- 1Electrical and Computer Engineering Program, Computer, Electrical and Mathematical Sciences and Engineering Division, King Abdullah University of Science and Technology (KAUST), Thuwal, Saudi Arabia
- 2Exploration and Petroleum Engineering Center-Advanced Research Center (EXPEC ARC), Saudi Aramco, Dhahran, Saudi Arabia
Hydrogen is considered one of the most promising decarbonized fuels. However, its applicability is limited due to the ecological constraints of its production. Hydrogen sulfide (H2S) is widely available in oil and gas reservoirs and has the potential of becoming an energetically favorable source of hydrogen. Nevertheless, its electrochemical separation into H2 and elemental sulfur has not been successfully achieved at the industrial scale, due to sulfur poisoning of the electrodes at the sulfur oxidation half-reaction. This review highlights the progress of the direct electrolytic separation of H2S below the sulfur dew point, where the sulfur poisoning effect becomes more prominent. The article discusses the different technologies and approaches explored to improve the energy efficiency and stability of H2S electrolytic systems, including the recent use of nanostructured electrodes and novel sulfur solvents as electrolytes.
1 Introduction
Current environmental conditions call for the development of ecological energy sources (Sekimoto et al., 2015; Raziq et al., 2022a; Ali et al., 2022b; Raziq et al., 2022b; Ali et al., 2022c; Ali et al., 2022d; Iqbal et al., 2022; Wahid et al., 2023). Among the candidates, H2 is one of the most attractive alternatives to fossil fuels. Its direct combustion or fuel cells oxidation release no pollutant byproducts. However, the industrial production of H2 is mainly carried out by energy-intensive hydrocarbon reformation process (Kalamaras and Efstathiou, 2013). This aspect overshadows the ecological qualities of H2 and hinders its usage as a standard fuel. Research towards finding scalable, efficient, and environmentally friendly methods to produce H2 has been relentless, focusing primarily on catalytic water splitting (Ahmad et al., 2015; Chen et al., 2016; Shiva Kumar and Himabindu, 2019; Iqbal et al., 2021; Ali et al., 2022a; Yasin et al., 2022a; Yasin et al., 2022b; Ibraheem et al., 2022), due to its worldwide availability. Currently, the most economically and environmentally viable option to drive this reaction is via electrolysis. H2 generation systems based on this method are already commercially available. Nevertheless, water electrolysis, a longstanding known phenomenon (Trasatti, 1999), still has constraints such as high-cost electrodes, electrolytes, and catalysts, as well as long-term stability issues (Li et al., 2016).
An alternative source of H2 is hydrogen sulfide, although it is conventionally overlooked. Gaseous H2S is recognized for its characteristic rotten egg odor and its health hazards at concentrations as low as 10 ppm (Li et al., 2022). H2S is found concurrently in gas and oil reservoirs. It is conventionally considered a burden in processing fossil fuels, as it requires strict handling safety measures and costly remedial treatments. H2S is helpful in diverse industrial applications, nevertheless it is primarily oxidized in the Claus process (Zhang et al., 2015). Its byproducts are mostly sulfur and water, as indicated by the overall reaction found in Eq. 1.
An unwanted byproduct of the Claus process is SOx. Its emission is reduced to acceptable standards using tail-gas treatments, which achieve more than 99% sulfur recovery (de Crisci et al., 2019). Sulfur is the only valuable byproduct of this process, although it is not a high value commodity. Therefore, the Claus process is considered a convenient H2S mitigation method rather than a sulfur production process. In this regard, finding cost-effective and scalable methods to generate H2 from H2S is essential to unlocking the ecological and economic benefits of H2. Furthermore, such a process would bring value to the oil and gas industry.
The different explored approaches to achieve efficient and stable H2S splitting include high-temperature catalysis, thermochemical methods (Bandermann and Harder, 1982; Noring and Fletcher, 1982), photolysis, photocatalysis (Oladipo et al., 2021), plasma (Nunnally et al., 2009), and electrolysis. However, several challenges have hindered the development of a practical and economically viable splitting method. In the case of heterogeneous catalysis, the most common obstacle is the passivation of the electrocatalyst by elemental sulfur. This phenomenon has forced the development of specialized techniques to inhibit it, e. g., stirring the electrolyte, and using porous electrodes, organic solvents, and high operational temperatures (Jangam et al., 2021). The last technique is common in current Claus H2S treatment units. They are operated above the dew temperature of sulfur (180°C) to avoid precipitation (Li et al., 2022). However, its implementation becomes more delicate when the purpose of the reaction is to obtain H2 (Lim and Winnick, 1984; Weaver and Winnick, 1987). Additionally, operating at sustained high temperatures requires a continuous heat supply, which increases the reactor’s operational cost and promotes the components’ degradation. Thus, low-temperature dissociation methods would be preferable from an economic and environmental perspective. This review intends to highlight, from a technological and chronological perspective, the progress towards the decomposition of H2S into H2 and elemental sulfur by direct electrolysis at temperatures below the dew point of sulfur. The electrochemical conditions of the reactions, the electrocatalyst materials, and the available techniques to diminish the electrocatalyst passivation by sulfur precipitation are discussed, including novel techniques involving nanostructured electrodes and specialized sulfur solvents.
2 Electrochemical separation of H2S
Low-temperature electrochemical methods have gained interest in splitting H2S due to its scalability and promising overall energetic requirements. The former is due to the relatively simple electrochemical cell setup, especially when compared to complex photoelectrochemical methods. The latter attribute is a consequence of the minimal energy required for heating and relatively favorable thermodynamics of the H2S decomposition reaction. The potential difference between the H2 evolution reaction (HER) and sulfur oxidation reaction (SOR) is only 0.14 V (Kelsall and Thompson, 1993), substantially lower than the theoretical 1.23 V necessary to drive water splitting (Kay et al., 2006). However, the overall energy required to drive H2S splitting depends not only on its thermodynamics but also on the electrochemical setup, given that it is largely affected by the ohmic and mass transport losses as well as kinetic overpotentials on the electrocatalysts (Obata et al., 2019). Proper selection of the electrochemical conditions is a crucial step for optimizing the energy expenditure of the process.
In general, the electrolytic splitting of H2S is conducted via direct and indirect electrolysis. In direct electrolysis, H2S is oxidized straightforwardly into elemental sulfur and free protons, whereas indirect electrolysis is a two-step process. First, an intermediate is used to react chemically with H2S and precipitate or separate sulfur. Subsequently, the intermediate is regenerated by electrolytic oxidization. The advantage of the indirect electrolysis method is that the sulfur extraction is facilitated since the initial H2S reaction is decoupled from the electrolytic process. Nevertheless, this method requires a higher voltage to electrolyze the intermediate, and in some cases, the intermediate can degrade over time or migrate from the anodic to the cathodic chamber (Huang et al., 2019). On the other hand, the advantage of the direct electrolysis method is that it requires less energy because the potential necessary to drive the reaction is close to the HER and SOR potential difference. However, these systems suffer from sulfur passivation on the electrocatalyst surface or struggle to separate the sulfur from the electrolyte during the reaction. The sections below describe the advances in the field, and a summary of the mentioned works is found in Table 1.
2.1 Early developments on H2S splitting
One of the crucial steps in direct electrolysis of H2S at low temperatures is their absorption by a suitable electrolyte. This fluid should be chemically stable and thermodynamically inactive within the electrolysis potential difference. Early works demonstrated the possibility of using alkaline solutions for this purpose, particularly solutions containing NaOH (Anani et al., 1990; Mao et al., 1991). In those works, attention was shifted from the material composition of the SOR electrocatalyst, as their primary objective was to find the optimal H2S processing conditions. This involves splitting H2S with minimal sulfur deposition on the electrocatalysts at ∼80°C. Anani et al. (Anani et al., 1990) used graphite, nickel, nickel-chromium, and titanium as SOR electrodes. However, they did not present a meaningful comparison of their electrocatalytic activity as they only mentioned a worse passivation effect in the metallic electrodes. Indeed, at that time, the deactivation of electrocatalysts by sulfur precipitation during the electrolysis of polysulfide solutions was a well-known problem (Fetzer, 1928; Hodes et al., 1980; Buckley et al., 1987), and several attempts to palliate it are documented, including the use of organic solvents to remove it from the electrocatalyst (Shih and Lee, 1986).
Research towards optimizing the H2S splitting continued, looking for better SOR electrocatalysts and more practical methods to avoid their deactivation. Subsequent works expanded the list of SOR electrocatalytic materials, adding LaSrMnO3 and Raney-nickel. Petrov and Srinivasan (Petrov and Srinivasan, 1996) demonstrated that LaSrMnO3 had better SOR electrocatalytic performance than graphite, CoS, Raney-nickel, and Pt/C electrodes. They reached a current density of 300 mAcm−2 at a cell potential of only 1 V. Their process scrubbed H2S in a NaOH solution, then alkalinized the solution to pH 14, and electrolyzed it. All those steps were done in separate chambers, as Anani et al. (Anani et al., 1990) had previously proposed. Petrov and Srinivasan improved Anani et al.‘s method by doing the final sulfur precipitation in a separate chamber, preventing sulfur precipitation in the graphite, CoS, and LaSrMnO3 electrodes but not in the Raney-nickel, Ni, and Pt/C electrodes.
2.2 Sulfide electrooxidation mechanisms
Concomitantly, other researchers focused in understanding the reaction mechanism of sulfide electrooxidation. Cobalt phthalocyanine traces, used previously to catalyze the electroreduction of oxygen in KNO3 aqueous solutions (Komorsky-Lovrić, 1995), were studied as electrocatalysts for the oxidation of sulfide ions by Komorsky-Lovrić et al. (Komorsky-Lovrić et al., 1997) via cyclic voltammetry (CV). Under the chemical conditions of their experiments, the CV measurements were consistent with a two-step oxidation of HS− ions:
In the case of a low sulfide ion concentration (<10−4 M), the KHS2 adsorbed species would dissociate following the reaction shown in Eq. 4.
Komorsky-Lovrić et al. (1997) stated that the adsorbed sulfur stays on the surface of the electrocatalyst and works as a bridge for the charge transfer process between the electrode and HS− ions. However, it is essential to note that the proposed reaction mechanism does not yield free elemental sulfur as a final byproduct and that there were no analytical measurements of the species in the electrolyte after the redox reaction. Moreover, there was no description of gas evolution in electrodes, so it is unclear whether cobalt phthalocyanine-based SOR electrodes can perform the corresponding electrooxidation reaction to produce elemental sulfur.
Chen and Miller (Chen and Miller, 2004; Miller and Chen, 2005) performed further studies related to the mechanism of the electrochemical oxidation of sulfide ions. The SOR electrode used in their experiments was Ti/Ta2O5-IrO2, and they performed different electrochemical characterizations under different conditions, including variations in temperature and sulfide concentration in the aqueous electrolyte. In their experiments, temporal oscillations of the SOR electrode potential (vs. a reference electrode) were present in specific galvanostatic conditions and at specific current intervals during linear galvanic voltammograms. The analysis of their chronopotentiometry observations indicates that the origin of the oscillations was the alternation of the dominant oxidation reaction performed by the electrode. In the lower part of the potential oscillation, the dominant reaction is the oxidation of sulfide into sulfur, causing the sulfur deposition on the electrode and therefore inducing an increase of the electrode potential to keep the current at the set value. However, this increment in potential favors the oxygen evolution reaction (OER) from the aqueous electrolyte, making water oxidation the dominant reaction in the oscillation peak potential. The shift towards OER increases the availability of HS− and S2- ions as sulfur oxidation is minimized, increasing the dissolution of sulfur through the reactions displayed in Eq. 5 and Eq. 6.
The enhanced sulfur solubility and the increased mass transfer rate generated by the oxygen evolution led to the removal of sulfur deposits from the SOR electrode, promoting the rebound of the electrode potential at the lower bound of the oscillation. The continuous formation and removal of sulfur deposits on the surface of the electrode were observed during those measurements. This further supports the proposed reaction mechanism. These results highlight the importance of the selection of electrode materials, electrolytes, and electrical conditions of the electrolysis. The latter encompasses current density and potential, which influence the preferred chemical reactions. Such observation becomes even more relevant when there is great interest in applying these electrolytic processes at an industrial scale, where it is necessary to use high currents and voltages to obtain a higher yield of desired products.
2.3 H2S splitting beyond fossil fuels
Subsequent works explored the possibility of using electrolysis for H2S splitting in applications beyond the discussed cases of fossil fuel and natural gas scenarios. Sanli et al. (Sanli et al., 2014) researched the attainability of oxidizing sulfide ions contained in artificial seawater with chemical conditions like the ones found in the Black Sea. Their investigations used MoS2 as the SOR electrode, which exhibited electrocatalytic activity towards the oxidation of HS− into elemental sulfur. However, the final purpose of their work was to use the SOR electrode in H2S fuel cells, where the catalytic HS− oxidation must occur spontaneously to generate electricity besides elemental sulfur. The idea of oxidizing sulfide from brines was also explored by Ateya et al. (Ateya et al., 2005). They used carbon-felt SOR electrodes to drive H2S splitting from wastewater brines. Alternatively, Karapekmez and Dincer (Karapekmez and Dincer, 2018) presented an H2S abatement system using a proton exchange membrane electrolyzer (PEME). The H2S PEME had a CsHSO4 electrolyte and operated at 150°C. Their system was designed to treat the H2S generated in geothermal power plants, and its practical implementation led to a substantial decrease in H2S emissions.
2.4 Avoiding the passivation of SOR electrodes
The passivation of SOR electrodes had been the bottleneck in the development of low-temperature H2S splitting systems. Recently, two research approaches, shown schematically in Figure 1, demonstrated the possibility of avoiding the passivation of SOR electrodes, one through the design of nanostructured electrocatalysts and the other using a novel organic sulfur solvent. In the case of nanostructured electrodes, Zhang et al. (Zhang et al., 2020) reported the fabrication of a SOR electrode consisting of CoNi nanoparticles encapsulated in graphene shells and supported in Ni foam. Three-electrode measurements showed that using this electrode, the onset potential of sulfide oxidation was only ∼0.25 V vs. reversible hydrogen electrode (RHE), 1.24 V lower than the onset potential of water oxidation. Additionally, the electrode exhibited a stable performance during a 500 h chronoamperometry test using a Na2S/NaOH aqueous solution with a current density of around 30 mAcm−2. These results showcase the possibility of generating H2 reliably, with a Faradaic efficiency of about 98% and using significantly less energy than water splitting. The chronopotentiometry analysis of the nanostructured electrode in more realistic conditions was also reported, where it was tested for 1,200 h in a 1 M NaOH solution saturated with 2% H2S/Syngas (50% CO and 50% H2) at a current density of 20 mAcm−2. In such conditions, the electrode’s potential was maintained at around 0.5 V vs. RHE with minimal variations. Density functional theory calculations showed that the free adsorption energy of the sulfide oxidation intermediate is minimized in graphene-encapsulated CoNi nanoparticles compared with pure graphene or CoNi nanoparticles alone. This characteristic is generally accepted as an indicator of good catalytic material (Nørskov et al., 2009), thus explaining the excellent activity of the electrode towards the SOR and its capacity to avoid sulfur deposition on its surface.
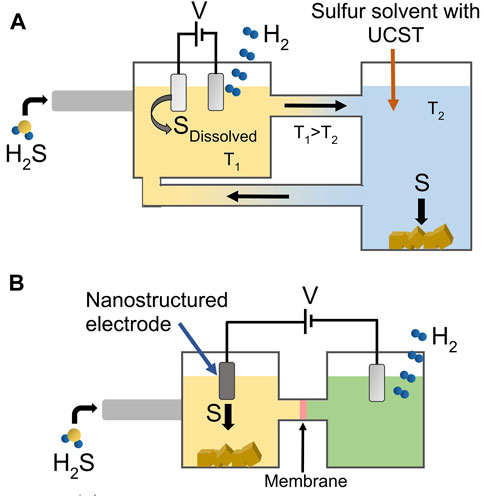
FIGURE 1. Current approaches to solve the SOR electrode passivation by sulfur deposition by using either (A) a sulfur solvent with a upper critical solution temperature (UCST), where sulfur solubility drastically depends on the solvent temperature; and (B) a nanostructured electrode with high sulfur-deposition resistance.
Nanostructured electrodes exhibited further advantages in the work led by Kumar and Nagaiah (Kumar and Nagaiah, 2022). They used CoFeS2 nanograins conjugated with nitrogen-containing carbon as a SOR electrode. They tested several stoichiometries of the sulfide material, finding a better electrocatalytic activity towards the SOR using an electrode with a Co:Fe ratio of 3:1. Linear sweep voltammograms of this electrode showed a SOR onset potential of just 0.23 V vs. RHE. The current of the electrode in chronopotentiometry measurements for 120 h was about 55 mAcm−2 at only 0.3 V vs. RHE, a better performance than the nanostructured SOR electrode from the work of Zhang et al. (Zhang et al., 2020) with a comparable Faradaic efficiency for H2 generation (∼97%). The electrode’s current during the 120-h characterization was relatively stable, with a slight decrease over time due to a reduction of the electrochemically active area to 81% of its initial value, demonstrating a minor passivation effect suffered by the electrode.
Ma et al. (2020) proposed another approach to suppress the passivation of the SOR electrode. They electrolyzed H2S in a mixture of an ionic liquid (IL) [C3OHmim]- BF4, tetraethylene glycol dimethyl ether (TGDE), and monoethanolamide (MEA). The IL, TGDE, and MEA mixture exhibited a superior sulfur solubility above 20°C when compared with a mixture of IL and TGDE. This is ascribed to the protonation of MEA by H2S, as indicated in Eq. 7.
They took advantage of the gradient of the sulfur solubility as a function of temperature to propose a cyclic electrolytic process. A Pt microdisk was used as a SOR electrode and the temperature was modified at different stages of the cycle. During H2S electrolysis, the temperature of the electrolyte is kept at 50°C. As the electrolysis process continues, the electrolyte’s color shifts from transparent to yellow, indicating the presence of elemental sulfur dissolved in the electrolyte. The electrolysis is stopped, and the electrode is removed before the electrolyte saturates with dissolved sulfur to avoid its precipitation on the surface of the Pt microdisk. Next, the electrolyte is cooled down to room temperature, which decreases its sulfur solubility, enhancing the precipitation of sulfur. Then the solid sulfur is separated from the electrolyte, which can be used to absorb H2S again and start a new electrolytic cycle. The electrode’s endurance test consisted of three 7-h electrolytic cycles with a current density in the range of 10–13 mAcm−2. The Faraday efficiency of the H2 evolution presented a slight decrease from 90.5% to 89.3% from the first to the third electrolytic cycles. These relatively low values were attributed to the formation of polysulfides. The “shuttling mechanism,” could explain the large overpotential (∼1.63 V at ∼10.5 mAcm−2) in comparison to the nanostructured electrode proposed by Zhang et al. (0.4 V at ∼30 mAcm−2) (Zhang et al., 2020). The report does not discuss the origin of the large overpotential or if it originates in the oxidation or reduction side. However, the cyclic voltammograms suggest that the electrocatalytic activity of the Pt microdisk is larger when using the electrolyte containing MEA. The origin of the overpotential is likely related to the slower kinetics of this amine, for example, in the reduction of the protonated MEA species formed by the reaction shown in Eq. 7.
3 Conclusion and perspectives
The direct electrolysis of H2S into H2 and elemental sulfur has proven to be a laborious task. The challenge of driving the reaction at low temperatures adds a new dimension to this conversion pathway. However, the research done so far in this field has brought several promising achievements, particularly regarding the avoidance of the passivation of SOR electrodes by using novel nanostructured electrodes or novel sulfur solvents. At this point, there is no feasible method that can be taken to an industrial scale, and there is room for improvement and research on low-temperature H2S splitting systems. For example, it is cumbersome to evaluate the actual capability of a SOR electrode when its electrocatalytic activity is immediately blocked by sulfur deposition. Thus, with the development of novel sulfur solvents, it might be worth revisiting the performance of the SOR electrode materials studied so far in conditions where they are not affected by sulfur passivation. From Table 1, the variety of materials used as SOR electrocatalysts is not as diverse as it might be expected after almost a century of research. Possibly the electrode passivation and H2S toxicity discouraged researchers from dedicating their effort and resources to the topic. Further research may also include sustainable regeneration techniques, where the electrocatalysts can be subjected to chemical or thermal treatment to reactivate their catalytic sites (Ledoux et al., 2000).
Author contributions
MV-R and AC contributed to the discussion of the topic and the structuring of the manuscript. MV-R wrote the first draft of the manuscript and AC revised the final version. All the authors approved the submitted version of the manuscript.
Funding
This research was internally funded by Saudi Aramco. The funder was not involved in the study design, collection, analysis, interpretation of data, the writing of this article, or the decision to submit it for publication.
Conflict of interest
The authors declare that the research was conducted in the absence of any commercial or financial relationships that could be construed as a potential conflict of interest.
Publisher’s note
All claims expressed in this article are solely those of the authors and do not necessarily represent those of their affiliated organizations, or those of the publisher, the editors and the reviewers. Any product that may be evaluated in this article, or claim that may be made by its manufacturer, is not guaranteed or endorsed by the publisher.
Supplementary material
The Supplementary Material for this article can be found online at: https://www.frontiersin.org/articles/10.3389/fceng.2022.1087435/full#supplementary-material
References
Ahmad, H., Kamarudin, S. K., Minggu, L. J., and Kassim, M. (2015). Hydrogen from photo-catalytic water splitting process: A review. Renew. Sustain. Energy Rev. 43, 599–610. doi:10.1016/j.rser.2014.10.101
Ali, S., Ali, S., Ismail, P. M., Shen, H., Zada, A., Ali, A., et al. (2022a). Synthesis and bader analyzed cobalt-phthalocyanine modified solar UV-blind β-Ga2O3 quadrilateral nanorods photocatalysts for wide-visible-light driven H2 evolution. Appl. Catal. B 307, 121149. doi:10.1016/J.APCATB.2022.121149
Ali, S., Iqbal, R., Wahid, F., Ismail, P. M., Saleem, A., Ali, S., et al. (2022b). Cobalt coordinated two-dimensional covalent organic framework a sustainable and robust electrocatalyst for selective CO2 electrochemical conversion to formic acid. Fuel Process. Technol. 237, 107451. doi:10.1016/j.fuproc.2022.107451
Ali, S., Ismail, P. M., Wahid, F., Kumar, A., Haneef, M., Raziq, F., et al. (2022c). Benchmarking the two-dimensional conductive Y3(C6X6)2 (Y = Co, Cu, Pd, Pt; X = NH, NHS, S) metal-organic framework nanosheets for CO2 reduction reaction with tunable performance. Fuel Process. Technol. 236, 107427. doi:10.1016/j.fuproc.2022.107427
Ali, S., Yasin, G., Iqbal, R., Huang, X., Su, J., Ibraheem, S., et al. (2022d). Porous aza-doped graphene-analogous 2D material a unique catalyst for CO2 conversion to formic-acid by hydrogenation and electroreduction approaches. Mol. Catal. 524, 112285. doi:10.1016/j.mcat.2022.112285
Anani, A. A., Mao, Z., White, R. E., Srinivasan, S., and Appleby, A. J. (1990). Electrochemical production of hydrogen and sulfur by low-temperature decomposition of hydrogen sulfide in an aqueous alkaline solution. J. Electrochem Soc. 137, 2703–2709. doi:10.1149/1.2087021
Ateya, B. G., Al-Kharafi, F. M., Abdallah, R. M., and Al-Azab, A. S. (2005). Electrochemical removal of hydrogen sulfide from polluted brines using porous flow through electrodes. J. Appl. Electrochem 35, 297–303. doi:10.1007/s10800-004-7273-6
Bandermann, F., and Harder, K. B. (1982). Production of H2 via thermal decomposition of H2S and separation of H2 and H2S by pressure swing adsorption. Int. J. Hydrogen Energy 7, 471–475. doi:10.1016/0360-3199(82)90103-3
Buckley, A. N., Hamilton, I. C., and Woods, R. (1987). An investigation of the sulphur(-II)/sulphur(0) system on bold electrodes. J. Electroanal. Chem. 216, 213–227. doi:10.1016/0022-0728(87)80208-5
Chen, A., and Miller, B. (2004). Potential oscillations during the electrocatalytic oxidation of sulfide on a microstructured Ti/Ta2O5-IrO2 electrode. J. Phys. Chem. B 108, 2245–2251. doi:10.1021/jp036639h
Chen, S., Thind, S. S., and Chen, A. (2016). Nanostructured materials for water splitting - state of the art and future needs: A mini-review. Electrochem Commun. 63, 10–17. doi:10.1016/j.elecom.2015.12.003
de Crisci, A. G., Moniri, A., and Xu, Y. (2019). Hydrogen from hydrogen sulfide: Towards a more sustainable hydrogen economy. Int. J. Hydrogen Energy 44, 1299–1327. doi:10.1016/j.ijhydene.2018.10.035
Fetzer, W. R. (1928). The electrolysis of sodium sulphide solutions. J. Phys. Chem. 32, 1787–1807. doi:10.1021/j150294a002
Hodes, G., Manassen, J., and Cahen, D. (1980). Electrocatalytic electrodes for the polysulfide redox system. J. Electrochem Soc. 127, 544–549. doi:10.1149/1.2129709
Huang, H., Shang, J., Yu, Y., and Chung, K. H. (2019). Recovery of hydrogen from hydrogen sulfide by indirect electrolysis process. Int. J. Hydrogen Energy 44, 5108–5113. doi:10.1016/j.ijhydene.2018.11.010
Ibraheem, S., Yasin, G., Kumar, A., Mushtaq, M. A., Ibrahim, S., Iqbal, R., et al. (2022). Iron-cation-coordinated cobalt-bridged-selenides nanorods for highly efficient photo/electrochemical water splitting. Appl. Catal. B 304, 120987. doi:10.1016/J.APCATB.2021.120987
Iqbal, R., Akbar, M. B., Ahmad, A., Hussain, A., Altaf, N., Ibraheem, S., et al. (2022). Exploring the synergistic effect of novel Ni-Fe in 2D bimetallic metal-organic frameworks for enhanced electrochemical reduction of CO2. Adv. Mater Interfaces 9, 2101505. doi:10.1002/admi.202101505
Iqbal, R., Yasin, G., Hamza, M., Ibraheem, S., Ullah, B., Saleem, A., et al. (2021). State of the art two-dimensional covalent organic frameworks: Prospects from rational design and reactions to applications for advanced energy storage technologies. Coord. Chem. Rev. 447, 214152. doi:10.1016/J.CCR.2021.214152
Jangam, K., Chen, Y. Y., Qin, L., and Fan, L. S. (2021). Perspectives on reactive separation and removal of hydrogen sulfide. Chem. Eng. Sci. X 11, 100105. doi:10.1016/j.cesx.2021.100105
Kalamaras, C. M., and Efstathiou, A. M. (2013). Hydrogen production technologies: Current state and future developments. Conf. Pap. Energy 2013, 1–9. doi:10.1155/2013/690627
Karapekmez, A., and Dincer, I. (2018). Modelling of hydrogen production from hydrogen sulfide in geothermal power plants. Int. J. Hydrogen Energy 43, 10569–10579. doi:10.1016/j.ijhydene.2018.02.020
Kay, A., Cesar, I., and Grätzel, M. (2006). New benchmark for water photooxidation by nanostructured α-Fe 2O3 films. J. Am. Chem. Soc. 128, 15714–15721. doi:10.1021/ja064380l
Kelsall, G. H., and Thompson, I. (1993). Redox chemistry of H2S oxidation by the British gas Stretford process part V: Aspects of the process chemistry. J. Appl. Electrochem 23, 427–434. doi:10.1007/BF00707618
Komorsky-Lovrić, Š. (1995). Voltammetry of microcrystals of cobalt and manganese phthalocyanines. J. Electroanal. Chem. 397, 211–215. doi:10.1016/0022-0728(95)04194-5
Komorsky-Lovrić, Š., Lovrić, M., and Scholz, F. (1997). Sulfide ion electrooxidation catalysed by cobalt phthalocyanine microcrystals. Mikrochim. Acta 127, 95–99. doi:10.1007/bf01243171
Kumar, M., and Nagaiah, T. C. (2022). Efficient production of hydrogen from H2S via electrolysis using a CoFeS2 catalyst. J. Mater Chem. A Mater 10, 7048–7057. doi:10.1039/d1ta09888h
Ledoux, M. J., Pham-Huu, C., Keller, N., Nougayrède, J. B., Savin-Poncet, S., and Bousquet, J. (2000). Selective oxidation of H2S in Claus tail-gas over SiC supported NiS2 catalyst. Catal. Today 61, 157–163. doi:10.1016/S0920-5861(00)00365-5
Li, F., Laaksonen, A., Zhang, X., and Ji, X. (2022). Rotten eggs revaluated: Ionic liquids and deep eutectic solvents for removal and utilization of hydrogen sulfide. Ind. Eng. Chem. Res. 61, 2643–2671. doi:10.1021/acs.iecr.1c04142
Li, X., Hao, X., Abudula, A., and Guan, G. (2016). Nanostructured catalysts for electrochemical water splitting: Current state and prospects. J. Mater Chem. A Mater 4, 11973–12000. doi:10.1039/c6ta02334g
Lim, H. S., and Winnick, J. (1984). Electrochemical removal and concentration of hydrogen sulfide from coal gas. J. Electrochem Soc. 131, 562–568. doi:10.1149/1.2115627
Ma, Y., Jin, X., Hu, Y., Huang, Q., and Wang, Z. (2020). Recovery of hydrogen and sulfur by electrolysis of ionized H2S in an amine-containing organic electrolyte with highly temperature-dependent sulfur solubility. Energy Fuels 34, 7756–7762. doi:10.1021/acs.energyfuels.0c01161
Mao, Z., Anani, A., White, R. E., Srinivasan, S., and Appleby, A. J. (1991). A modified electrochemical process for the decomposition of hydrogen sulfide in an aqueous alkaline solution. J. Electrochem Soc. 138, 1299–1303. doi:10.1149/1.2085775
Miller, B., and Chen, A. (2005). Effect of concentration and temperature on electrochemical oscillations during sulfide oxidation on Ti/Ta2O5-IrO2 electrodes. Electrochim Acta 50, 2203–2212. doi:10.1016/j.electacta.2004.10.002
Noring, J. E., and Fletcher, E. A. (1982). High temperature solar thermochemical processing-hydrogen and sulfur from hydrogen sulfide. Energy 7, 651–666. doi:10.1016/0360-5442(82)90002-0
Nørskov, J. K., Bligaard, T., Rossmeisl, J., and Christensen, C. H. (2009). Towards the computational design of solid catalysts. Nat. Chem. 1, 37–46. doi:10.1038/nchem.121
Nunnally, T., Gutsol, K., Rabinovich, A., Fridman, A., Starikovsky, A., Gutsol, A., et al. (2009). Dissociation of H2S in non-equilibrium gliding arc “tornado” discharge. Int. J. Hydrogen Energy 34, 7618–7625. doi:10.1016/j.ijhydene.2009.07.045
Obata, K., Shinohara, Y., Tanabe, S., Waki, I., Kotsovos, K., Ohkawa, K., et al. (2019). A stand-alone module for solar-driven H2 production coupled with redox-mediated sulfide remediation. Energy Technol. 7, 1900575. doi:10.1002/ente.201900575
Oladipo, H., Yusuf, A., al Jitan, S., and Palmisano, G. (2021). Overview and challenges of the photolytic and photocatalytic splitting of H2S. Catal. Today 380, 125–137. doi:10.1016/j.cattod.2021.03.021
Petrov, K., and Srinivasan, S. (1996). Low temperature removal of hydrogen sulfide from sour gas and its utilization for hydrogen and sulfur production. Int. J. Hydrogen Energy 21, 163–169. doi:10.1016/0360-3199(95)00003-8
Raziq, F., Aligayev, A., Shen, H., Ali, S., Shah, R., Ali, S., et al. (2022a). Exceptional photocatalytic activities of rGO modified (B, N) Co-doped WO3, coupled with CdSe QDs for one photon Z-scheme system: A joint experimental and dft study. Adv. Sci. 9, 2102530. doi:10.1002/advs.202102530
Raziq, F., Khan, K., Ali, S., Ali, S., Xu, H., Ali, I., et al. (2022b). Accelerating CO2 reduction on novel double perovskite oxide with sulfur, carbon incorporation: Synergistic electronic and chemical engineering. Chem. Eng. J. 446, 137161. doi:10.1016/j.cej.2022.137161
Sanli, A. E., Aytaç, A., and Mat, M. (2014). Investigation of the electro-oxidation of artificial Black Sea water by cyclic voltammetry on molybdenum (II). Int. J. Hydrogen Energy 39, 9221–9229. doi:10.1016/j.ijhydene.2014.03.047
Sekimoto, T., Shinagawa, S., Uetake, Y., Noda, K., Deguchi, M., Yotsuhashi, S., et al. (2015). Tandem photo-electrode of InGaN with two Si p-n junctions for CO2 conversion to HCOOH with the efficiency greater than biological photosynthesis. Appl. Phys. Lett. 106, 073902–073910. doi:10.1063/1.4910510
Shih, Y.-S., and Lee, J.-L. (1986). Continuous solvent extraction of sulfur from the electrochemical oxidation of a basic sulfide solution in the CSTER system. Industrial Eng. Chem. Process Des. Dev. 25, 834–836. doi:10.1021/i200034a041
Shiva Kumar, S., and Himabindu, V. (2019). Hydrogen production by PEM water electrolysis – a review. Mater Sci. Energy Technol. 2, 442–454. doi:10.1016/j.mset.2019.03.002
Trasatti, S. (1999). Water electrolysis: Who first? J. Electroanal. Chem. 476, 90–91. doi:10.1016/S0022-0728(99)00364-2
Wahid, F., Ali, S., Ismail, P. M., Raziq, F., Ali, S., Yi, J., et al. (2023). Metal single atom doped 2D materials for photocatalysis: Current status and future perspectives. Prog. Energy 5, 012001. doi:10.1088/2516-1083/ac9eff
Weaver, D., and Winnick, J. (1987). Electrochemical removal of H2S from hot gas streams: Nickel/Nickel-sulfide cathode performance. J. Electrochem Soc. 134, 2451–2458. doi:10.1149/1.2100220
Yasin, G., Ibraheem, S., Ali, S., Arif, M., Ibrahim, S., Iqbal, R., et al. (2022a). Defects-engineered tailoring of tri-doped interlinked metal-free bifunctional catalyst with lower gibbs free energy of OER/HER intermediates for overall water splitting. Mater Today Chem. 23, 100634. doi:10.1016/J.MTCHEM.2021.100634
Yasin, G., Ibrahim, S., Ajmal, S., Ibraheem, S., Ali, S., Nadda, A. K., et al. (2022b). Tailoring of electrocatalyst interactions at interfacial level to benchmark the oxygen reduction reaction. Coord. Chem. Rev. 469, 214669. doi:10.1016/J.CCR.2022.214669
Zhang, M., Guan, J., Tu, Y., Chen, S., Wang, Y., Wang, S., et al. (2020). Highly efficient H2 production from H2S: Via a robust graphene-encapsulated metal catalyst. Energy Environ. Sci. 13, 119–126. doi:10.1039/c9ee03231b
Keywords: hydrogen sulfide, electrolysis, H2 generation, sulfur generation, ionic liquids
Citation: Velazquez-Rizo M and Cavazos Sepulveda AC (2023) Low-temperature direct electrochemical splitting of H2S. Front. Chem. Eng. 4:1087435. doi: 10.3389/fceng.2022.1087435
Received: 02 November 2022; Accepted: 19 December 2022;
Published: 05 January 2023.
Edited by:
Ghulam Yasin, Beijing University of Chemical Technology, ChinaReviewed by:
Sajjad Ali, University of Electronic Science and Technology of China, ChinaSaira Ajmal, Shenzhen University, China
Copyright © 2023 Velazquez-Rizo and Cavazos Sepulveda. This is an open-access article distributed under the terms of the Creative Commons Attribution License (CC BY). The use, distribution or reproduction in other forums is permitted, provided the original author(s) and the copyright owner(s) are credited and that the original publication in this journal is cited, in accordance with accepted academic practice. No use, distribution or reproduction is permitted which does not comply with these terms.
*Correspondence: Adrian Cesar Cavazos Sepulveda, YWRyaWFuLmNhdmF6b3NzZXB1bHZlZGFAYXJhbWNvLmNvbQ==