- 1Department of Energy Systems Engineering, Seoul National University, Seoul, South Korea
- 2Research Institute of Energy and Resources, Seoul National University, Seoul, South Korea
In an enhanced geothermal system (EGS), geothermal energy in rocks with insufficient permeability or fluid saturation can be used by creating artificial geothermal reservoirs. Generally, EGS geothermal fluid contains high concentrations of total dissolved solids that originated from various geochemical reactions between the fluid in the reservoir and the minerals in the rock. For example, the concentration of lithium ions are measured approximately 150 mg/L, and several researchers have focused on the recovery of lithium in the geothermal fluid using various methods, one of which is liquid extraction. Solvent extraction has been used to recover lithium from various sources, and successful recovery efficiency have been attained. However, the geothermal fluid in EGS reservoirs contains high concentrations of SiO2, which might inhibit the selective recovery of lithium. Thus, in this study, two consecutive stages of solvent extraction were used to separate the lithium from the geothermal fluid that contained different concentrations of SiO2 ions. The divalent ions were removed in the first stage, and the lithium ions were extracted effectively in the second stage. The SiO2 inhibits the selective recovery of lithium in the first stage to a greater extent than it does in the second stage. The spectroscopy data shows a decrease of the organic solvents main functional group (P=O & P-O-H) absorbance that reacts with the metal ions of the geothermal water after extraction however the intensity difference was reduced as the SiO2 concentrations increases. Silicate ions can be problematic due to the formation of scaling in EGSs, so controlling its concentration in the geothermal reservoir would be beneficial for the long-term operation of EGSs and for the successful recovery of valuable metal resources from EGS reservoirs.
1 Introduction
Geothermal energy is known to be thermal energy that is contained inside the Earth (Barbier, 2002), and geothermal systems use this geothermal energy to generate electricity (Olasolo et al., 2016). Enhanced geothermal systems (EGSs) are one of the geothermal systems used to extract thermal energy from hot dry rock (HDR) (Lei et al., 2019). EGS technology has been developed in several countries for more than 40 years, and it is known to be a source of clean renewable energy (Lu, 2018). EGSs apply hydraulic stimulation at depths deeper than 3 km of HDR to create an artificial geothermal reservoir for a sustainable geothermal system (Hofmann et al., 2014; Kim et al., 2018). Water is injected through an injection well where the thermal energy is stored in the deep fractured network, and it comes out through the pumping well with thermal energy (Caulk et al., 2016; Hofmann et al., 2019; Lee and Chung., 2020a). The heated water passes through a geothermal heat exchanger where the heat is used to generate electricity.
During the circulation of a geothermal fluid or hydraulic stimulation, the chemical composition of the fluid changes abruptly compared to the initial stage. The composition changes originate from various geochemical reactions in the geothermal reservoir (Owen et al., 2020; Lee and Chung, 2020a). As described in the article by Pauwels et al. (1992), the total dissolved solids (TDS) of the production fluid of the Soultz-sous-Forêts EGS increase due to a short duration injection test, and lithium ions especially are increased in this site. Lithium is an essential metal that is used in many industries, such as ceramics, glass, rubber, and batteries (U.S. Geological Survey, 2018), and the demand for lithium is estimated to increase due to its use in electric vehicles and batteries (An et al., 2012; Swain, 2017; Xu C. et al., 2020). Usually, lithium is obtained from lithium-rich brines (as dissolved lithium chloride (LiCl)) and from lithium-bearing minerals, such as petalite and lepidolite (Flexer et al., 2018). The separation of lithium ions from massive volumes of seawater, which has an average lithium concentration of 0.17 mg/L, has been conducted in many studies (Nishihama et al., 2011; Harvianto et al., 2016; Li et al., 2018). Compared to the low average concentration of lithium in seawater, the geothermal fluid that comes from the Soultz-sous-Forêts EGS has a relatively high lithium content (approximately 150 mg/L) after its long-term circulation from the GPK-2 production well (Scheiber et al., 2012). The concentration of lithium ions in the geothermal water in the Soultz-sous-Forêts EGS is higher than the worldwide concentrations (i.e., 1–100 mg/L) reported by Flexer et al. (2018), so it provides many economic advantages in selectively recovering lithium ions.
Several studies of selective lithium recovery from various solutions including geothermal brines have been reported, including adsorption, ion exchange, electrochemical extraction, and solvent extraction (Han et al., 2014; Yen et al., 2016; Jang and Chung, 2019; Battistel et al., 2020; Warren, 2021). Many researchers applied the adsorption and the ion exchange methods to recover lithium ions from various solutions, and many of them showed significantly selective lithium recovery results (Braun et al., 2002; Kumar et al., 2017; Wang et al., 2017; Jang and Chung, 2018; Goc et al., 2021; Warren, 2021). However, since the use of the oxidant or acid is essential for a desorption process, it might not be economical to recover lithium using adsorption in a large scale (He et al., 2018; Xu W. et al., 2020). Also, for the ion exchange method, fouling such as calcium sulfate can occur during a regeneration process due to the significant amount of calcium ions in the geothermal fluid (Scheiber et al., 2012; Wachinski, 2016). The electrochemical method uses lithium-selective electrodes like λ-MnO2 or HFePO4 to capture the lithium ions from solutions (Kim et al., 2021; Wang et al., 2021). The electrochemical extraction method showing the high lithium selectivity does not require the chemicals such as organic solvent or acid. However, high energy consumption is expected due to the application of high voltage during the operation (Kanoh et al., 1993; Battistel et al., 2020; Joo et al., 2020; Kim et al., 2021). The solvent extraction method uses the transfer of a compound from one liquid phase to another based on the different solubility or distribution coefficients of the compound (Chen and Wang, 2016). In this study, the solvent extraction or liquid-liquid extraction method was tested due to the simplicity of the process and the relatively short operation time (Yang et al., 2003; Masmoudi et al., 2021). In many studies, it was shown that the contact time of less than an hour (few minutes at least) was enough for the process (Yang et al., 2003; Silva et al., 2005; Jin et al., 2014). Also, the recyclability of the solvent makes this method beneficial. Based on the advantages described above, the solvent extraction method is used extensively in industrial applications to recover valuable metal ions from solutions (Yen et al., 2016). Many researchers have used various solvents to extract specific metal ions from aqueous solutions (Sadakane et al., 1975; Umetani et al., 1987; Hano et al., 1992). In previous research, D2EHPA was used to recover manganese from a solution based on lithium-ion batteries (Vieceli et al., 2021), and strontium was extracted from the leach liquor of ore by using a crown ether (18-crown-6) (Alamdar Milani et al., 2021). Due to different functional groups or properties of solvents, solute-solvent interactions and the distribution ratio can be affected, resulting in a different cation affinity (Kislik et al., 2003; Kislik and Eyal. 2003). In Soultz-sous-Forêts geothermal water, various divalent cations and silicate ions exist, and they can have an adverse effect on the efficiency of lithium extraction. D2EHPA (Di-(2-ethylhexyl)phosphoric acid, C16H35O4P) is well known as an extractant for its higher affinity for divalent cations than for monovalent cations (i.e., Ca2+ > Sr2+ > Mg2+ > Li+ > Na+) (Hano et al., 1992; Jang et al., 2017). In a study by Jang et al. (2017), two-step liquid extraction was used to selectively extract lithium ions from shale gas produced water. The first step was proposed to remove most of the divalent ions with a low lithium concentration extraction, while the second step was used to extract lithium ions selectively (Lee and Chung, 2020b). Due to a similar chemical composition especially for the cation concentration in geothermal water, the two-step solvent extraction process was used in this research to recover lithium selectively.
Geothermal water has a high TDS, including silicate ions, which usually is produced by geochemical reactions of silicate minerals (Lee and Chung, 2021). A high concentration of silicate ions (130–409 mg/L) was reported for the GPK-2 production well of the Soultz-sous-Forêts EGS on different sampling dates (Sanjuan et al., 2006). Due to a high concentration of silicate ions dissolved in the fluid, the precipitation of silicate minerals can occur in the pipe or reservoir during the circulation of the fluid, so fracture closure can decrease the permeability of the EGS (Sanjuan et al., 2010; Putera et al., 2018; Lee and Chung, 2020a). Not only is dissolved silicate fatal for the EGS process due to the scaling formation, high concentrations of dissolved silicate ions also have been reported to possibly inhibit the extraction efficiency of metal ions (Hano et al., 1992). Accordingly, several studies have reported methods to remove dissolved silicate from solutions (Putera et al., 2018; Spitzmüller et al., 2021), the relationship between the existence of silicate ions and the specific metal extraction efficiency during solvent extraction has not been researched fully. Therefore, in this study, different concentrations of silicate ions were tested in geothermal water to investigate their interaction and influence during lithium recovery from solvent extraction.
2 Materials and Methods
2.1 Sample Preparation
Soultz-sous-Forêts geothermal water was synthesized using various chemicals to set the concentration of major cations, i.e., Na+, Ca2+, K+, Li+, Mg2+, Sr2+, and Ba2+, for a chemical composition similar to that of actual geothermal fluid (Scheiber et al., 2012). The main chemical properties of synthetic geothermal water are shown in Table 1.
After synthesizing geothermal water, solutions with different concentrations of dissolved SiO2 were added to observe the lithium extraction efficiency. The content of Si ions is measured and converted into SiO2 content that can be converted to a soluble form of silica in the geothermal water. In this study, 0, 150, and 350 mg/L of dissolved SiO2 solutions were put to the geothermal water, and they were classified as samples A, B, and C in this study. As shown in the previous studies by authors, high TDS concentration of a solution might inhibit the efficient lithium recovery during solvent extraction process (Jang et al., 2017) and solvent extraction was applied in a 50× diluted shale gas produced water of which the TDS concentration was up to 157,000 mg/L. Soultz-sous-Forêts geothermal water has a high TDS value, i.e., approximately 100,000 mg/L (Scheiber et al., 2012), therefore, three different dilution rates for the synthetic geothermal fluid were tested. The results were provided in Supplementary Material. When the original geothermal fluid was tested, most of the divalent ions showed a low removal efficiency (<40%). For In the 25× diluted geothermal water, Sr2+ and Ba2+ showed > 90% removal efficiency and Mg2+ showed 84% removal efficiency. When the solvent extraction method was applied to the 50× and diluted geothermal water, all divalent ions showed significantly higher removal efficiency than for the 25× diluted geothermal water, especially, Sr2+ and Ba2+ showed removal efficiencies greater than 97%. In both 25× and 50× diluted geothermal water, Ca2+ showed the highest removal efficiency (close to 100%) and Li+ showed removal efficiency about 69–70%. Based on the results, it was concluded that the 50× diluted geothermal water was optimal to be tested for the lithium recovery from geothermal fluid.
2.2 Experimental Methods
In all stages of the solvent extraction, D2EHPA (97%, Sigma Aldrich, St. Louis, MO, United States) was used as an extractant, and kerosene (Sigma-Aldrich, St. Louis, MO, United States) was used as a diluent to control the molarity of the organic phases. During the solvent extraction process, the ratio of the organic phase to the aqueous phase was 1:1, and the two phases were mixed using a shaking incubator (SH-BSI16R, Samheung Instrument, Korea). The shaking speed and temperature were set to 150 rpm and 25°C, respectively, for 30 min. After mixing the organic and aqueous phases, the two-phase solution was equilibrated until the two phases were separated in a separating funnel. The aqueous solution was reused for repetitive extractions up to four times to improve the efficiency of the removal of the divalent cations and fresh organic solvents (1.0 M D2EHPA) were applied in every repetitive extraction. The process of the second stage was similar to the previous one but TBP (Tributyl phosphate, C12H27O4P, 98.5% purity, Daejung Co., Siheung, Korea) was used for lithium recovery in the mixture of D2EHPA and kerosene as an additive. Fresh organic solvent (1.5 M D2EHPA + 0.3 M TBP) was used in every repetition and the aqueous solution was reused for repetitive extractions.
After every extraction cycle, 10 ml of the aqueous phase was withdrawn and filtered with a 0.45 μm polytetrafluoroethylene (PTFE) filter (Millipore, Germany) to remove particulates. The permeate was then analyzed cations and anions by inductively coupled plasma optical emission spectrometry (ICP-OES, Optima 8300, Perkin Elmer, United States) and ion chromatography (IC, Dionex ICS-1100, Thermo Scientific, United States). The pH level was measured using a multimeter (Orion Star A329, Thermo Fisher Scientific, United States). The organic solution was analyzed by Fourier transform infrared spectroscopy (FT-IR spectra, Nicolet 6700, Thermo Fisher Scientific, Waltham, MA, United States) to analyze the changes in the absorbances of the major functional groups.
According to Hano et al. (1992), the chemical reaction of D2EHPA (HR) and metal ion (Mn+) can be described as Eq. 1 (x: solvation number of complex)
A chelate (metal-extractant complex) can be formed during the interaction of deprotonated D2EHPA and metal ions, and the metal ions move from the aqueous phase to the organic phase (Lee et al., 2011). A proper amount of TBP would act as a synergistic reagent in the mixture of D2EHPA, TBP, and metals to increase the extraction efficiency of metal ions, and this reaction can be described as Eq. 2 (Hano et al., 1992; Amani et al., 2017).
One molecule of D2EHPA from the D2EHPA-metal complex can be replaced with one molecule of TBP to react with another metal ion to increase the extraction efficiency.
The extraction efficiency of each metal ion is calculated by Eq. 3 (Jafari et al., 2018):
In Eq. 3, the concentration of a specific metal ion in the aqueous phase before solvent extraction is [C]in,aq, and the concentration of a specific metal ion in the aqueous phase after solvent extraction is [C]fin,aq.
Distribution ratio (Dm) is the ratio of the concentration of the metal ion in the organic phase to the concentration of the metal ion in the aqueous phase and is calculated by Eq. 4 (Ganji et al., 2016).
The separation factor of a specific metal ion (M1) over another metal ion (M2) in the extraction was calculated by the distribution ratio (Dm), as described by Eq. 5 (Jang et al., 2017).
3 Results and Discussion
3.1 First Step of Solvent Extraction for the Extraction of Divalent Ions
The influence of different dissolved SiO2 concentrations (0, 150, 350 mg/L) in geothermal water was observed during the solvent extraction process of metal ions. Figure 1 shows the extraction efficiency of cations (Ca2+, Sr2+, Mg2+, Li+, Na+) in geothermal fluid, with different dissolved SiO2 concentrations for four extraction processes. Ba2+ was not drawn in the figure due to its lower concentration (<0.4 mg/L) in the initial fluid after 50× dilution. The pH of the aqueous solution was measured after every repetition extraction process, and the range of the pH values was 1.8–2.1 due to the production of hydrogen ions, as described in Eq. 1.
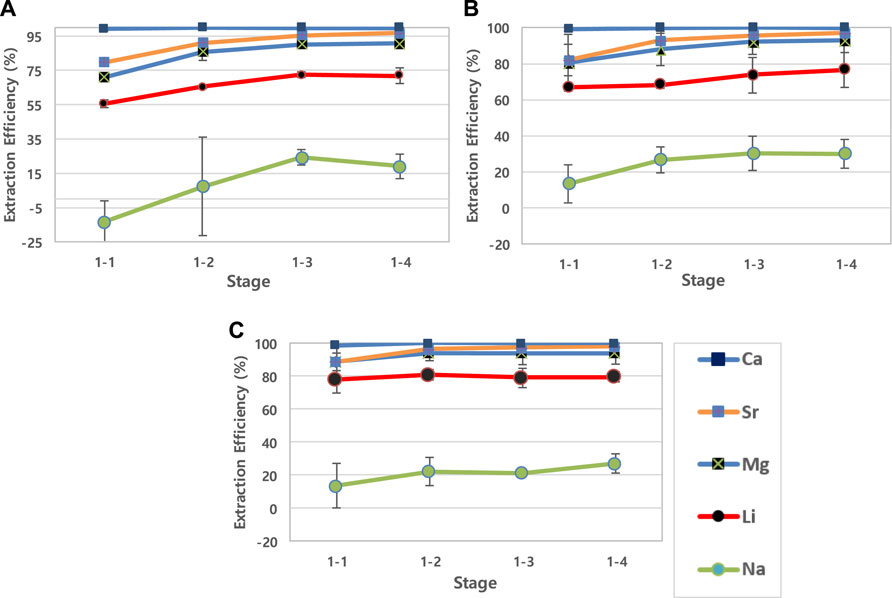
FIGURE 1. 1st step removal efficiency of cations in different SiO2 concentrations of geothermal water. (Sample (A)-SiO2 0 mg/L; Sample (B)-SiO2 150 mg/L; Sample (C)-SiO2 350 mg/L).
The removal efficiencies of Ca2+, Mg2+, and Sr2+ were greater than 90% after four repetitions in all three samples. The removal rates of SiO2 were also calculated after each step and the values were significantly low. For sample A and C, the removal rates of silicate ions from the aqueous side were 0% and, for sample B, the removal rate was measured as about 7% after 4 repetitions. D2EHPA affinity for cation extraction has been reported to be in the order of Ca2+ > Sr2+ > Mg2+ > Li+ > Na+ (Hano et al., 1992; Jang et al., 2017), and all the cations tendency satisfied in three samples. For example, as D2EHPA shows the highest affinity for Ca2+ compared to other divalent ions (Hano et al., 1992; Jang et al., 2017), the removal efficiency showed the highest values in all conditions as shown in Figure 1.
The removal efficiency of cations including lithium increased as the SiO2 concentration increased. Especially, the lithium removal efficiency in solution A at the first extraction was 55.5%, and the efficiency increased to 66.8 % and 77.9% in solutions B and C, respectively. To observe the effect of dissolved SiO2 in the solution, distribution ratio of the cations were calculated for the first (1-1) extraction (Table 2). The DNa values in all samples indicate that the extraction of Na+ was not significant during the first solvent extraction. The distribution ratio of Ca2+ showed a decrease while the SiO2 concentration increases but the other divalent ions (Sr2+ and Mg2+) and Li+ showed higher distribution ratio in higher SiO2 concentration solution.
After four repetitive stages of extraction, more cations were extracted from the aqueous phase and the distribution ratio of cations increased. The separation factors of three divalent ions over lithium after four stages were calculated as shown in Table 3. Ca2+ had the highest selectivity factors in the geothermal water without SiO2, and the selectivity increased when the SiO2 concentrations increased in the solution. However, Sr2+ and Mg2+ showed slight decreased separation factors with the increase of SiO2 concentrations.
The absorbance of the metal-D2EHPA complex in the organic phase was analyzed using FT-IR to observe the change in the functional group during solvent extraction (Figure 2). The result for D2EHPA before the extraction is shown in red line, and the sample A, B, and C results are shown in Figures 2A–C, respectively. Due to the formation of a metal-D2EHPA complex during the extraction with no SiO2 ions (sample A), the absorbance of the P=O bond (1034 cm−1) and the P-O-H bond (1230 cm−1) decreased after extraction (Figure 2A). It seems that the metal ion and an electronegative P=O bond from D2EHPA form a bond, and a hydrogen from the P–O–H bond is replaced with a metal ion. Figure 2A shows that the wavenumbers for the absorbance peaks increase as the SiO2 concentrations increased, which indicates that the existence of the SiO2 ions interferes with the loading of the metal ion during the solvent extraction.
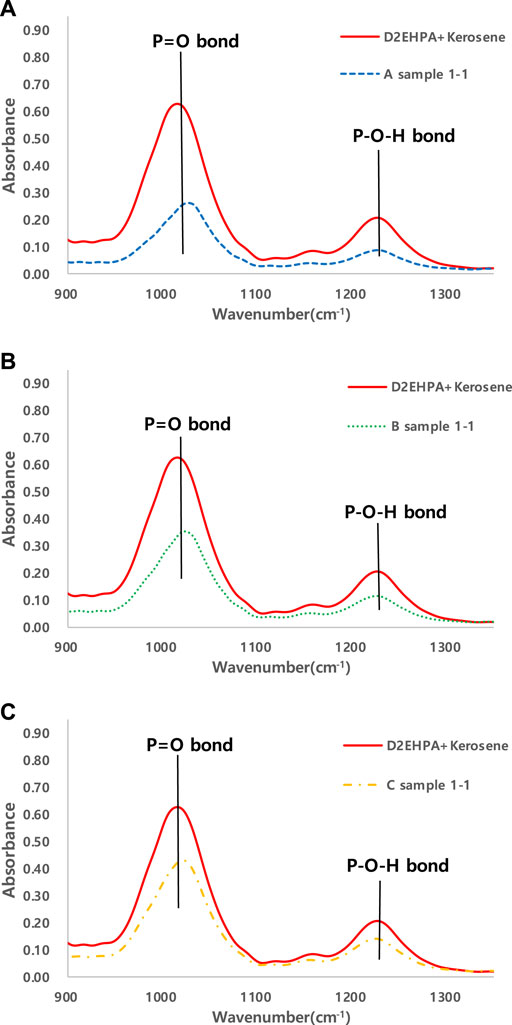
FIGURE 2. FT-IR spectra of samples (A) D2EHPA + Kerosene & D2EHPA + Kerosene after the 1st stage solvent extraction of the geothermal water without SiO2; (B) D2EHPA + Kerosene & D2EHPA + Kerosene after the 1st stage of solvent extraction of the geothermal water that contained 150 ppm of SiO2; (C) D2EHPA + Kerosene & D2EHPA + Kerosene after the 1st stage of solvent extraction of the geothermal water that contained 350 ppm of SiO2.
Although the formation of metal-D2EHPA complex was slightly inhibited by the SiO2 ions in the aqueous fluid (Figure 2), more metal ions were extracted from the aqueous solution in the existence of the SiO2. One of the explanation for that could be the silica polymerization. The silanol group (Si-O-H) originated from dissolved SiO2 in geothermal water can form polymeric, colloidal, and particulate silica due to various conditions, such as pH or the presence of other ions (Park et al., 2020). The polymerization of silicic acid occurs rapidly in neutral or slightly alkaline pH values and is formed slowly below pH 6.5 (Putera et al., 2018; Park et al., 2020). Due to a low pH solution (1.8–2.1) after every repetition extraction process, the polymerization of silicic acid actually occurs probably at a slow rate. With other divalent cations, silica polymerization can be formed because silicic acid is classified as a weak acid in geothermal water (Brown, 2013; Park et al., 2020).
3.2 Second Step of Solvent Extraction for Lithium Ion Extraction
After removing the divalent cations from the geothermal water in the first extraction step, lithium was extracted during four repetitive cycles in the second step (Figure 3). The [C]in,aq value in Eq. 3 for all data points (2-1, 2-2, 2-3 and 2-4) is the concentration value measured after the stage 1–4 is completed, and the [C]fin,aq value implies the concentration measured after each stage. Li+ had a higher extraction efficiency than the other monovalent cation, Na+, for all three samples and the Li+ extraction efficiency ranged from 21.9 to 24.8%. The separation factor of Li+ over Na+ could not be calculated due to the negative extraction efficiency of Na+ ions. The separation factor of Li+ over Na+ could not be calculated due to the negative extraction efficiency of Na+ ions. Jang (2016) explained that the effect of TBP addition can dissolve the hydrated complex (HRn ∙ xH2O) that was not extracted in the previous extraction stage. If the coordination number of the metal ion is not satisfied to load from the aqueous phase to the organic phase during the extraction reaction, the metal ions can be stocked with water molecules with a creation of a hydrated complex (HRn ∙ xH2O) (Tanaka and Akaiwa, 2009). The Na+ showed relatively low extraction efficiency in the first stage due to the formation of a hydrated chelate complex with D2EHPA. TBP molecules have a low affinity with Na+ ions and the metal chelate complex (HRn ∙ xH2O) can be resolved in the solution by the addition of TBP, which might cause an increase of Na+ ions concentration (Jang, 2016).
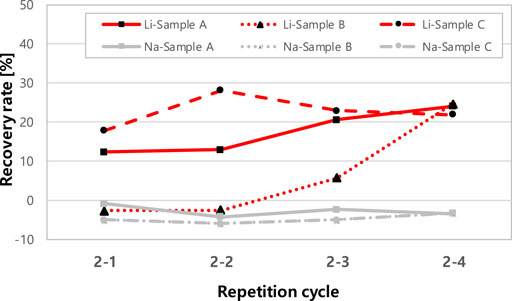
FIGURE 3. 2nd step removal efficiency of Li and Na in different SiO2 concentrations of geothermal water.
After removing most of the divalent ions in the first step, the overall extraction efficiency of Li was calculated as shown in Figure 4. In the first stage extraction, 71.9–79.50% of the original lithium was lost and 21.9–24.8% of the remaining lithium was recovered during the second stage extraction. Therefore, the overall lithium recovery rate was calculated as 4.5–6.8%. The overall recovery of Li+ decreased from 6.8 % to 4.5% as the SiO2 concentration increased. The difference between the Li+ recovery rates depends mainly on the loss of Li+ in the first step of the extraction process.
A certain amount of the geothermal fluid is generally lost in EGSs during the circulation of the fluid, and less than 10% of the fluid loss would make the long-term operation of the system possible (Clark et al., 2013; Schill et al., 2017). Therefore, in some cases, the input of new fluid such as river or lake water near the site would be required to operate the system properly. In this study, it was shown that 50× dilution was favorable for the lithium extraction in the geothermal water. If 0.2% of the geothermal water is used for the lithium extraction, the volume of the solution after the extraction will be close to 10% of the original geothermal water volume. If further study shows that the lithium recovery is feasible from the diluted geothermal fluid with the freshwater near the EGSs, the simultaneous lithium recovery and EGS operation without fluid loss might be possible.
4 Conclusion
Solvent extraction was implemented in two repetitive stages for the recovery of Li+ from geothermal fluid. The first stage of solvent extraction was used to remove divalent cations, and the second stage was to recover Li+ selectively from the geothermal water. During the two step solvent extraction process, the total lithium recovery efficiency decreased from 6.8 % to 4.5% as the SiO2 ion concentrations increased. As the concentration of SiO2 ions increased from 0 mg/L to 350 mg/L in the geothermal water, the loss of Li ions from the aqueous phase in the first step increased from 55.5% to 77.9% and the Li recovery rates in the second step did not show significant difference (21.9–24.8%) in different SiO2 concentrations. Accordingly, the overall Li + recovery efficiency has been decreased and it indicates that the influence of SiO2 on the Li+ recovery occurs mainly in the first step of the extraction process. The separation factor of divalent ions such as Sr2+ and Mg2+ over Li+ decreased as increased SiO2 concentration because greater amounts of Sr2+ and Mg2+ have been extracted with the existence of SiO2 ions in the geothermal fluid. In this study, 50× dilution was preferable to extract the lithium ions efficiently so 50 times greater volume of the solution was produced after the extraction. The solution could be added, if necessary, to the geothermal fluid for an effective EGS operation. It is well known that SiO2 ions in the geothermal fluid can cause the scaling problem in geothermal systems and it was found, in this study, that SiO2 ions also inhibits the selective recovery of lithium from the geothermal water. Therefore, controlling the concentrations of SiO2 ions in geothermal reservoirs is suggested for the efficient operation of geothermal systems and successful recovery of lithium from the geothermal fluid.
Data Availability Statement
The original contributions presented in the study are included in the article/Supplementary Material, further inquiries can be directed to the corresponding authors.
Author Contributions
All authors listed have made a substantial, direct, and intellectual contribution to the work and approved it for publication.
Funding
This research was supported by Korea Electric Power Corporation (Grant number: R18XA06-37).
Conflict of Interest
The authors declare that the research was conducted in the absence of any commercial or financial relationships that could be construed as a potential conflict of interest.
Publisher’s Note
All claims expressed in this article are solely those of the authors and do not necessarily represent those of their affiliated organizations, or those of the publisher, the editors and the reviewers. Any product that may be evaluated in this article, or claim that may be made by its manufacturer, is not guaranteed or endorsed by the publisher.
Supplementary Material
The Supplementary Material for this article can be found online at: https://www.frontiersin.org/articles/10.3389/fceng.2022.741281/full#supplementary-material
References
Alamdar Milani, S., Hafizi, M., Abolghasemi, H., and Zahakifar, F. (2021). Solvent Extraction of Strontium from Leach Liquor of central iran Metasomatic Ores Using Macrocyclic crown Ether Ligands. J. Water Wastewater 32 (2), 68–79. doi:10.22093/WWJ.2020.230420.3019
Amani, P., Asadi, J., Mohammadi, E., Akhgar, S., and Esmaili, M. (2017). Cooperative Influence of D2EHPA and TBP on the Reactive Extraction of Cobalt from Sulfuric Acid Leach Solution in a Horizontal Semi-industrial Column. J. Environ. Chem. Eng. 5 (5), 4716–4727. doi:10.1016/j.jece.2017.08.044
An, J. W., Kang, D. J., Tran, K. T., Kim, M. J., Lim, T., and Tran, T. (2012). Recovery of Lithium from Uyuni Salar Brine. Hydrometallurgy 117-118, 64–70. doi:10.1016/j.hydromet.2012.02.008
Barbier, E. (2002). Geothermal Energy Technology and Current Status: an Overview. Renew. Sustain. Energ. Rev. 6 (1–2), 3–65. doi:10.1016/s1364-0321(02)00002-3
Battistel, A., Palagonia, M. S., Brogioli, D., La Mantia, F., and Trócoli, R. (2020). Electrochemical Methods for Lithium Recovery: A Comprehensive and Critical Review. Adv. Mater. 32 (23), 1905440. doi:10.1002/adma.201905440
Braun, A., Evans, D., Gandhi, P. M., Garamszeghy, M., Geiser, H., Hooper, E. W., et al. (2002). Application of Ion Exchange Processes for the Treatment of Radioactive Waste and Management of Spent Ion Exchangers. Technical Reports Series. Vienna: International Atomic Energy Agency.
Brown, K. (2013). Mineral Scaling in Geothermal Power Production. Reports 2013-39. Reykjavik, Iceland: United Nations University Geothermal training program, 1–25.
Caulk, R. A., Ghazanfari, E., Perdrial, J. N., and Perdrial, N. (2016). Experimental Investigation of Fracture Aperture and Permeability Change within Enhanced Geothermal Systems. Geothermics 62, 12–21. doi:10.1016/j.geothermics.2016.02.003
Chen, H., and Wang, L. (2016). Technologies for Biochemical Conversion of Biomass. 1st edition. Academic Press, 200–202.
Clark, C. E., Horner, R. M., and Harto, C. B. (2013). Life Cycle Water Consumption and Water Resource Assessment for Utility-Scale Geothermal Systems: An In-Depth Analysis of Historical and Forthcoming EGS Projects. United States: U.S. Department of Energy.
Flexer, V., Baspineiro, C. F., and Galli, C. I. (2018). Lithium Recovery from Brines: A Vital Raw Material for green Energies with a Potential Environmental Impact in its Mining and Processing. Sci. Total Environ. 639, 1188–1204. doi:10.1016/j.scitotenv.2018.05.223
Goc, K., Kluczka, J., Benke, G., Malarz, J., Pianowska, K., and Leszczyńska-Sejda, K. (2021). Application of Ion Exchange for Recovery of Noble Metals. Minerals. 11 (11), 1188. doi:10.3390/min11111188
Han, K. N., Kellar, J. J., Cross, W. M., and Safarzadeh, S. (2014). Opportunities and Challenges for Treating Rare-Earth Elements. Geosystem Eng. 17 (3), 178–194. doi:10.1080/12269328.2014.958618
Hano, T., Matsumoto, M., Ohtake, T., Egashir, N., and Hori, F. (1992). Recovery of Lithium from Geothermal Water by Solvent Extraction Technique. Solvent Extraction and Ion Exchange 10 (2), 195–206. doi:10.1080/07366299208918100
Harvianto, G. R., Kim, S.-H., and Ju, C.-S. (2016). Solvent Extraction and Stripping of Lithium Ion from Aqueous Solution and its Application to Seawater. Rare Met. 35 (12), 948–953. doi:10.1007/s12598-015-0453-1
He, L., Xu, W., Song, Y., Luo, Y., Liu, X., and Zhao, Z. (2018). New Insights into the Application of Lithium-Ion Battery Materials: Selective Extraction of Lithium from Brines via a Rocking-Chair Lithium-Ion Battery System. Glob. Challenges 2 (2), 1700079. doi:10.1002/gch2.201700079
Hofmann, H., Babadagli, T., and Zimmermann, G. (2014). Numerical Simulation of Complex Fracture Network Development by Hydraulic Fracturing in Naturally Fractured Ultratight Formations. J. Energ. Resour. Technol 136 (4), 042905. doi:10.1115/1.4028690
Hofmann, H., Zimmermann, G., Farkas, M., Huenges, E., Zang, A., Leonhardt, M., et al. (2019). First Field Application of Cyclic Soft Stimulation at the Pohang Enhanced Geothermal System Site in Korea. Geophys. J. Int. 217 (3), 926–949. doi:10.1093/gji/ggz058
Jafari, H., Abdollahi, H., Gharabaghi, M., and Balesini, A. A. (2018). Solvent Extraction of Zinc from Synthetic Zn-Cd-Mn Chloride Solution Using D2EHPA: Optimization and Thermodynamic Studies. Separat. Purif. Tech. 197, 210–219. doi:10.1016/j.seppur.2018.01.020
Jang, E. (2016). Application of Solvent Extraction Method for Lithium Recovery from Shale Gas-Produced Water. MS Thesis. Seoul, South Korea: Seoul National University, 52–55.
Jang, E., Jang, Y., and Chung, E. (2017). Lithium Recovery from Shale Gas Produced Water Using Solvent Extraction. Appl. Geochem. 78, 343–350. doi:10.1016/j.apgeochem.2017.01.016
Jang, Y., and Chung, E. (2018). Adsorption of Lithium from Shale Gas Produced Water Using Titanium Based Adsorbent. Ind. Eng. Chem. Res. 57, 8381–8387. doi:10.1021/acs.iecr.8b00805
Jang, Y., and Chung, E. (2019). Lithium Adsorptive Properties of H2TiO3 Adsorbent from Shale Gas Produced Water Containing Organic Compounds. Chemosphere 221, 75–80. doi:10.1016/j.chemosphere.2019.01.032
Jin, Y., Ma, Y., Weng, Y., Jia, X., and Li, J. (2014). Solvent Extraction of Fe3+ from the Hydrochloric Acid Route Phosphoric Acid by D2EHPA in Kerosene. J. Ind. Eng. Chem. 20 (5), 3446–3452. doi:10.1016/j.jiec.2013.12.033
Joo, H., Lee, J., and Yoon, J. (2020). Short Review: Timeline of the Electrochemical Lithium Recovery System Using the Spinel LiMn2O4 as a Positive Electrode. Energies 13, 6235. doi:10.3390/en13236235
Kanoh, H., Ooi, K., Miyai, Y., and Katoh, S. (1993). Electrochemical Recovery of Lithium Ions in the Aqueous Phase. Sep. Sci. Technol. 28 (1-3), 643–651. doi:10.1080/01496399308019512
Kim, K.-I., Min, K.-B., Kim, K.-Y., Choi, J. W., Yoon, K.-S., Yoon, W. S., et al. (2018). Protocol for Induced Microseismicity in the First Enhanced Geothermal Systems Project in Pohang, Korea. Renew. Sustain. Energ. Rev. 91, 1182–1191. doi:10.1016/j.rser.2018.04.062
Kim, N., Su, X., and Kim, C. (2021). Electrochemical Lithium Recovery System through the Simultaneous Lithium Enrichment via Sustainable Redox Reaction. Chem. Eng. J. 420 (2), 127715. doi:10.1016/j.cej.2020.127715
Kislik, V., and Eyal, A. (2003). Competitive Complexation/solvation Theory of Solvent Extraction: General Statements, Acid Extraction by Amines, Influence of Active Solvents and Temperaturefluence of Active Solvents and Temperature. J. Chem. Technol. Biotechnol. 78, 358–363. doi:10.1002/jctb.786
Kislik, V., Eyal, A., and Hazan, B. (2003). Competitive Complexation/Solvation Theory of Solvent Extraction. III. Influence of Active Solvents on Acid Solvent Extraction by Amine Based Extractants. Separat. Sci. Tech. 38 (8), 1681–1704. doi:10.1081/ss-120019403
Kumar, P., Pournara, A., Kim, K.-H., Bansal, V., Rapti, S., and Manos, M. J. (2017). Metal-organic Frameworks: Challenges and Opportunities for Ion-Exchange/sorption Applications. Prog. Mater. Sci. 86, 25–74. doi:10.1016/j.pmatsci.2017.01.002
Lee, J., and Chung, E. (2020a). Application of Geochemical Modelling for Hydraulic Stimulation in Enhanced Geothermal Systems. Geosystem Eng. 23 (6), 342–350. doi:10.1080/12269328.2020.1832923
Lee, J., and Chung, E. (2021). Geochemical Effect of Geothermal Water Flushing during Enhanced Geothermal Systems Operation. ksmer 58 (3), 205–214. doi:10.32390/ksmer.2021.58.3.205
Lee, J., and Chung, E. (2020b). Lithium Recovery by Solvent Extraction from Simulated Shale Gas Produced Water - Impact of Organic Compounds. Appl. Geochem. 116 (6), 104571. doi:10.1016/j.apgeochem.2020.104571
Lee, P.-C., Li, C.-W., Chen, J.-Y., Li, Y.-S., and Chen, S.-S. (2011). Dissolution of D2EHPA in Liquid-Liquid Extraction Process: Implication on Metal Removal and Organic Content of the Treated Water. Water Res. 45, 5953–5958. doi:10.1016/j.watres.2011.08.054
Lei, Z., Zhang, Y., Yu, Z., Hu, Z., Li, L., Zhang, S., et al. (2019). Exploratory Research into the Enhanced Geothermal System Power Generation Project: The Qiabuqia Geothermal Field, Northwest China. Renew. Energ. 139, 52–70. doi:10.1016/j.renene.2019.01.088
Li, L., Deshmane, V. G., Paranthaman, M. P., Bhave, R., Moyer, B. A., and Harrison, S. (2018). Lithium Recovery from Aqueous Resources and Batteries: a Brief Review. Johnson Matthey Tech. Rev. 62 (2), 161–176. doi:10.1595/205651317x696676
Lu, S.-M. (2018). A Global Review of Enhanced Geothermal System (EGS). Renew. Sustain. Energ. Rev. 81 (2), 2902–2921. doi:10.1016/j.rser.2017.06.097
Masmoudi, A., Zante, G., Trébouet, D., Barillon, R., and Boltoeva, M. (2021). Solvent Extraction of Lithium Ions Using Benzoyltrifluoroacetone in New Solvents. Separat. Purif. Tech. 255, 117653. doi:10.1016/j.seppur.2020.117653
Nishihama, S., Onishi, K., and Yoshizuka, K. (2011). Selective Recovery Process of Lithium from Seawater Using Integrated Ion Exchange Methods. Solvent Extraction and Ion Exchange 29 (3), 421–431. doi:10.1080/07366299.2011.573435
Olasolo, P., Juárez, M. C., Morales, M. P., D´Amico, S., and Liarte, I. A. (2016). Enhanced Geothermal Systems (EGS): A Review. Renew. Sustain. Energ. Rev. 56, 133–144. doi:10.1016/j.rser.2015.11.031
Owen, J., Bustin, R. M., and Bustin, A. M. M. (2020). Insights from Mixing Calculations and Geochemical Modeling of Montney Formation post Hydraulic Fracturing Flowback Water Chemistry. J. Pet. Sci. Eng. 195, 107589. doi:10.1016/j.petrol.2020.107589
Park, Y.-M., Yeon, K.-M., and Park, C.-h. (2020). Silica Treatment Technologies in Reverse Osmosis for Industrial Desalination: A Review. Environ. Eng. Res. 25 (6), 819–829. doi:10.4491/eer.2019.353
Pauwels, H., Fouillac, C., and Criaud, A. (1992). Water-rock Interactions during Experiments within the Geothermal Hot Dry Rock Borehole GPK1, Soultz-Sous-Foreˆts, Alsace, France. Appl. Geochem. 7 (3), 243–255. doi:10.1016/0883-2927(92)90040-a
Putera, A. D. P., Wiranda, A., Mergiana, S., Perdana, I., and Olvianas, M. (2018). Assessing Silica Precipitation Using Calcium Hydroxide Addition on Dieng’s Geothermal Brine. IOP Conf. Ser. Earth Environ. Sci. 200, 012022. doi:10.1088/1755-1315/200/1/012022
Sadakane, A., Iwachido, T., and Tôei, K. (1975). The Extraction of Alkali Metal Picrates with dibenzo-18-crown-6. Bcsj 48, 60–63. doi:10.1246/bcsj.48.60
Sanjuan, B., Millot, R., Dezayes, C., and Brach, M. (2010). Main Characteristics of the Deep Geothermal Brine (5km) at Soultz-Sous-Forêts (France) Determined Using Geochemical and Tracer Test Data. C. R. - Geosci. 342 (7–8), 546–559. doi:10.1016/j.crte.2009.10.009
Sanjuan, B., Pinault, J. L., Rose, P., Gerard, A., Brach, M., Braibant, G., et al. (2006). “Geochemical Fluid Characteristics and Main Achievements about Tracer Tests at Soultz-sous-Forêts(France),” in EHDRA Scientific Conference, Soultz-sous-Forêts, France, June 15–16, 2006. 13. hal-00773150.
Scheiber, J., Nitschke, F., Seibt, A., and Genter, A. (2012). “Geochemical and Mineralogical Monitoring of the Geothermal Power Plant in Soultz-Sous-Forets (France),” in Proceedings of the 37th Workshop on Geothermal Reservoir Engineering, Stanford University, Stanford, CA, January 30–February 1, 2012, 1033–1042.
Schill, E., Genter, A., Cuenot, N., and Kohl, T. (2017). Hydraulic Performance History at the Soultz EGS Reservoirs from Stimulation and Long-Term Circulation Tests. Geothermics 70, 110–124. doi:10.1016/j.geothermics.2017.06.003
Seyed Alizadeh Ganji, S. M., Shafaei, S. Z., Goudarzi, N., and Azizi, A. (2016). Investigating the Best Mixture Extraction Systems in the Separation of Rare Earth Elements from Nitric Acid Solution Using Cyanex272, D2EHPA, and 8-Hydroxyquinoline. Geosystem Eng. 19 (1), 32–38. doi:10.1080/12269328.2015.1084243
Silva, J. E., Paiva, A. P., Soares, D., Labrincha, A., and Castro, F. (2005). Solvent Extraction Applied to the Recovery of Heavy Metals from Galvanic Sludge. J. Hazard. Mater. 120, 113–118. doi:10.1016/j.jhazmat.2004.12.008
Spitzmüller, L., Goldberg, V., Held, S., Grimmer, J. C., Winter, D., Genovese, M., et al. (2021). Selective Silica Removal in Geothermal Fluids: Implications for Applications for Geothermal Power Plant Operation and mineral Extraction. Geothermics 95, 102141. doi:10.1016/j.geothermics.2021.102141
Swain, B. (2017). Recovery and Recycling of Lithium: a Review. Separat. Purif. Tech. 172, 388–403. doi:10.1016/j.seppur.2016.08.031
Tanaka, M., and Akaiwa, H. (2009). Solvent Extraction Chemistry. (Choi, Y. Trans) Free Academy. 2nd edition.
Umetani, S., Maeda, K., Kihara, S., and Matsui, M. (1987). Solvent Extraction of Lithium and Sodium with 4-benzoyl or 4-Perfluoroacyl-5-Pyrazolone and TOPO. Talanta 34, 779–782. doi:10.1016/0039-9140(87)80095-4
U.S. Geological Survey (2018). Mineral Commodity Summaries 2018. Reston, VA: U.S. Geological Survey.
Vieceli, N., Reinhardt, N., Ekberg, C., and Petranikova, M. (2021). Optimization of Manganese Recovery from a Solution Based on Lithium-Ion Batteries by Solvent Extraction with D2EHPA. Metals 11, 54. doi:10.3390/met11010054
Wang, L., Frisella, K., Srimuk, P., Janka, O., Kickelbick, G., and Presser, V. (2021). Electrochemical Lithium Recovery with Lithium Iron Phosphate: what Causes Performance Degradation and How Can We Improve the Stability? Sustain. Energ. Fuels 5, 3124–3133. doi:10.1039/d1se00450f
Wang, S., Li, P., Zhang, X., Zheng, S., and Zhang, Y. (2017). Selective Adsorption of Lithium from High Mg-Containing Brines Using H X TiO 3 Ion Sieve. Hydrometallurgy 174, 21–28. doi:10.1016/j.hydromet.2017.09.009
Warren, I. (2021). Techno-Economic Analysis of Lithium Extraction from Geothermal Brines. NREL/TP-5700–79178. Golden, CO: National Renewable Energy Laboratory.
Xu, C., Dai, Q., Gaines, L., Hu, M., Tukker, A., and Steubing, B. (2020). Future Material Demand for Automotive Lithium-Based Batteries. Commun. Mater. 1, 99. doi:10.1038/s43246-020-00095-x
Xu, W., Liu, D., He, L., and Zhao, Z. (2020). A Comprehensive Membrane Process for Preparing Lithium Carbonate from High Mg/Li Brine. Membranes 10, 371. doi:10.3390/membranes10120371
Yang, W. F., Yuan, S., Xu, Y., Xiao, Y., Fang, K. M., and Xong, B. (2003). Extraction of Thorium Traces from Hydrochloric Acid media by 1-Phenyl-3-Methyl-4-Benzoyl- Pyrazolone-5. J. Radioanal. Nucl. Chem. 256, 149–152. doi:10.1023/A:1023324714875
Keywords: enhanced geothermal systems, geothermal fluid, lithium recovery, solvent extraction, silicates
Citation: Lee J and Chung E (2022) The Effect of Silicate Ions on the Separation of Lithium From Geothermal Fluid. Front. Chem. Eng. 4:741281. doi: 10.3389/fceng.2022.741281
Received: 14 July 2021; Accepted: 17 February 2022;
Published: 09 March 2022.
Edited by:
Patricia Taboada-Serrano, Rochester Institute of Technology, United StatesReviewed by:
Christiaan Richter, University of Iceland, IcelandJ. Paul Chen, National University of Singapore, Singapore
Copyright © 2022 Lee and Chung. This is an open-access article distributed under the terms of the Creative Commons Attribution License (CC BY). The use, distribution or reproduction in other forums is permitted, provided the original author(s) and the copyright owner(s) are credited and that the original publication in this journal is cited, in accordance with accepted academic practice. No use, distribution or reproduction is permitted which does not comply with these terms.
*Correspondence: Eunhyea Chung, ZWNodW5nQHNudS5hYy5rcg==