J. Electrochem. Soc. 163, A62–A66. doi:10.1149/2.0261602jes
Gummow, R. J., Liles, D., and Thackeray, M. (1993). Spinel versus Layered Structures for Lithium Cobalt Oxide Synthesised at 400°C. Mater. Res. Bull. 28, 235–246. doi:10.1016/0025-5408(93)90157-9
Gummow, R., Liles, D., Thackeray, M., and David, W. (1993). A Reinvestigation of the Structures of Lithium-Cobalt-Oxides with Neutron-Diffraction Data. Mater. Res. Bull. 28, 1177–1184. doi:10.1016/0025-5408(93)90098-x
Heider, Y., and Scheschkewitz, D. (2021). Molecular Silicon Clusters. Chem. Rev. 121 (15), 9674–9718. doi:10.1021/acs.chemrev.1c00052
Hubaud, A. A., Yang, Z., Schroeder, D., Dogan, F., Trahey, L., and Vaughey, J. T. (2015). Interfacial Study of the Role of SiO2 on Si Anodes Using Electrochemical Quartz crystal Microbalance. J. Power Sourc. 282, 639–644. doi:10.1016/j.jpowsour.2015.02.006
Jiang, S. S., Hu, B., Sahore, R., Liu, H. H., Pach, G., Carroll, G. M., et al. (2019). ZC Zhang “Tailoring the Surface of Silicon Nanoparticles for Enhanced Chemical and Electrochemical Stability for Li-Ion Batteries”. ACS Appl. Energ. Mater 2 (9), 6176–6183. doi:10.1021/acsaem.9b01601
Joyce, C., Trahey, L., Bauer, S. A., and Dogan, F. (2012). Vaughey “Metallic Copper Binders for Lithium-Ion Battery Silicon Electrodes”. J. Electrochem. Soc. 159 (6), A909–A914. doi:10.1149/2.107206jes
Kale, A., Nemeth, W., Guthrey, H., Kennedy, E., Norman, A., Page, M., et al. (2019). Understanding the Charge Transport Mechanisms through Ultrathin SiOx Layers in Passivated Contacts for High-Efficiency Silicon Solar Cells. Appl. Phys. Lett. 114 (8), 083902. doi:10.1063/1.5081832
Kan, Y., Hu, Y., Ren, Y., Bareño, J., Bloom, I., Sun, Y.-K., et al. (2014). Differentiating Allotropic LiCoO2/Li2Co2O4: A Structural and Electrochemical Study. J. Power Sourc. 271, 97–103. doi:10.1016/j.jpowsour.2014.07.178
Kuhn, A., Narayanan, S., Spencer, L., Goward, G., Thangadurai, V., and Wilkening, M. (2011). Li Self-Diffusion in Garnet-type Li7La3Zr2O12 as Probed Directly by Diffusion-Induced7 Li Spin-Lattice Relaxation NMR Spectroscopy. Phys. Rev. B 83, 094302. doi:10.1103/physrevb.83.094302
Li, X., Gilbert, J., Trask, S., Uppuluri, R., Lapidus, S., Cora, S., et al. (2021). Investigating Ternary Li-Mg-Si Zintl Phase Formation and Evolution for Si Anodes in Li-Ion Batteries with Mg(TFSI)(2) Electrolyte Additive. Chem. Mater. 33 (13), 4960–4970. doi:10.1021/acs.chemmater.1c00616
Mackenzie, K. J. D., and Smith, M. E. (2002). Multinuclear Solid-State NMR of Inorganic Materials. in Pergamon Material Series, Vol. 6. Oxford: Pergamon.
Michan, A. L., Leskes, M., and Grey, C. P. (2016). Voltage Dependent Solid Electrolyte Interphase Formation in Silicon Electrodes: Monitoring the Formation of Organic Decomposition Products. Chem. Mater. 28 (1), 385–398. doi:10.1021/acs.chemmater.5b04408
Petit, D., Chazalviel, J. N., Ozanam, F., and Devreux, F. Appl. Phys. Lett. 70 (2), 13. doi:10.1063/1.118382
Philippe, B., Dedryvere, R., Allouche, J., Lindgren, F., Gorgoi, M., Rensmo, H., et al. (2012). Nanosilicon Electrodes for Lithium-Ion Batteries: Interfacial Mechanisms Studied by Hard and Soft X-ray Photoelectron Spectroscopy. Chem. Mater. 24 (6), 1107–1115. doi:10.1021/cm2034195
Philippe, B., Dedryvere, R., Gorgoi, M., Rensmo, H., Gonbeau, D., and Edstrom, K. (2013). Role of the LiPF6 Salt for the Long-Term Stability of Silicon Electrodes in Li-Ion Batteries - A Photoelectron Spectroscopy Study. Chem. Mater. 25 (3), 394–404. doi:10.1021/cm303399v
Philippe, B., Dedryvère, R. M., Gorgoi, M., Rensmo, H. K., Gonbeau, D., and Edstrom, K. (2013). Improved Performances of Nanosilicon Electrodes Using the Salt LiFSI: A Photoelectron Spectroscopy Study. J. Am. Chem. Soc. 135, 9829–9842. doi:10.1021/ja403082s
Pietraβ, T., Bifone, A., Roth, R. D., Koch, V. P., Alivisatos, A. P., and Pines, A. (1996). 29Si High Resolution Solid State Nuclear Magnetic Resonance Spectroscopy of Porous Silicon. Journalof Non-Crystalline Sol. 202, 68–76. doi:10.1016/0022-3093(96)00144-5
Schulze, M., Carroll, G. M., Martin, T., Sanchez-Rivera, K., Urias, F., and Neale, N. R. (2021). Hydrophobic versus Hydrophilic Interfacial Coatings on Silicon Nanoparticles Teach Us How to Design the Solid Electrolyte Interphase in Silicon-Based Li-Ion Battery Anodes. ACS Appl. Energ. Mater 4 (2), 1628–1636. doi:10.1021/acsaem.0c02817
Shi, B., Gim, J., Li, L., Wang, C., Vu, A., Croy, J. R., et al. (2021). Eungje Lee “LT-LiMn0.5Ni0.5O2: a Unique Co-free Cathode for High Energy Li-Ion Cells. Chem. Commun. 57, 11009–11012. doi:10.1039/d1cc04334j
van der Ven, A., Bhattacharya, J., and Belak, A. A. (2013). Understanding Li Diffusion in Li-Intercalation Compounds. Acc. Chem. Res. 46 (5), 1216–1225. doi:10.1021/ar200329r
Zeilinger, Michael., Baran, Volodymyr., Leo van Wüllen, , Ulrich, Häussermann., and Thomas, F. (2013). “Stabilizing the Phase Li15Si4 through Lithium−Aluminum Substitution in Li15−xAlxSi4 (0.4 < X < 0.8 - Single Crystal X-ray Structure Determination of Li15Si4 and Li14.37Al0.63Si4”. Chem. Mater. 25, 4113–4121. doi:10.1021/cm402721n
Keywords: silicon, NMR, silicates, zintl, electrochemistry, energy storage
Citation: Dogan F, Key B and Vaughey JT (2022) Utilization of 29Si MAS-NMR to Understand Solid State Diffusion in Energy Storage Materials. Front. Chem. Eng. 4:833162. doi: 10.3389/fceng.2022.833162
Received: 10 December 2021; Accepted: 28 January 2022;
Published: 17 February 2022.
Edited by:
Alexander Roberts, Coventry University, United KingdomReviewed by:
Congrui Jin, University of Nebraska-Lincoln, United StatesSteven G. Greenbaum, Hunter College (CUNY), United States
Copyright © 2022 Dogan, Key and Vaughey. This is an open-access article distributed under the terms of the Creative Commons Attribution License (CC BY). The use, distribution or reproduction in other forums is permitted, provided the original author(s) and the copyright owner(s) are credited and that the original publication in this journal is cited, in accordance with accepted academic practice. No use, distribution or reproduction is permitted which does not comply with these terms.
*Correspondence: John T. Vaughey, vaughey@anl.gov