- 1Department of Bioengineering, Northeastern University, Boston, MA, United States
- 2Department of Chemical Engineering, Northeastern University, Boston, MA, United States
Neural stem cells have attracted attention in recent years to treat neurodegeneration. There are two neurogenic regions in the brain where neural stem cells reside, one of which is called the subventricular zone (SVZ). The SVZ niche is a complicated microenvironment providing cues to regulate self-renewal and differentiation while maintaining the neural stem cell’s pool. Many scientists have spent years understanding the cellular and structural characteristics of the SVZ niche, both in homeostasis and pathological conditions. On the other hand, engineers focus primarily on designing platforms using the knowledge they acquire to understand the effect of individual factors on neural stem cell fate decisions. This review provides a general overview of what we know about the components of the SVZ niche, including the residing cells, extracellular matrix (ECM), growth factors, their interactions, and SVZ niche changes during aging and neurodegenerative diseases. Furthermore, an overview will be given on the biomaterials used to mimic neurogenic niche microenvironments and the design considerations applied to add bioactivity while meeting the structural requirements. Finally, it will discuss the potential gaps in mimicking the microenvironment.
1 Introduction
Until the second half of the 20th century, it was believed that the adult mammalian brain did not maintain active neurogenesis after embryonic development; however, it is now known that neurogenesis takes place continuously throughout life in the subventricular and the subgranular zones of the brain. In 1962, Joseph Altman was the first scientist to discover evidence of neurogenesis in the adult brain by injecting radioactively labeled thymidine to detect newly formed cells. His group found significant labeling of dentate gyrus granule cells in the hippocampus, suggesting a continuous formation of neurons (Altman, 1962). Later in the 1980s, Fernando Nottebohm provided the first incontrovertible evidence of adult neurogenesis by labeling newly formed cells in birds’ brains that were conducting electricity (Nottebohm, 1981). He also identified new neurons formed by the division of cells in the walls of the lateral ventricle (Nottebohm, 2004). All of these findings were not taken seriously until the early 1990s with the emergence of advanced technologies in imaging and methods to determine protein and gene expression. By the end of the 1990s, several studies showed continued neuronal differentiation in various mammals, including humans. In the same decade, the self-renewal capability and multipotency of neural stem cells derived from the adult mammalian brain were also indicated (Reynolds and Weiss, 1992). Since demonstrating that new neurons are formed throughout a lifetime, scientists have focused on understanding how new neurons are generated while engineers seek to design new models to better mimic the physiological and pathological neurogenic niche (Figure 1). Combining both points of views, researchers can develop therapies for brain injuries and neurodegenerative diseases and for rejuvenation of brain circuits that deteriorate with aging and diseases.
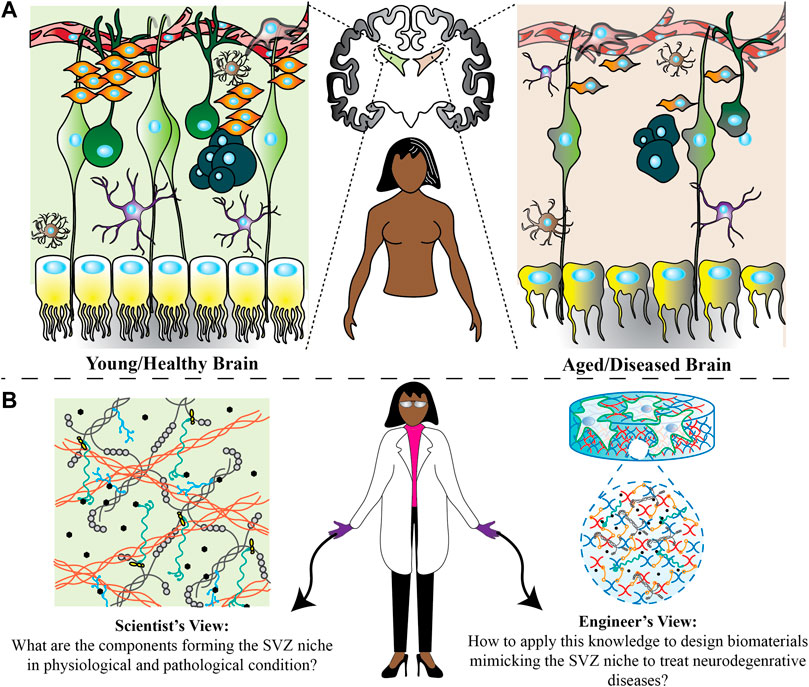
FIGURE 1. Possible changes happening in the SVZ region in physiological and pathological conditions. (A) Through pathological conditions, the shape of ependymal cells changes from cubical to more flattened with scattered cilia. Furthermore, residing astrocytes and microglia undergo functional and morphological changes. There are also fewer numbers of neural stem cells, progenitor cells and neuroblasts in an aged/diseased brain. All of these could be the potential reasons for brain function changes. (B) Scientists and engineers can develop new treatment ideas from two different perspectives. Scientists can find out how these changes affect the microenvironment homeostasis and engineers can use this information to design a microenvironment in vitro to understand how every single factor change can affect the neural stem cell fate decisions, and ultimately examine in vivo how factors can be used.
Within the field of regenerative medicine, neural stem cells have attracted increasing attention because of their potential for treating neurodegenerative diseases. They are present within two main regions of the mammalian brain from embryonic development to adulthood, the subgranular zone (SGZ) within the dentate gyrus (DG) of the hippocampus and the subventricular zone (SVZ) lining the lateral ventricles. Neural stem cells are tri-potent with the capacity to generate neurons, astrocytes, and oligodendrocytes in the central nervous system (CNS) and self-renew (Ma et al., 2009). Neural stem cells in SGZ can generate glutamatergic dentate granule neurons while the ones located in SVZ give rise to olfactory bulb interneurons and oligodendrocytes in the corpus callosum, fimbria fornix, and striatum (Jackson and Alvarez-Buylla, 2008; Capilla-Gonzalez., 2015). In the adult mammalian brain, SGZ and SVZ niches are unique in supporting neurogenesis, whereas other regions of the brain only support gliogenesis (Kjell et al., 2020); however, it is still controversial if neurogenesis in humans is similar to what is observed in rodents (Conover and Todd, 2017). In this regard, “neurogenesis” refers to sets of different events, including neural stem cell activation, proliferation, differentiation, and integration to neural circuits leading to the development of new neurons.
Regardless of the location, the niche where neural stem cells reside is a complex microenvironment that both supports the resident stem cell potency and helps to determine a cell’s fate (Bjornsson et al., 2015). To fully harness the power of neural stem cells, understanding the interactions between stem cells and their extracellular materials is crucial. The physical properties and bioactivity of the niche play a pivotal role in determining the regenerative capacity of neural stem cells. As engineers, we seek to investigate these material properties to be able to design new solutions to both regulate stem cell fate and provide new solutions for diseased or aged tissues. In this review paper, we first delve into more details about the neural stem cell’s niche properties with a focus on the SVZ niche and the properties that affect neural stem cell’s fate. We will then highlight some design considerations in biomaterial development to advance neural tissue engineering. Finally, we discuss the future direction in biomaterial development.
2 SVZ niche
During development, neural stem cells generate the whole nervous system (Zhao and Moore, 2018). They undergo symmetric division to extend the stem cell pool and asymmetric division to generate progenitors and differentiated cells. There are three main cell types, neurons, astrocytes and oligodendrocytes, all of which are generated from neural stem cells. However, through adulthood, a fewer number of neural stem cells survive and they are mostly quiescent with low metabolic rates and undergo self-renewal with a prolonged cell cycle under specific physiological circumstances (Wang et al., 2011). The balance between quiescence and activity of neural stem cells determines the neurogenesis and long-term maintenance of the stem cell pool while aging. Upon receiving physiological stimuli (e.g., damaged signals), quiescent stem cells respond by increasing the proliferation, differentiation, and migration of newborn neurons to lesion sites (Wang et al., 2011).
The stem cell microenvironment within the SVZ is complex, as shown in Figure 1. The SVZ region is adjacent to the lateral wall of the lateral ventricles of the adult mammalian brain and is one region that houses neural stem cells. In this region, neural stem cells are the most studied cells with the potential to differentiate toward glial cells and neurons. SVZ stem cells are classified in various states as type A, B, or C. Type B cells are radial glia-like neural stem cells that spread to contact both the cerebrospinal fluid in the ventricular space on the apical side while contacting the blood vessels with basal processes (Cutler and Kokovay, 2020). Type B cells are abundant cells within the niche and are generally quiescent. As Type B cells exit from the quiescence state and become reactivated to proliferate, they give rise to transient amplifying progenitors (Type C cells) (Ding et al., 2020). Type C cells then proliferate and generate neuroblasts, Type A cells, which migrate along blood vessels and proceed to differentiate within existing neuronal circuits (Lledo, et al., 2008) (Hsieh, 2012). However, most cells will either die or differentiate toward astrocytes or oligodendrocytes, resulting in a very limited number of new neurons integrating into neuronal circuits (Wang et al., 2011). Neuroblasts have also been found to migrate toward an injured area, guided by vasculature, to differentiate to mature neurons to facilitate neuronal repair (Fujioka et al., 2017).
As brain self-regeneration may not efficiently occur, exogenous stimuli are needed to promote its function. Thus, in the following sections, we highlight the recent insights into the various natural stimuli provided within the SVZ niche, including cell-cell interactions, soluble and insoluble ligands, and mechanical properties (Manabe et al., 2008; Hynes, 2009). We will then explain how researchers used this knowledge to develop a microenvironment in vitro to study the effect of each factor and to be able to direct the cell behavior to the success of the therapeutic of interest.
2.1 Cells within the SVZ
In the SVZ region, neural stem cells contact different cell types, including different progenies of neural stem cells, ependymal cells, microglia, astrocytes, and vascular cells, depending on the region (either vascular niche, intermediate SVZ niche, or cerebrospinal fluid/ependymal niche) as shown in Figure 2. Ependymal cells are multi-ciliated cells derived from radial glial cells, most of which persist throughout life, but their regeneration is limited (Merkle et al., 2004). They line the ventricular space forming a protective barrier. Their apical surfaces are covered with beating cilia that contribute to cerebrospinal fluid circulation around the CNS (Mahuzier et al., 2018). Interaction with this fluid flow and the key signaling molecules within the cerebrospinal fluid is necessary for brain homeostasis, neuroblast migration, and neurogenesis (Seo et al., 2021). Ependymal cells and neural stem cells are arranged into polarized structures called pinwheels, through the center of which a single apical process of a stem cell is extended that is surrounded by rosette-shaped bi- or multi-ciliated ependymal cells (Mirzadeh et al., 2008). Mirzadeh et al. (2008) suggested that this structure is essential for type B cells to contact the cerebrospinal fluid to regulate their behavior, and direct neural stem cell symmetric and asymmetric division. Ependymal cells also regulate neurogenesis (Omiya et al., 2021) through expression of Noggin, which promotes neurogenesis and inhibits differentiation toward glial cells by providing a neurogenic environment (Lim et al., 2000).
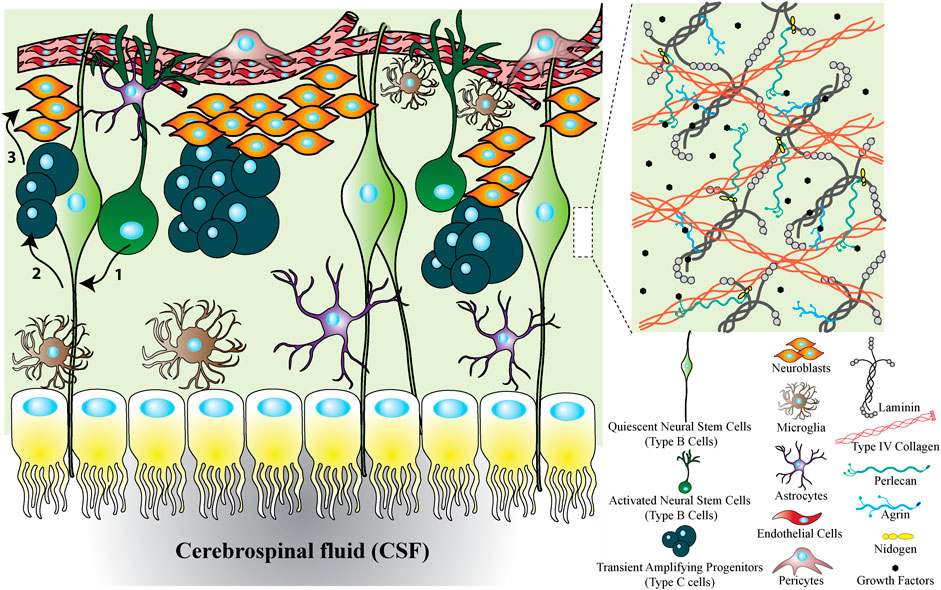
FIGURE 2. SVZ niche to show the cellular and ECM factors interacting with neural stem cells. The SVZ niche is formed from different types of cells, ECM proteins and growth factors. The cellular cross section shows that the SVZ located between the CSF and vascular niche. Upon activation of quiescent neural stem cells, they transit to activated neural stem cells. Activated neural stem cells then divide symmetrically or asymmetrically to transit amplifying cells and they produce neuroblasts. Finally, neuroblasts migrate through rostral migratory stream to olfactory bulb where they differentiate to mature neurons. In addition to cells, the ECM forming the SVZ niche is mainly composed of insoluble factors including laminin, collagen, HSPGs (e.g., perlecan and agrin), and soluble factors including growth factors. Altogether, the SVZ niche is a combination of different types of cells, proteins and growth factors each of which can be influential on neural stem cell fate decisions.
Furthermore, microglia and astrocytes are two common glial cells available in the neural stem cell niche. Microglia as a resident immune cell have a key functional role in neural stem/progenitor cell modulation by surveying the microenvironment. Some reports note that resting microglia under homeostatic conditions are proneurogenic (Solano Fonseca et al., 2016). For example, microglia-secreted factors promote dopaminergic differentiation of human neural stem cells by regulating cell proliferation and apoptotic/necrotic cell deaths (Schmidt et al., 2021). Upon activation, depending on the activation phenotype, either pro-inflammatory M1 or anti-inflammatory M2 activation, microglia can act as neurotoxic or neuroprotective (Vay et al., 2018; Schmidt et al., 2021). M1 microglia promote astrocytic differentiation while M2 microglia favor neurogenesis (Vay et al., 2018). However, another report demonstrated dopaminergic neuronal differentiation due to both pro-inflammatory and anti-inflammatory, which makes the effect of microglial factors still questionable (Schmidt et al., 2021). Different populations of astrocyte-like cells reside in the SVZ niche, including activated and quiescent neural stem cells, and share many characteristics of mature astrocytes. For example, Type B cells are fusiform and possess fewer processes than astrocytes but still have a high density of projections extending from apical and basal processes (Platel and Bordey, 2016). In addition, Type B cells express the glial fibrillary acidic protein (GFAP) but not S-100β, while astrocytes express GFAP and S-100β and contact Type B cells in the adult SVZ (Ma et al., 2005). The interaction of astrocytes with neural stem cells and their progenies may regulate stem cell maintenance, self-renewal, neurogenesis, migration, and neuronal maturation (Ma et al., 2005).
In the SVZ, the communication between the vascular and neural cells is also necessary for appropriate development and function (Karakatsani et al, 2019). Endothelial cells are the most studied cells in this region that play roles within the neural niche, even if not fully defined yet. Studies have found that endothelial cells promote neural stem cell survival and maintenance (Karakatsani et al., 2019), and endothelial cell-derived laminin sustains proliferation and multipotency of neural stem cells through interactions between α6β1 integrin and laminin (Rosa et al., 2016). Furthermore, Shen et al. (2008) showed that blocking α6β1 integrin in neural stem cells inhibits their adhesion to endothelial cells, likely via deposited laminin, leading to altered position and proliferation in vivo. Endothelial cells also secrete vascular endothelial growth factor (VEGF) and neutrophin-3 (NT-3), which promote neural stem cell self-renewal, quiescence, and long-term maintenance (Delgado et al., 2014; Han et al., 2015; Lim and Alvarez-Buylla, 2016). In addition to the cellular composition of the SVZ niche, the soluble and insoluble factors are critical in forming the niche, which will be discussed in the next section.
2.2 Extracellular matrix proteins and growth factors in the SVZ
As mentioned above, neural stem cells are located in the SVZ in which there are different types of proteins, including soluble and insoluble proteins, that regulate the stem cells’ proliferation, differentiation, and migration. The cooperation of soluble and insoluble factors with neural stem cell’s receptors dictates their response to differentiate or to maintain multipotency and self-renewal. Therefore, we will explain the soluble and insoluble factors available in the SVZ niche and how they are connected to each other.
To discuss the soluble factors, we begin with some of the insoluble structures and components of the niche. Both neural stem cells and their progenies directly contact blood vessels covered with the basement membrane, a thin sheet-like cell-adherent extracellular matrix. Almost 50 ECM proteins have been introduced as basement membrane proteins (Manabe et al., 2008). In the SVZ, there are two types of basement membranes, including vascular basement membranes and fractones (Manabe et al., 2008). Vascular basement membranes are underlying the endothelium, encasing the pericytes, ensheathing the smooth muscle cells, and creating the outermost fibrous matrix layer of a blood vessel. Vascular basement membrane proteins are mainly produced by endothelial cells, pericytes, and smooth muscle cells (Yousif et al, 2013). Fractones are structures consisting of aggregated extracellular matrix molecules adjacent to neural stem cells in the intermediate SVZ niche and ependymal niche. Discovered for the first time by Mercier et al. (2002), they described them as structures regularly distributed along the ventricle wall as puncta and named them fractones because of their fractal geometry (Mercier et al., 2002). As puncta, a fractone’s size is about 1–6 µm, but their specific branched morphology makes them accessible to numerous neural stem cells and other cell types available in the SVZ niche, suggesting the potential role of fractones as adhesive sites for resident cells in the SVZ (Sato et al., 2019) (Mercier, 2016). Fractones resemble vascular basement membranes compositionally (Figure 3); they both are mainly constituted of heparan sulfate proteoglycans (perlecan and agrin), laminins (laminin α5, laminin β1/2, and laminin γ1), type IV collagen, nidogen-1/2, SMOC-1/2, each of which has effects on neural stem cell behavior in neurogenic niche (Mercier et al., 2011; Yousif et al., 2013; Mercier, 2016; Sato et al., 2019; Melrose, 2022). However, fractones include type I collagen and laminin α3, which are absent in vascular basement membranes. On the other hand, vascular basement membranes, not fractones, contain laminin α2/4 and WARP (Sato et al., 2019). It is worth noting that the presence of laminin α3 and laminin α2 in fractones is not still clear since some literature demonstrated their presence and others did not (Kazanis et al., 2010; Nascimento et al., 2018; Sato et al., 2019). In contrast with vascular basement membranes, the cells that produce fractones are still under investigation. Sato et al. (2019) showed that fractones are deposited by neural stem cells while Nascimento et al. (2018) revealed that ependymal cells are the cells producing them; however, it is evident that they are in close contact with both cells (Figure 4). Furthermore, it is still unclear whether fractones are extending from vascular basement membranes or independent structures with different functions. Sato et al. (2019) found that fractones are structurally independent of vascular basement membranes, although fractones were first identified as fractal structures extending from vascular basement membranes. Some researchers have demonstrated the presence of fractones within the tissue parenchyma even before the development of blood vessels (Mercier, 2016); however, Sato et al. (2019) found that fractones became apparent at postnatal day 5 and better observed at postnatal day 7–10 when the mature ependymal cells were differentiated and neural stem cells emerged. This result was consistent with another finding where fractone bulbs appeared during the first week after birth (Nascimento et al., 2018). However, regardless of their origin, it is clear that basement membrane and fractones play important roles as both insoluble interaction points for cells and structural reservoirs for soluble factors throughout the niche.
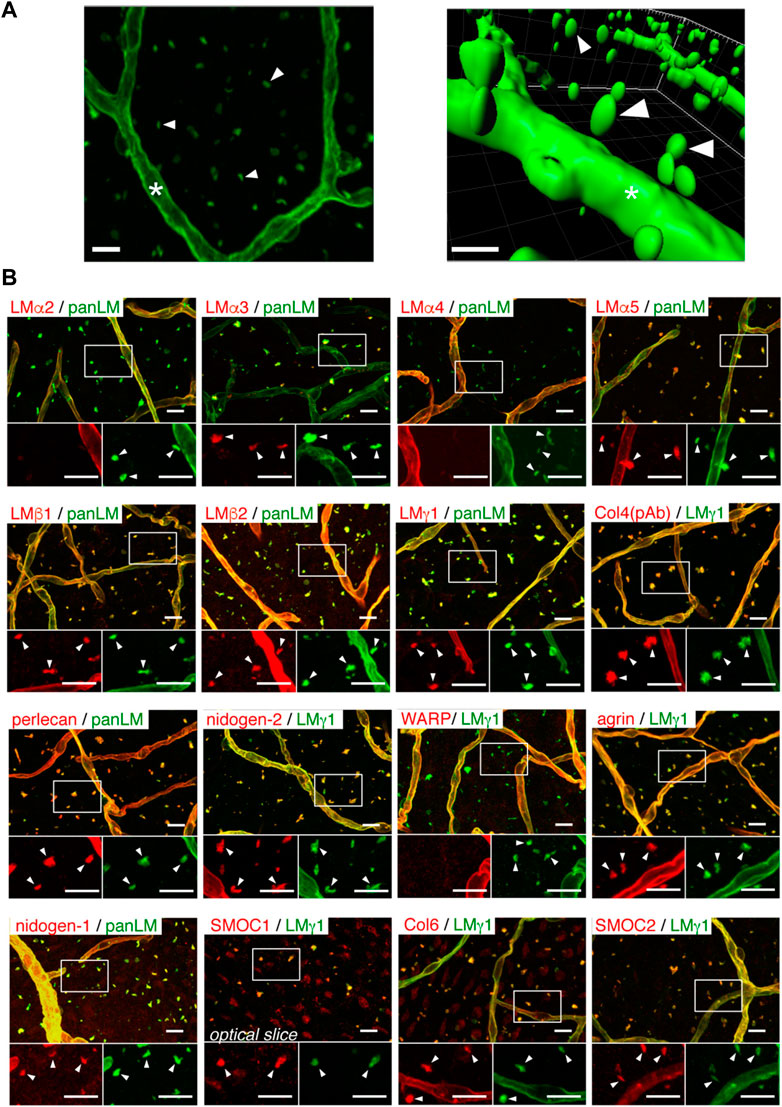
FIGURE 3. Fractones are visible as BM speckles in whole-mount V-SVZ immunostaining. (A) Whole-mount images of a mouse V-SVZ stained with an anti-panLM antibody. Left, image from the ventricle. Right, three-dimensional reconstruction of the left panel. (B) Whole-mount V-SVZs were labeled with antibodies against individual BM proteins (red) together with anti-panLM or anti-LMγ1 antibodies (green). Each panel shows a merged image (top) and higher-magnification images (bottom) of the two channels in the boxed area. Asterisks, blood vessel BMs; arrowheads, speckled BMs. Scale bars, 10 μm. Adapted from (Sato et al., 2019) with permission.
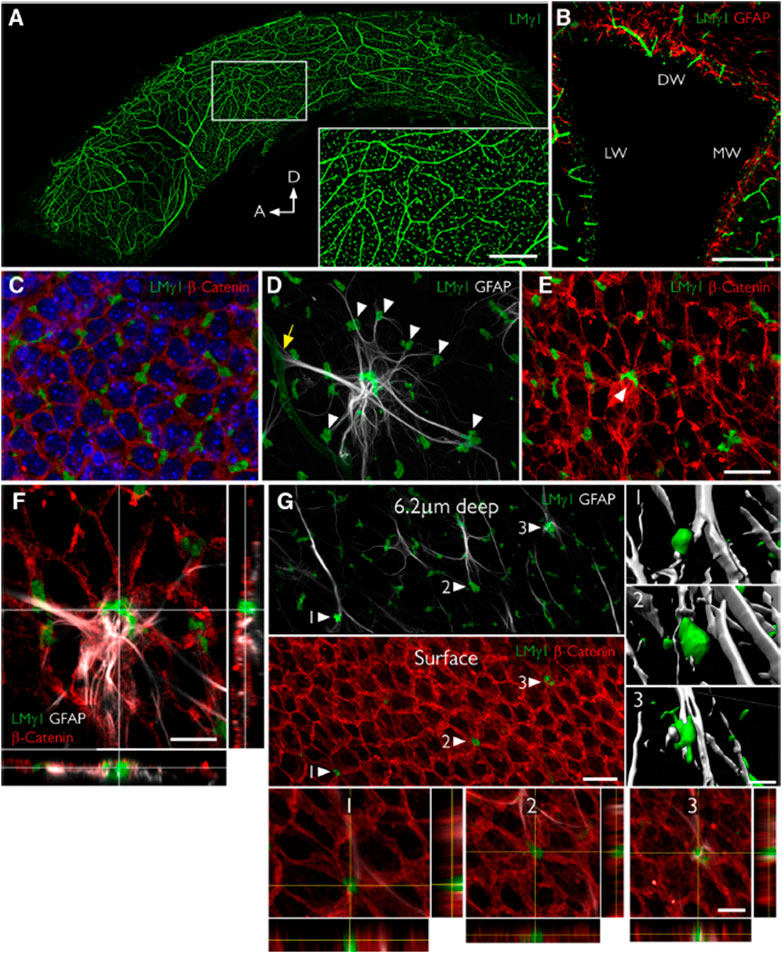
FIGURE 4. Fractones are a major BM source in the SVZ and appear at the center of pinwheels. (A), Whole mount of the lateral wall of the lateral ventricle of an adult mouse immunofluorescently stained for laminin γ1 (green). The frontal view reveals the profuse distribution of fractone bulbs. A higher magnification of the boxed area is depicted in the inset. (B), Coronal section through the lateral ventricle, showing the uniform distribution of laminin γ1+ fractone bulbs (green) on all three walls: dorsal wall (DW), lateral wall (LW), and medial wall (MW). (C), Frontal view of the ependymal cell layer, seen at 1 μm depth relative to the ventricular surface, stained for laminin γ1 (green) to visualize fractones and β-catenin (red) to visualize cell junctions in the ependymal layer. All fractone bulbs sit at the interface between cells. (D), Maximum intensity projection of a Z-stack of a whole mount showing a GFAP + NSC (gray) in contact with one blood vessel (yellow arrow) and >10 fractone bulbs (arrowheads) at the same time. (E), The neural stem cell body also contacts a large bulb located at the center of a pinwheel (arrowhead). (F), Orthogonal views revealing that the NSC–fractone interaction seen in (C,D) occurs at the surface of a pinwheel center. (G), GFAP + processes contact more bulbs at pinwheel centers (arrowheads) than bulbs at the basolateral surface of ependymal cells. Interactions between GFAP + processes and fractone bulbs were labeled 1–3 and rendered, revealing that GFAP + processes establish direct and complex contacts with fractones. Orthogonal views of these interactions confirm that fractone bulbs sit between ependymal cells at pinwheel centers and that GFAP + processes directly contact them. Scale bars: (A), 500 μm; (A), inset, 200 μm; (B), 200 μm; (C–E), 25 μm; (F), 12 μm; (G), 25 μm; (G), 1–3, 12 μm. Adapted from (Nascimento et al., 2018) with permission.
As a component of basement membranes and fractones, heparan sulfate proteoglycans (HSPGs) are considered potent regulators of cellular responses in most niches, including the SVZ niche, as their side chains reversibly bind various growth factors and cytokines serving as soluble factors. Therefore, the basement membrane and fractone storage of growth factors serves to promote the residence time and potency of these growth factors (Mercier, 2016). While many growth factors influence cells within the niche (Table 1), the majority of these factors, including Wnt, SHH, BMP, VEGF, FGFs, TGFs, IGFs, and EGFs, are all heparin-binding factors, highlighting the importance of insoluble matrix composition to transduce biological signals and stimulate neural stem cell’s proliferation or differentiation. Perlecan and agrin are considered as the most common HSPGs available in the SVZ. Perlecan is an HSPG with five protein domains (I-V) with a critical role in developmental neurogenesis by maintaining basement membrane integrity and angiogenesis. Lee et al. (2011) indicated the neuroprotective and angiogenic role of perlecan domain V by associating a higher level of VEGF. In addition, the interaction of perlecan with laminin and FGF-2 enhanced the neural stem cell’s proliferation, while its interaction with BMP growth factors inhibited the cells’ proliferation (Melrose, 2022). Wnt and ShH morphogens can also bind to perlecan to regulate neural stem cell proliferation and differentiation (Yang et al., 2019; Melrose, 2022). Agrin is another HSPG available in the SVZ, whose effect on neural stem cells is less studied compared to perlecan. Thus, HSPGs, as a basement membrane and fractone components are crucial in regulating neural stem cell’s proliferation and differentiation by associating with both growth factors and neural stem cells.
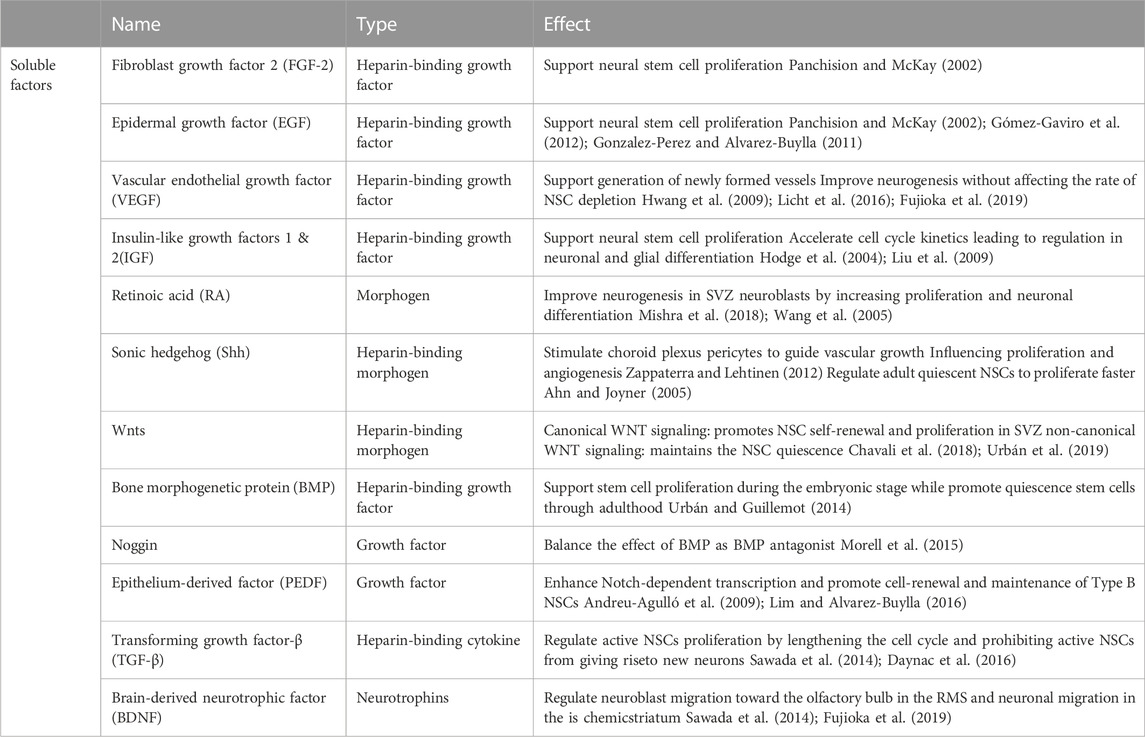
TABLE 1. Soluble growth factors within the neural stem cell niche contribute to neural stem cell maintenance and differentiation.
The other two important insoluble components present in basement membrane and fractones are laminin and collagen proteins serving as mediators between HSPGs, growth factors and cell receptors. Collagen I and collagen IV are two types of collagens available in the SVZ neurogenic niche (Sato et al., 2019). However, while collagens are commonly studied as cellular adhesive proteins for much of the body, laminin is the most studied adhesive ECM protein in the SVZ niche. Laminin α5, laminin β1, laminin β2, and laminin γ1 are the most common types of laminins expressed in basement membranes and fractones. As shown in Figure 5, cells interact with laminin through various receptors including non-integrin and integrin receptors. Non-integrin laminin receptors include 67 kDa laminin receptors, 110 kDa laminin receptors, dystroglycans, lutherans, syndecans, and melanoma cell adhesion molecule. Oikari et al. (2016) reported high expression of syndecans 1/2/3 in neural stem cells and a considerable change in their expression profile while the culture was extended or differentiated, showing their key contribution in stemness and differentiation. In thinking about materials design, it would be important to consider that the level of expression of syndecan and Lutheran increases in activated neural stem cells compared to quiescent ones (Kazanis et al., 2010; Mouthon et al., 2020). However, of the various types of laminin receptors, integrins are the most widely recognized and studied. Integrins are heterodimeric membrane proteins mediating cell-ECM interactions broadly. Formed of non-covalently associated α and β chains, from which 24 integrins have been identified in mammals having selectivity toward collagens, fibronectin, vitronectin, laminins, etc. (Humphries et al., 2006; Yamada and Sekiguchi, 2015; Isomursu et al., 2019), integrins play a significant role in bidirectional signal transmission between cells and their microenvironment. Integrin-binding interactions regulate cell functions, integrating biochemical (integrin activation) and biomechanical (mechanotransduction) signals through conformational changes in their ectodomain, which increases the affinity of binding (Sun et al., 2016). Due to the key role of β1 integrin in forming the neuroblast chain during development (Fujioka et al., 2017), β1 integrins are generally studied more often as modulators of cell activity in the brain. β1 integrins promote the neuroblast adhesion to laminin and allow the translocation of cells through migration (Fujioka et al., 2017). In addition to the migration effect, both neural stem cells and progenitor cells express α6β1 integrin in the SVZ region, which is believed to contribute to SVZ cell proliferation and stemness (Rosa et al., 2016). α6β1 integrin binds to laminins 111, 322, 511, and 521; the latter two are the most preferred ones (Nishiuchi et al., 2006). Neural stem cells express a high level of integrin α6β1 in addition to the expression of Sox1-3 and nestin; the selection of any of which was similarly effective in enriching human-derived neural stem cells from neurospheres (Hall et al., 2006); expression of α6β1 integrins is lost as they differentiate (Shen et al., 2008). α3β1 integrin is another key integrin required for proper neuronal migration and placement during the development of cerebral cortical (Schmid et al., 2004). However, while α6β1 integrin interactions control adult SVZ-derived neuroblast migration (Emsley and Hagg, 2003), α3β1 integrin impairment alters the neuroblast migration directed radially and tangentially (Schmid et al., 2004). Continued study will likely uncover other critical interactions, but key for this paper is that cells currently express and utilize these binding interactions to guide the cellular response of neural stem cells.
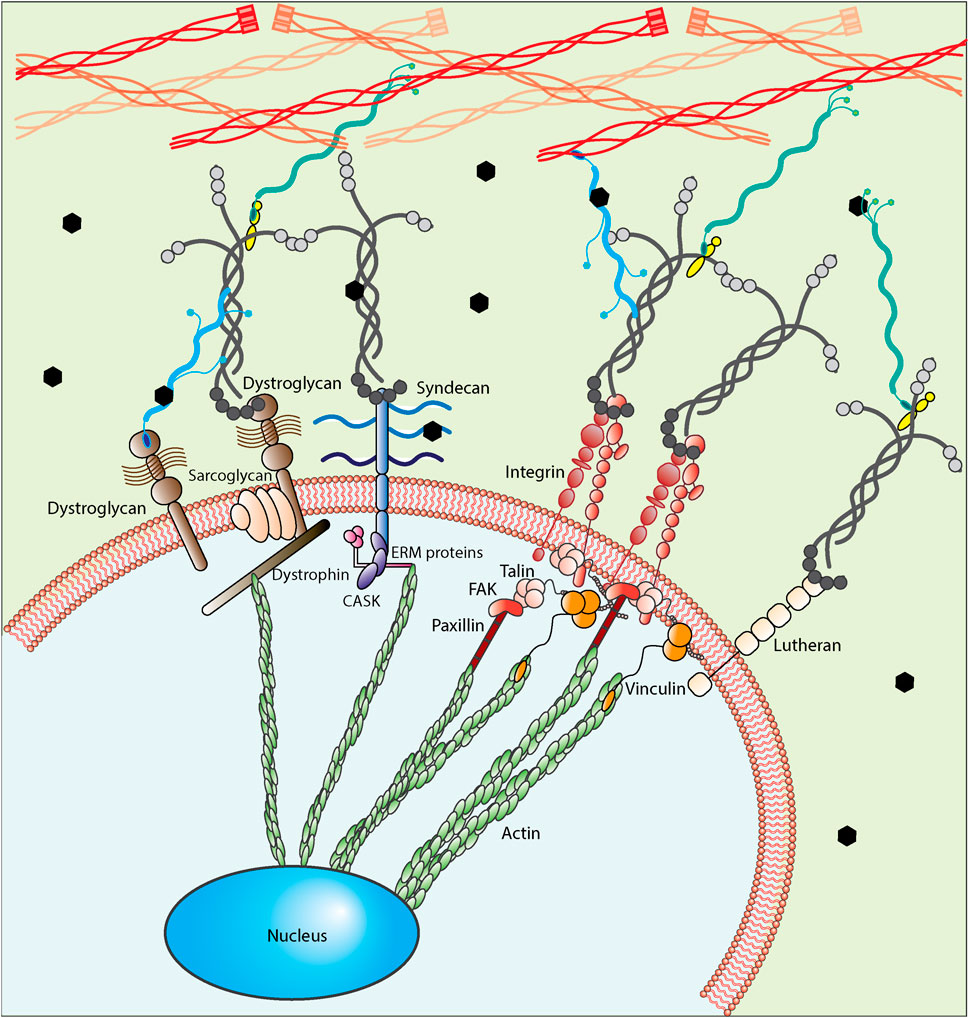
FIGURE 5. A close up of cell-ECM interaction. Neural stem cells interact with their microenvironment using different types of receptors including non-integrin laminin receptors (Dystroglycan, Syndecan and Lutheran) and integrins. A physical link between cells and ECM proteins exists, utilizing integrin or non-integrin receptors based on the biochemical nature of the microenvironment. These interactions can be further classified as nascent adhesions and focal adhesions and further allow cells to distinguish the structural components of the microenvironment through their bonds or interaction points. For integrin-mediated interactions, the first step is called integrin activation, through which integrins go from a bent-closed conformation with low-affinity ligand binding to an extended conformation with high-affinity ligand binding. After forming the bond between integrins and their ligands, talin binds to integrin cytoplasmic tail promoting integrin activation. Upon integrin activation, integrins form clusters adhering to the ligands. They then recruit adaptor proteins (e.g., vinculin, FAK, and paxillin) facilitating the formation of a signaling complex at the cytoplasmic tails and the formation of the nascent adhesion accordingly. These nascent adhesions are transient force-independent structures whose formation does not rely on myosin-II activity. Finally, through the third step, the adhesions are mostly disassembled, but a few of them persist, associate with actin filaments polymerized at the leading edges and develop into focal adhesions, which are force-dependent. FAs are highly dynamic structures that are stable compared to NAs and require further integrin clustering. For dystroglycan-mediated interactions, the sarcoglycans form a complex of membrane proteins and dystrophin with the dystroglycan complex. Dystrophin then binds to actin cytoskeleton to provide the link between the extracellular matrix and cytoskeleton and finally nucleus. For syndecan-mediated interactions, it links to actin cytoskeleton using CASK and ERM (ezrin, radixin and moesin) proteins. For Lutheran-mediated interactions, the cytoplasmic domain interacts with spectrin-based cytoskeleton, not actin cytoskeleton.
Therefore, insoluble and soluble components forming the fractones and basement membranes are signaling the neural stem cells individually and in concert with each other to direct the fate decision. Knowing the effective factors in the SVZ niche, we as engineers need to know what will happen if these factors do not act as normal due to aging, disease and injuries. We then are required to find a way to make them act as normal again or decrease the deleterious effects of the imbalance factors. In the following section, we will talk about some studies on the SVZ changes, and the potential development of biomaterials engineers are suggesting.
3 The changes in SVZ niche by aging/disease/injuries
Brain function deteriorates with age, disease (e.g., neurodegenerative diseases including Alzheimer’s and Parkinson’s diseases), and injury from which the SVZ niche is not protected. This deterioration can result in misfunction of niche factors, including unexpected cell-cell interaction (Capilla-Gonzalez et al., 2013) (Solano Fonseca et al., 2016), abnormal structural composition (Kerever et al., 2021), or alteration of stiffness (Segel et al., 2019; Ryu et al., 2021; Takamura et al., 2020) that impact the proliferative and neurogenic potential of the SVZ region (Urbán et al., 2019). Specific examples are provided below to show how the imbalance of factors could affect the niche function.
Cellular organization and cell number within the SVZ niche are impacted by aging, affecting cellular interaction with their surrounding microenvironment. While a similar number of ependymal cells, astrocytes, and oligodendrocytes exist with aging, reduced numbers of neural stem cells, progenitor cells, and neuroblasts occur in mice (Capilla-Gonzalez et al, 2015; Capilla-Gonzalez et al., 2013). An example of the detrimental effect of this imbalance can be seen with neuron migration. Decreases in the number of neuroblasts and their tendency to form smaller chains lead to the disappearance of the migration chain with age (Capilla-Gonzalez et al., 2013). Aging alters microglial activation that might contribute to the increased level of proinflammatory M1 cytokines and, therefore, reduced neurogenesis while aging (Solano Fonseca et al., 2016). Disrupting the cellular balance also disturbs the gliogenesis: neurogenesis balance (Capilla-Gonzalez et al., 2013). In the SVZ, transit-amplifying cells, activated astrocytes, and endothelial cells express BMP to favor gliogenesis, while ependymal cells express noggin to promote neurogenesis (Larripa and Gallegos, 2017). Increasing activation of microglia can cause an increase in BMP signaling, which has been previously found in the aged murine hippocampus to inhibit neural progenitor cell proliferation and subsequent neurogenesis (Yousef et al., 2015). Upon CNS injury, neural stem cells tended to differentiate into astrocytes through activation of a BMP-2 transcriptional regulator, Id3 (Bohrer et al., 2015). Therefore, regulation of noggin and BMP in the SVZ niche alter signaling balances during aging and injury, resulting in aberrant niche function.
In addition to cellular changes, the structural composition of the SVZ niche changes during aging. Kerever et al. (2021) reported a change in the composition of fractone’s heparan sulfate size and sulfation that correlate with decline in neurogenesis in an aged brain. Since the complexity of fractones is increased during aging, laminin, as another critical factor, might undergo changes as well. Recent findings show that a lack of laminin α5 chain increased the proliferation and decreased the quiescence of neural stem cells, possibly through TGF-β signaling (Nascimento et al., 2018). Furthermore, in age-dependent lineage decisions of neural stem cells, the lack of integrin β1 led to astrocyte differentiation (Brooker et al. 2016). The vasculature also plays a role in extracellular matrix availability within the niche. Zhao et al. (2021) found vasculature changes with age and reported the sex-dependent nature of diameter and vessel density. With these vascular changes, the decline in neural stem/progenitor cell number and proliferation was significant in males in aged mice, but not female (Zhao et al., 2021). They also studied the effect of vasculature change in neuroblast migration, and found a reduced neuroblast chain density by age in both sexes, which is in line with other reports, but more disorganized in male comparing to female (Zhao et al., 2021).
The material property of the niche as defined by stiffness is a critical factor where change may influence neural stem cell differentiation spatially and temporally (Kjell et al., 2020). Several studies reported heterogenous tissue stiffness in rodent brains from neonatal to adulthood (Ryu et al., 2021). As an example, Segel et al. (2019) reported that an aged brain is stiffer than a young adult and neonate brain; however, Takamura et al. (2020) reported a significant decrease in stiffness with age, except posterior lobes and cerebellum. Potential reasons for stiffness changes in the brain have included gray matter volume (Takamura et al., 2020), neuronal structure (Ryu et al., 2021), or ECM composition (Ryu et al., 2021). For example, higher quantities of insoluble ECM proteins correlated to increased stiffness of SVZ niche compared to cortical gray matter (Kjell et al., 2020), suggesting that changes to basement membrane composition in the SVZ with age will alter the stiffness. Due to a correlation between the environmental stiffness and senescent phenotype (Blokland et al., 2020), the lack of a cellular response can be connected to disease onset and progression that alters stiffness. Therefore, changes in stiffness of the aged/diseased brain can suggest the importance of this factor while designing disease models.
Knowing the potential changes that might occur due to aging and pathology, each factor can be examined with reductionist point of view to understand their potential effect on the neural stem cells’ changes. Engineers have therefore developed and examined biomaterials to mimic the microenvironment physical, chemical, and physiological properties. In the next section, we will discuss about the currently available biomaterials and designing considerations utilizing the knowledge we have from neurogenic niche, SVZ niche.
4 Biomaterials to mimic the niche
Neural stem cells are considered a potential source to give rise to new neurons and regenerate lost brain function. Extracellular cues are vital to regulate neural stem cell fate and function. In this regard, engineers are poised to address challenges related to brain injury or aging—while the full design constraints are yet to be laid out, the neural stem cell environment offers many clues to better design a response to altered disease states. From a reductionist point of view, engineers can apply these clues by selecting the appropriate backbone biomaterial type and regulating its mechanical properties, topographical features and biodegradability status matching the neural stem cell’s niche properties (Figure 6). Furthermore, they should consider adding components that make the targeted biomaterial bioactive for neural stem cell interaction (Figure 6). Considering this, biomaterials can serve as a platform containing the desired clues, including both the compositional and structural elements, as either a platform for cellular growth and proliferation in vitro or a cell/drug/DNA carrier to be transplanted in vivo. It is important to note that a functional biomaterial requires decoupling individual properties to allow understanding the effect of each component on the biological response. Therefore, the engineers’ attempt is to design and develop functional and applicable scaffolds with defined compositional and structural characteristics to produce the desired neural stem cell response.
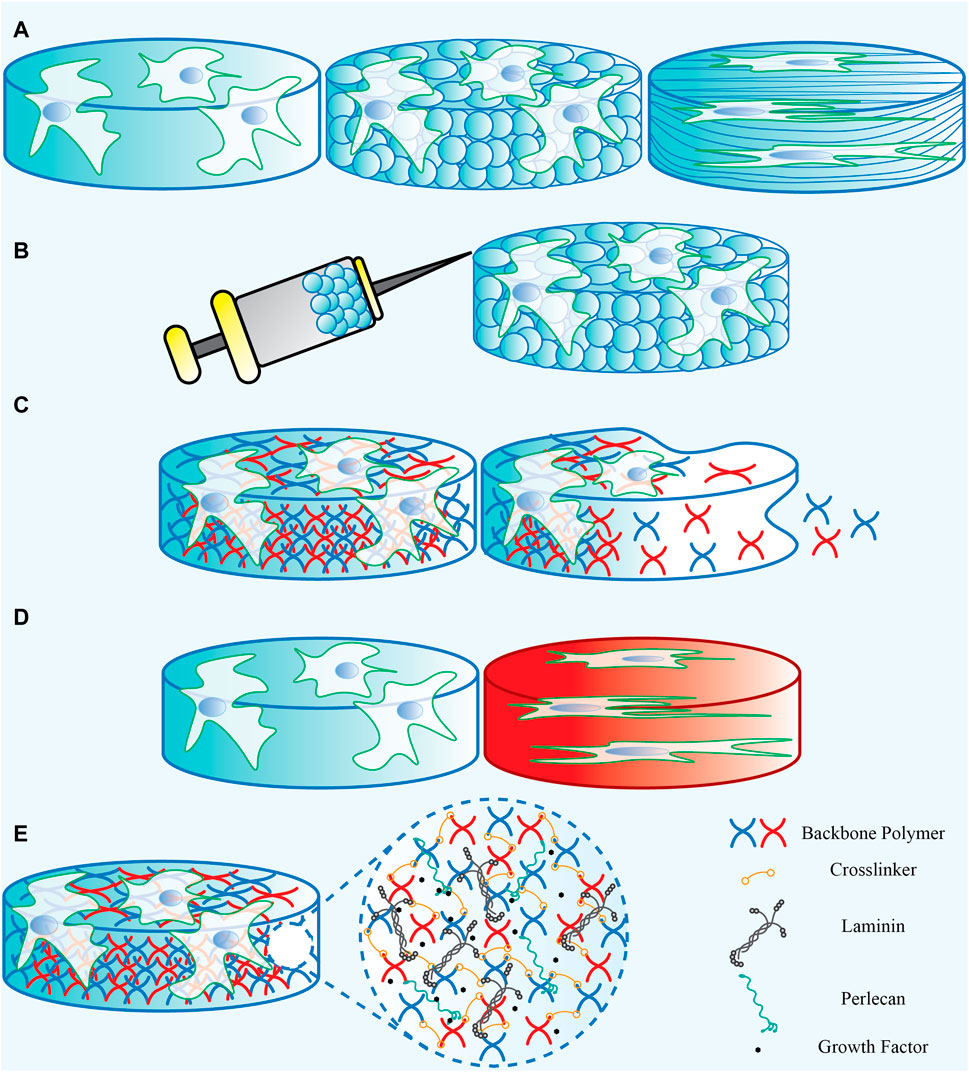
FIGURE 6. A representation of the biomaterials’ (A) physical properties, (B) Injectability, (C) degradability, (D) mechanical properties, and (E) the bioactive components that can be added.
4.1 Biomaterial properties
4.1.1 Backbone biomaterial
To select the backbone biomaterial, the main consideration should be based on the fact that the brain tissue is one of the softest tissues in the body. In this regard, many natural biomaterials or synthetic polymers have been examined as backbone structures to mimic lower mechanical properties. Table 2 provides a list of commonly used natural and synthetic biomaterials in neural stem cell studies, each of which has some pros and cons required to be considered [refer to review paper (Doblado et al., 2021)]. Natural biomaterials can be derived from either ECM proteins or the polysaccharides produced in other organisms. They are popular because of their biologically active nature and their excellence in promoting cell adhesion and growth (O’Brien, 2011). Moreover, natural biomaterials are biodegradable and are easily remodeled by cell-mediated proteases such as matrix metalloproteinases, allowing cells to deposit their own ECM and grow (Kamatar et al., 2020; Caliari and Burdick, 2016). Many natural biomaterials have direct analogues in the human body, reducing the risk of cytotoxic reactions (Boni et al., 2018). Although natural biomaterials have been widely used due to their advantages, certain drawbacks can still make their use limited. First, most of the natural-based biomaterials are large and complex proteins making them hard to be produced through a recombinant expression system. Therefore, they are mainly purified from an animal- or human-derived ECM and have the risk of pathogen and immunogen contamination and lot-to-lot variability. A lack of control over their mechanical properties, thermal sensitivity, and induced biological signals due to complex chemical structures make their broad use for a reductionist’s view more challenging. Synthetic polymers have several advantages over natural ones, including the potential to maintain a range of mechanical (e.g., stiffness) and biochemical (e.g., ligand concentration) properties. They can also be designed in different forms, including bulk hydrogels, microgel-based scaffolds, and fiber-aligned polymer scaffolds to provide different types of topographical features for inducing favorable responses. One key drawback, however, is that most synthetic materials lack biological signaling capability. Therefore, they need to be modified with natural bioactive materials to promote cell adhesion, activation, and to ultimately be remodeled by cells (Kamatar et al., 2020). The backbone biomaterial is usually selected based on the application under investigation and choosing between natural and synthetic biomaterials depends on the priorities of each study. Because of the complex nature of the natural tissue, many structural and compositional improvements are still needed to make both natural and synthetic biomaterials better candidates for neural stem cell studies.
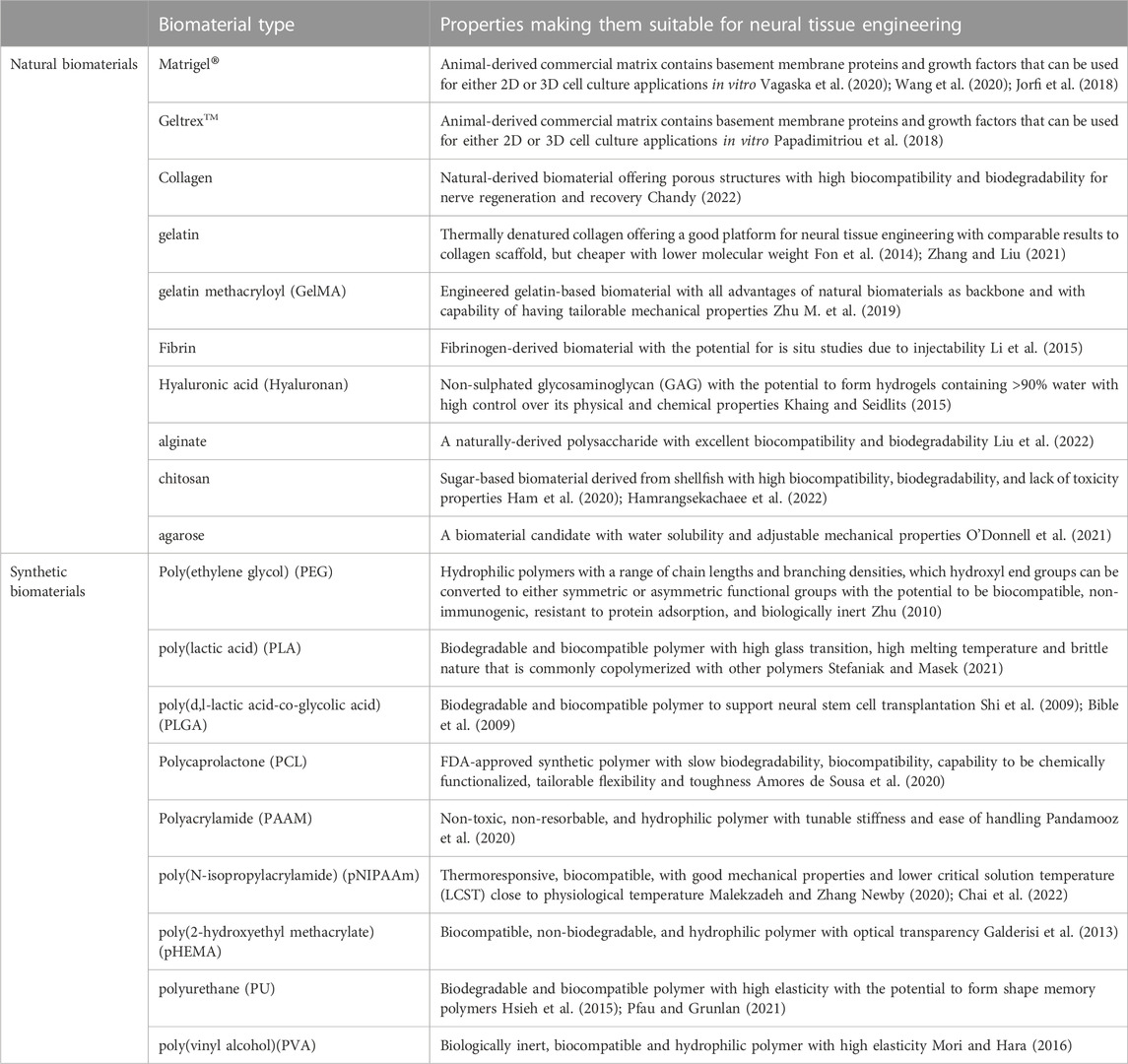
TABLE 2. The list of backbone biomaterials that are commonly used in neural stem cell’s niche studies.
4.1.2 Degradability
Degradability is one of the important factors to be employed if the scaffold is to provide support for cells to grow, as degradation is required to make space for proliferating cells. While natural biopolymers typically have sequences within their backbone that are broken by enzymes such as matrix metalloproteinases within the body, synthetic polymers must be designed with degradability in mind. Researchers have long investigated co-polymerization with a biodegradable polymer, such as PLA or PGA, to add degradability to otherwise non-degradable polymers. More recently, various groups have shown that matrix metalloproteinases can be incorporated into synthetic polymer systems to support cellular degradation (Barros et al., 2019) (Lutolf et al., 2003) (Lévesque and Shoichet, 2007). For applications to neural systems, the addition of matrix metalloproteinases-sensitive cleavage peptides to PEG-heparin-hydrogel system supported the generation of elaborate neuronal network while modeling Alzheimer’s disease (Papadimitriou et al., 2018). The advantage of using naturally occurring proteinase-sensitive materials is that the degradation of these crosslinkers relies on cell demand, so using them allows tuned rates of degradation based on tissue remodeling. In the design process, both the rate of biodegradability and degraded byproducts should be considered since accumulation of degraded components can instigate high astrocyte response, resulting in the glial scar formation and inflammatory response (Bjugstad et al., 2010). Furthermore, the byproducts can induce new signals to stem cells influencing their function and fate decisions (Murphy et al, 2014). In addition, the mechanical properties of the environment where the cells are encapsulated varies dynamically as the material degrades, and an important design consideration is a balance between providing space for cell growth and vascularization and the strength of the scaffold (Peressotti et al., 2021). Therefore, decoupling the cellular infiltration and scaffold’s mechanical properties is one of the main challenges that engineers have faced when using degradable biomaterials.
4.1.3 Mechanical properties
Mechanical properties of a biomaterial are key factors that need to be intentionally designed into scaffolds to mimic neural stem cell niche. Failure of tissue integration due to mechanical mismatch between a biomaterial and its surrounding tissue has been well described in other systems such as bone or cardiovascular tissue (Soliman et al., 2022; Boccafoschi et al., 2017), and when selecting materials for use, it is an important design constraint here due to the brain’s soft nature. Biomechanical measurement of brain tissue is challenging due to its softness, leading to inconsistent findings by comparing different reports; the properties of the brain vary with age, sex and disease (Budday et al., 2017). Recently, the human mechanical properties could be measured non-invasively using magnetic resonance elastography (Hiscox et al., 2016). Hiscox et al. (2016) reviewed forty-one studies using magnetic resonance elastography to measure the brain shear stiffness of healthy and patient participants with an age range between 16 and 94 years old. They reported the mechanical properties of human brain as a whole with a range of shear stiffness from .62 kPa to 2.99 kPa with a mean shear stiffness of 2.07 kPa ±.42 kPa at actuation frequency of 50 Hz in healthy participants and showed significant changes in shear stiffness due to different types of neurological disorders [for full review, see (Hiscox et al., 2016)]. Therefore, mechanical properties of a biomaterial used for brain tissue regeneration should then match the native brain tissue in healthy young—and not pathological—conditions to match the desired response of the niche appropriately. In this respect, Saha et al. (2008) demonstrated that soft substrates promote neuronal differentiation while stiffer ones supported glial differentiation for the first time. Following that report, Leipzig and Shoichet (2009) reported a consistent result that soft substrates with E < 1 kPa enhanced neuronal differentiation; medium-stiff substrates with 1 kPa < E < 3.5 kPa favored astrocyte differentiation, and stiff substrates with E > 7 kPa promoted differentiation toward the oligodendrocytes. Furthermore, using PEG-based hydrogels decorated with RGD, Stukel et al. found that softer scaffolds (.1–.8 kPa) provided a better platform for neural stem cell differentiation and neurite extension compared to stiffer ones (4.2–7.9 kPa) (Stukel and Willits, 2018). In addition, Segel et al. (2019) found that aged oligodendrocyte progenitor cells (OPCs) could be rejuvenated when cultured on young-mimicked stiffness scaffolds. These types of studies highlight the importance of scaffold mechanical properties in lineage decisions. Therefore, both investigation into changes in vivo under pathological conditions and designing biomaterials with tunable properties are top priorities in harnessing the therapeutic potential of neural stem cells (Zhu M et al., 2019; Zhu Y et al., 2019).
4.1.4 Physical properties
Like mechanical properties, cells sense the physical environment, e.g., topography and architecture, and are therefore important when studying designing scaffolds for neural stem cells. Flat, 2D cultures have long been studied in vitro; however, they are not representative of in vivo conditions which are inherently 3D. A 2D system could affect the cell response since the distribution of cues that cells receive is different in 2D culture than 3D culture. Therefore, the ideal in vitro model is 3D model in which the cells are encapsulated, as it better mimics the natural niche of neural stem cells. However, both 2D and 3D cultures can have different spatial and physical features from the nanoscale to microscale in a variety of shapes including plain, fibrous, or spherical structures with rough or smooth characteristics.
One method of introducing topography into this system is through fiber formation. Fibers can be formed by various techniques and used for neural tissue engineering [for full review, see (Uyar Tamer, 2017)]. However, the diameter (Christopherson et al., 2009) and alignment (Amores de Sousa et al., 2020) of fibers has influenced the neural stem cell response. For example, higher neuronal differentiation occurs on larger fiber diameters compared to smaller fiber diameters with laminin-coated polyethersulfone fibers (Christopherson et al., 2009). Without the presence of any growth factors, electrospun blended-polycaprolactone fiber mats induced higher levels of glial or neuronal differentiation of the neural stem cells depending upon the hydrophilicity of the mats (Fernández et al., 2019), so care should be taken to fully evaluate the material properties of samples. The next step for fibers is to be able to functionalize with molecules that instruct neural stem cell response. For example, fibers modified with adhesive RGD ligands were designed to guide neural stem cell distribution and promote higher cell elongation leading to neuronal differentiation (Amores de Sousa et al., 2020). In addition, spatial control over peptide or protein functionalization was achieved to increase and direct cell migration, regulate neural differentiation, and gradient formation, allowing for increased control over how bioactive cues are presented (Silantyeva et al., 2019; Cavanaugh et al., 2019; Motta et al., 2019; Silantyeva et al., 2018). Manufacturing systems that regulate both the topographical nature of the fibers at scale, as well as the bioactive moiety placement, are not broadly available and need more engineering development to have a wider impact.
Another common method to introduce topography is by fabricating granular platforms. Granular scaffolds offer many favorable properties, making them appealing in neural stem cell studies. These materials offer the ability to piece together different structures like Lego® bricks to regulate the properties of the scaffold. From a topographical view, the granular structure is thought to better mimic the brain structure (George et al., 2020). One such granular construct is the microgel-based scaffold, which uses micro-sized hydrogels as the basic brick to then link together to form a larger structure (Figure 7) (Zhou et al., 2016). The microgels are built and modified chemically and mechanically prior to forming the desired structure and then packed with/without encapsulated cells for their final applications (Scott et al., 2010b; Scott et al., 2011). They can be formed either in an array, film, or 3D structure to provide topographical cues to cells (Riegert et al., 2021). Wilson et al. (2022) showed that engineered microgels can serve to promote the neural stem cell’s neurogenesis or help them to keep their multipotency depending on how they are post-modified with the laminin-derived peptides. Because of the granular nature of the system, the environment of microgel-based biomaterials can either be homogenous, providing encapsulated cells similar signals throughout the scaffold, or can offer heterogeneous microenvironments to provide spatial regulation.
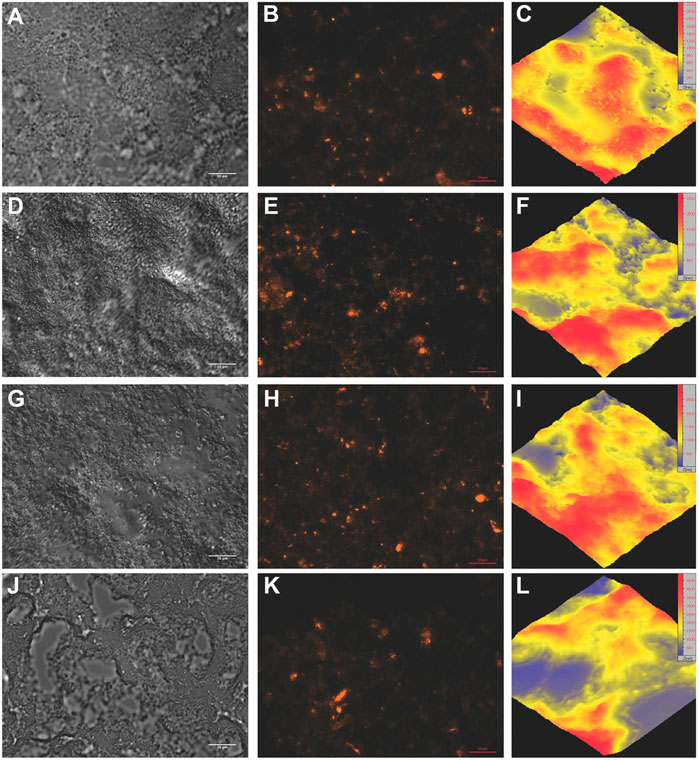
FIGURE 7. Microscopic evaluation of the microgel-based scaffold morphology and spacing within the scaffold. The images of the hydrated scaffolds were utilized to visualize the morphology of these scaffolds by DIC, fluorescence, and atomic force microscopy. Top row = 5 mg mL−1 PEG-VS, second row = 10 mg mL−1 PEG-VS, third row = 20 mg mL−1 PEG-VS, bottom row = 40 mg mL−1 PEG-VS. (A,D,G,J) Individual microgels can be discerned within the scaffolds, but are clustered into a structure, leaving gaps in the scaffold. This structure became more evident with increasing crosslinker concentration, where larger spaces were seen throughout the sections. Scale bars are 10 μm. (B,E,H,K) Homogeneous collagen distribution was confirmed by incorporating fluorescent collagen. No differences were seen with respect to crosslinker concentration. The images displaying the collagen are the fluorescent version of (A,D,G,J), respectively. Scale bars are 50 μm. (C,F,I,L) AFM (40 × 40 μm) of the scaffolds further confirmed the morphology seen by DIC, where the peaks of the network increased with increasing crosslinker concentration. Note thin increases in scale from blue (low) to red (high) with increasing crosslinker concentration. The increased bundling of the microgels also increased the spacing within the network. Adapted from (Zhou et al., 2016) with permission.
In addition to providing topographical cues, microgel-based scaffolds have been shown to have adjustable properties in all important features talked above to develop an appropriate scaffold for neural stem cell studies. These granular hydrogels can be easily fluidized while compressed because of shear thinning phenomena (Riley et al., 2019). Injectability is one of the aspects that engineers are working to improve. Although solid-like hydrogels are ideal for many aspects of brain tissue regeneration ex vivo, injectable and self-gelling systems are critical design parameters for use in vivo. In this regard, hyaluronic acid-based microgels were injected to the cavity post-stroke and found to reduce inflammation, astrocyte infiltration, and microglia activation. These changes made the environment suitable for triggering neural stem/progenitor cell migration toward the stroke cavity (Nih et al., 2017). They also studied the neural progenitor cell fate in these types of scaffolds designed with adhesive and non-adhesive laminin peptides; the first one led to the cell’s spread, migration and differentiation and the later one led to neurosphere formation, maintaining their level of stemness (Wilson et al., 2022). The microgel-based scaffolds also could be used as an alternative to solve the decoupled degradability and mechanical properties (Scott, Marquardt and Willits, 2010a; Scott et al., 2011; Coronel et al., 2022). Xin et al. (2018) demonstrated that microgel scaffolds, even without degradability potential, work better for cellular spreading comparing conventional bulk hydrogels, even with a degradable crosslinker peptide. The porous structure provides equal soluble nutrient accessibility and vascularization that make these microgels suitable for mimicking the native niche. Furthermore, the mechanical properties of microgel-based scaffolds are a result of the continuous phase and are usually determined by the polymer type, density and level of crosslinking (Daly et al., 2020). Combining these properties, plus the post-modification capability of the microgels, granular scaffolds are now considered as one of the most promising platforms as they meet most of the requirements to recapitulate the native tissue patterns. With the current technologies, we can only generate simple tissue patterns that replicate early tissue development; therefore, engineers need to expand the design technologies to make the scaffolds better meet all of the requirements for mimicking the natural tissue in vitro.
While designing scaffolds for neural stem cell studies, another important aspect is to make these scaffolds bioactive and enable them to support cell adhesion, growth and differentiation. The following section will delve more into the bioactive components that are currently common.
4.2 Bioactive components
Learning from the natural niche, bioactive components are required to be added to biomaterials to make them functional in neural tissue engineering. These components include residing cells, ECM proteins, growth factors, and proteoglycans which are commonly available in the SVZ niche to make the designed biomaterials suitable to interact with neural stem cells. In the following paragraphs, we summarize some of the most popular components using in the biomaterials appropriate for neural tissue engineering.
4.2.1 Laminin
Laminin is the most commonly studied ECM protein in the SVZ niche serving as an adhesive site. Therefore, adding naturally-derived, recombinant, or synthetic versions of laminin to biomaterial scaffolds can mimic the natural tissue by providing links for laminin receptor- or integrin-mediated cell adhesion. Laminin is not often used as a 3D scaffold by itself, but it has been used a lot with other natural ECM components (Willerth and Sakiyama-Elbert, 2019). Laminin 111 is the most studied type of laminin in neural stem cell studies since it can be easily isolated and purified from Engelbreth-Holm-Swarm (EHS) mouse sarcoma (Tate et al., 2009; Addington et al., 2015; Distler et al., 2021). There are also recombinant versions of laminin 111 protein, but their use is not still that common in neural stem cell cultures. Although natural-derived laminin 111 has been widely used for primary studies due to price and availability, laminin 111 is not appropriate for mimicking many disease models since it is only expressed in the CNS during embryogenesis, and it disappears progressively from basement membranes during development. Instead, laminin 511 and laminin 521 become ubiquitous during adulthood (Barros et al., 2020). Therefore, laminins 511 and 521 should be the preferred laminins to be utilized in engineered scaffolds for studying adulthood disease models. However, apart from mouse-derived laminin 111, other laminins, including laminin 511 and 521, are almost impossible to be isolated in their native form (Rodin et al., 2010). Although recombinant full-length laminins 511 and 521 are commercially available, they are large and difficult to be produced recombinantly. To overcome this challenge, the E8 fragment of laminin 511 and 521 is a functionally shorter form of laminins that supports integrin-mediated cell adhesion, enabling engineers to study the effect of niche laminin components. In this regard, Silva et al. (2017) showed that laminin 511 could support neural stem/progenitor cell adhesion and migration better than laminin 111 and laminin 511-based differentiation methods are used to produce clinical quality dopaminergic neurons to be used for Parkinson disease treatment (Yap et al., 2019). Although laminin 511 has been shown to support neurons in 2D (Zhang et al., 2017; Rodin et al., 2020; Zekonyte et al., 2016; Doi et al., 2020), 3D scaffolds using laminin 511 and 521 are not commonly examined for neural stem cell studies. Therefore, we have a gap in knowledge about neural stem cell-laminin 511 and 521 interaction in a mimicked niche that needs to be filled by designing new advanced scaffolds based on these proteins.
In addition to recombinant proteins, chemical peptide synthesis is available to generate binding sites and promote cell adhesion. Short laminin peptides are stable, easily synthesized, and target-specific (Li et al., 2014). Some of the laminin peptides that are used to interact with neural stem cells are listed in Table 3 [see review paper (Mukherjee et al., 2020), for more information]. These peptides are derived from different laminin chains, but there are few of them derived from laminin α5 chain of laminin 511 and laminin 521 that are the laminin of interest in the SVZ niche (AGQWHRVSVRWG and TWSQKALHHRVP). Huettner, Dargaville and Forget (2018) reports that 89% of biomaterials are functionalized with RGD, 6% with IKVAV and 4% with YIGSR, highlighting the need to extend studies on desirable peptides (derived from laminin α5, laminin β1, laminin β2, and laminin γ1) and discover more bioactive peptides to mimic the neural stem cell niche better.
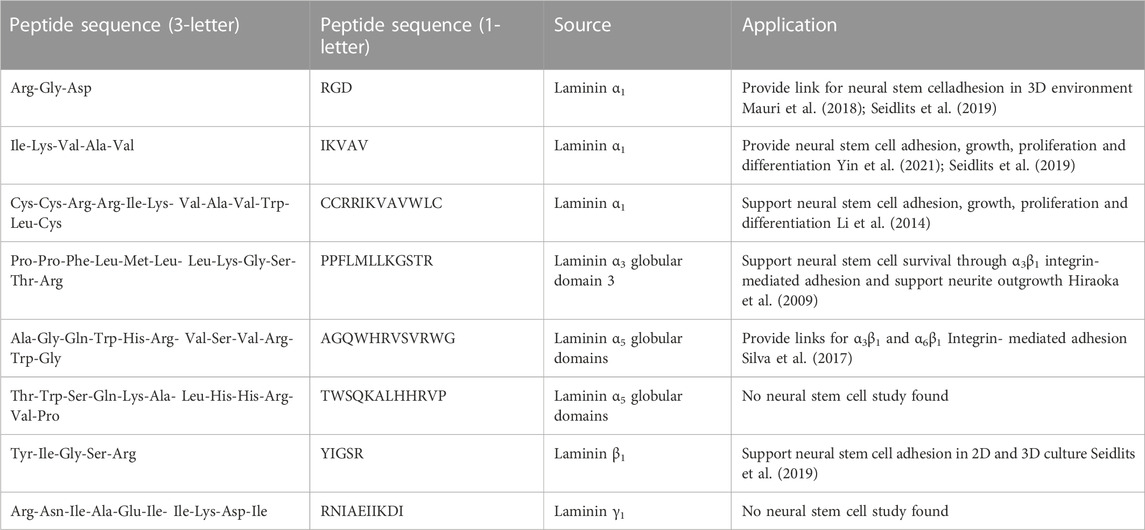
TABLE 3. List of laminin-derived peptides that are commonly used in mimicking neural stem cell niche.
4.2.2 Collagen
Despite its low abundance in adult SVZ, collagen is another ECM protein that can serve as a regulator for neural stem cell’s fate decisions. Since collagen I and collagen IV are the collagen types available in fractones and basement membranes, they could be studied to mimic the neural stem cell niche. In contrast with laminin, collagen is used both as a 3D structure backbone and as the added bioactive component. However, like laminin, there are natural, recombinant and synthetic versions of both collagen I and collagen IV. The whole-length animal-derived collagen I is the most common type of collagen to be used for in vitro models. However, Vagaska et al. (2020) found that collagen I alone is not sufficient for human neural stem cells to adhere and survive. They suggested that human neural stem cells do not express collagen I binding subunits, so other factors present in basement membranes including laminin, collagen IV, nidogen and perlecan are possibly required for human neural stem cells adhesion, survival and proliferation (Vagaska et al., 2020). In this regard, Bergström et al. (2014) confirmed that mRNA pattern encoding collagen I-binding integrins differs from postnatal to adulthood, especially integrin α1, α2 and integrin α11 and that is why adult human-derived neural stem cells cannot adhere to the collagen I-treated substrates while postnatal ones can. In studying the vascular basement membrane protein assembly for the neurogenic niche, Kim et al. (2021) illustrated that progenitor cells were less likely to adhere to collagen IV-overlaid laminin substrates versus laminin-overlaid collagen IV, suggesting the importance of laminin recognition within the niche. In pathological conditions, investigating laminin and collagen IV organization could be something interesting to study; however, there are still very limited studies on the effect of collagen IV on neural stem cell behavior. Although collagen does not seem to provide adherence sites for neural stem cells, understanding its effect on neural stem cell fate in concert with other matrix proteins or properties would give valuable information about its regulating role. Que et al. (2018) developed a recombinant collagen III scaffold decorated with integrin-binding peptide sequence from collagen I (GFOGER [Gly-Phe-Hyp-Gly-Glu-Arg]) and from laminin α1 (IKVAV [Ile-Lys-Val-Ala-Val]). They found that α1β1 integrin-mediated adhesion of fetal-derived neural stem/progenitor cells to the GFOGER peptide supported cell proliferation and differentiation toward both glial and neuronal lineages, but it was not clear if it works with adult-derived neural stem cells whose collagen binding receptors are not expressed through adulthood. Examining materials and proteins via high-throughput studies may provide further insight into the effects of interactions of the ECM with aging neural stem cells.
4.2.3 Heparan sulfate proteoglycans (HSPGs)
Added to laminin and collagen, HSPGs are considered as influential factors present in SVZ niche that can interact with other ECM proteins and contribute to neural stem cell regulation [for further information, see review by (Yu et al.t, 2017)]. HSPGs have been widely incorporated to biomaterials by taking advantage of high affinity between heparin and growth factor for tissue repairs (Sakiyama-Elbert, 2014). For example, Sakiyama-Elbert group found that delivery systems incorporated with the heparin and nerve growth factors help nerve regeneration (Wood et al., 2010). Perlecan and agrin are HSPGs present in both fractones and basement membranes that serve as mediators between laminin, collagen IV, growth factors and cell receptors. Therefore, in culture, HSPGs are used in combination with either laminin, collagen or growth factors. The combination of heparan sulfate with collagen as a scaffold for neural stem cells was used to investigate its effect on nerve regeneration and restoration after traumatic brain injury (Zhang and Wang, 2021). They found better neuronal recovery in the groups loaded with neural stem cells and suggested that the interaction between neural stem cells, collagen, heparan sulfate and FGF acted as anti-fibrosis and anti-inflammation inhibiting astrocyte activation and promoting neuronal differentiation (Zhang and Wang, 2021). A key role of heparan sulfate was in the stabilization of FGF2-FGF receptor complex to regulate neural stem cell proliferation and differentiation through activation of phosphorylation of extracellular regulated kinase 1 and 2 (pERK1/2) signaling (Latchoumane et al., 2022). Heparan sulfate offers great potential in biomaterials to mimic interactions with both ECM and growth factors within the neurogenic niche and improve neural stem cell response. Future work to expand our knowledge about the interplay of how these interactions affect neural stem cell fate will be critical to better understanding engineering design considerations to mimic the niche.
4.2.4 Residing cells
The final factor to discuss that adds bioactivity to these material scaffolds are cells from the SVZ, in addition to neural stem cells. In this regard, the most studied cells are endothelial cells, given the vascular changes that occur with age and pathology in the SVZ niche. Sosa et al. (2007) found that endothelial cells influence neural progenitor cells differently while contacting them directly or indirectly. Through indirect contact, an endothelial cell’s secreted factors (e.g., VEGF) helped support neural progenitor cell maintenance and proliferation, while direct contact led to neuronal differentiation (Sosa et al., 2007; Sun et al., 2010). Winkelman et al. (2021) reviewed the literature on neural stem cell and endothelial cell interactions and discussed that Ephrin-B2 and Jagged1 molecules on endothelial cells bind to Eph and Notch receptors located on neural stem cells to inhibit their proliferation. Another example of providing niche residing cells to neural stem cells is the co-culture of microglia and neural stem cells positively affecting neural stem cell differentiation toward dopaminergic neurons, which is the potential therapeutic for Parkinson’s disease treatment (Schmidt et al., 2021); co-culture with indirect contact provided higher yield of TH+ neurons possibly because of secreted factors (TNFa, IL-1b, and IGF1). However, they mentioned that different microglia cell lines may result in differentiation toward different neuronal subtypes like β-tubulin III+ neurons that should be considered (Schmidt et al., 2021) as future design considerations.
5 Current technologies
As discussed above, current knowledge about the brain organ’s structure and function has been used in order to develop models for treatment of neurodegenerative diseases. Pre-clinical animal models remain the standard, but cell-oriented in vitro models are currently attracting the engineer’s attention to better design and refine ex vivo. However, recreating in vitro cellular structures and biochemical factors in the exact order as the natural organ during development is the current challenge (Zhuang et al., 2018). Engineers use several methods to overcome these challenges, including but not limited to, brain organoids, 3D bioprinting, and microfluidics. Organoids are 3D complexes containing a variety of cells resembling the organ’s cellular composition, structure and function closely. In organoids, the self-organization of pluripotent stem cells/adult stem cells through cell sorting and differentiation leads to recreation of higher-order structures that replicate aspects of organs. As an example, organoids are used widely as an alternative model to AD animal models since the transgenic mice models can only recapitulate familial AD while the 97% of AD patients suffers from sporadic AD [refer to review paper (Kang and Cho, 2021)]. The culture conditions are important in organoid formation, where floating culture conditions are preferred over adherent conditions where Matrigel usually plays an important role (Lancaster et al., 2013). Currently, organoids are considered key models for understanding brain development since they can recapitulate some fundamental features of brain native tissue; however, forming organoids with consistent size and shape, without Matrigel because of its batch-to-batch variability, and with the potential of vascularization to prevent necrosis remain significant challenges. In this regard, readers are referred to review paper written by Li et al. (2023) summarizing the recent advances to develop vascularized neural organoids.
Three-dimensional bioprinting is another advanced method that provides the opportunity to form the complex tissue structures through layer-by-layer deposition of bioink that can incorporate materials, cells, and bioactive components. Bioprinting has the potential to create heterogenous tissue models with great consistency through fairly precise cell arrangement. For detailed review, readers are encouraged to read (Cadena et al., 2021). In addition to 3D bioprinting models, microfluidic platforms are widely used by manipulating the fluid flow to provide precise control over the microenvironment including the perfusion flow, cellular co-culture, insoluble factor organization, soluble factor gradients, etc. Microfluidic technologies provide unique brain microenvironment models, especially to study neuronal circuits, blood-brain barriers, and neurovascular unit models, since they provide control over the chemical gradients available in natural niche to better mimic the in-vivo dynamic environment. Holloway et al. (2021) has prepared a full review on microfluidic advances for neurological disease modeling. All of these technologies are new paths toward mimicking the brain microenvironment more effectively and precisely in vitro to understand the mechanisms behind disease onset.
6 Future perspectives
As engineers, we aim to develop platforms to examine the complex tissue niche to provide solutions for repair in the clinic. Current techniques are limited to mimic simpler microenvironmental aspects, not fully complex ones, integrating limited factors and replicating uncomplicated tissue architecture. In other words, many of these studies use a deconstructionist view to determine which factors may be important. Although these simplified systems are needed to indicate the interactions of different factors with the neural stem cells and understand their effects on neural stem cell fate, more complicated platforms decoupling the structural and bioactive characterizations of SVZ niche are still necessary to be developed for in vitro studies. For example, designing advanced tissue models that mimic the SVZ niche with its microvascular networks and CSF flow requires meeting all structural conditions (e.g., stiffness and degradability) with suitable micropatterns and bioactive components and with the capability to be used under microfluidic flow. Designing such complex systems still remains a challenge; however, as technologies advance, we will have increased potential to examine physiologically relevant interactions ex vivo.
The choice of bioactive components needs to be taken into careful consideration while designing hydrogels. The more they match the ones available in natural microenvironment, the more accurate information we acquire about the neural stem cell fate decision in healthy and pathological conditions. For example, laminin 111 purified from EHS mouse sarcoma cells is the most common laminin incorporated into 3D hydrogels for neural stem cell studies. However, it is only expressed in the CNS during embryogenesis and disappears from basement membranes during development. Therefore, it is not a good ECM representative when studying the aging and neurodegenerative diseases. Instead, laminin isoforms containing α5 chains including laminin 511 and laminin 521 are the laminins found in the adult niche and are appropriate candidates for studying neural stem cell’s change of behavior during adulthood. Specifically, the lack of knowledge about the effect laminin 511/521 (either full-length, recombinant, or peptides) in 3D platforms needs to be addressed. For this purpose, generating new laminin peptides derived from laminin 511 and laminin 521, which can interact effectively with neural stem cells, could be something to focus on for future studies.
The laminins are not the only part of the matrix environment that needs further consideration. The role of collagen I or collagen IV in fate decisions is not still clear, so more studies are needed to investigate if there are any structural changes and if their role alters while aging. These will provide more insight into the reasons why neurodegeneration initiates and progresses. We have to underscore the importance of HSPG use in the scaffolds designed to study neural stem cell behavior because of their critical role as a mediator in the SVZ niche interacting with ECM proteins, growth factors, and cell surface receptors. Determining the effect of each of these bioactive factors on the interactions between stem cells and their microenvironment (upstream signalling pathways) will provide more insight into identifying the activated downstream pathways leading to different cell decisions. Finally, decoupling the mechanical properties of a scaffold from bioactive factors and controlled degradation will highlight the effects of mechanotransduction on neural stem cell fate, which is not still clear. Balancing the complexity of the niche with well controlled and repeatable studies will be critical to fully understanding the neural stem cell niche ex vivo and being able to appropriately design solutions to pathological conditions.
7 Conclusion
Overall, this review paper provides a general overview of the SVZ niche, one of the two main regions neural stem cells reside. It highlights the important features of the niche that might be interesting for engineers to take into consideration while designing hydrogel-based scaffolds. These features include the cellular components versus the effective ECM proteins, proteoglycans, and growth factors. In the biomaterial section, we go into more detail about the requirements that scaffolds are required to meet to be considered as a potential option for studying neural stem cell behavior. In this regard, there are structural and bioactive features that need to be applied to the materials. It is hard to maintain the complexity of the natural microenvironment without compromising the important features for each application, either in vitro or for solutions for in vivo. Although there are advances in designing the scaffolds during the past few years to meet the characteristics of the SVZ niche, there is still a gap between where the ideal scaffolds could be and where they are now. Therefore, more efforts are needed to design the ideal hydrogel-based scaffolds combining the niche critical factors to study their interaction with neural stem cells and their effect on fate decisions.
Author contributions
Research conception and design: NY and RKW. Review of published data in the literature: NY and RKW. Interpretation of data published in the literature: NY and RKW. Preparation of figures: NY. Drafting of the manuscript: NY. Editing and revising the manuscript: NY and RKW. Approval of the final version of the manuscript: NY and RKW.
Funding
Authors acknowledge that this work was funded by National Science Foundation under NSF award number 2113403 awarded to RKW.
Acknowledgments
We thank McKay Cavanaugh for her final proofreading.
Conflict of interest
The authors declare that the research was conducted in the absence of any commercial or financial relationships that could be construed as a potential conflict of interest.
Publisher’s note
All claims expressed in this article are solely those of the authors and do not necessarily represent those of their affiliated organizations, or those of the publisher, the editors and the reviewers. Any product that may be evaluated in this article, or claim that may be made by its manufacturer, is not guaranteed or endorsed by the publisher.
References
Addington, C. P., Heffernan, J., Millar-Haskell, C., Tucker, E., Sirianni, R., and Stabenfeldt, S. (2015). Enhancing neural stem cell response to SDF-1α gradients through hyaluronic acid-laminin hydrogels. Biomaterials 72, 11–19. doi:10.1016/j.biomaterials.2015.08.041
Ahn, S., and Joyner, A. L. (2005). In vivo analysis of quiescent adult neural stem cells responding to Sonic hedgehog. Nature 437 (7060), 894–897. doi:10.1038/nature03994
Altman, J. (1962). Are new neurons formed in the brains of adult mammals? Sci. (New York, N.Y.) 135 (3509), 1127–1128. doi:10.1126/science.135.3509.1127
Amores de Sousa, M. C., Rodrigues, C. A. V., Ferreira, I. A. F., Diogo, M. M., Linhardt, R. J., Cabral, J. M. S., et al. (2020). Functionalization of electrospun nanofibers and fiber alignment enhance neural stem cell proliferation and neuronal differentiation. Front. Bioeng. Biotechnol. 8, 580135–580216. doi:10.3389/fbioe.2020.580135
Andreu-Agulló, C., Morante-Redolat, J. M., Delgado, A. C., and Farinas, I. (2009). Vascular niche factor PEDF modulates notch-dependent stemness in the adult subependymal zone. Nat. Neurosci. 12 (12), 1514–1523. doi:10.1038/nn.2437
Barros, D., Amaral, I. F., and Pêgo, A. P. (2020). Laminin-inspired cell-instructive microenvironments for neural stem cells. Biomacromolecules 21 (2), 276–293. doi:10.1021/acs.biomac.9b01319
Barros, D., Conde-Sousa, E., Goncalves, A. M., Han, W. M., Garcia, A. J., Amaral, I. F., et al. (2019). Engineering hydrogels with affinity-bound laminin as 3D neural stem cell culture systems. Biomaterials Sci. 7 (12), 5338–5349. doi:10.1039/c9bm00348g
Bergström, T., Holmqvist, K., Tararuk, T., Johansson, S., and Forsberg-Nilsson, K. (2014). Developmentally regulated collagen/integrin interactions confer adhesive properties to early postnatal neural stem cells. Biochimica Biophysica Acta - General Subj. 1840 (8), 2526–2532. doi:10.1016/j.bbagen.2014.01.021
Bible, E., Chau, D. Y., Alexander, M. R., Price, J., Shakesheff, K. M., and Modo, M. (2009). The support of neural stem cells transplanted into stroke-induced brain cavities by PLGA particles. Biomaterials 30 (16), 2985–2994. doi:10.1016/j.biomaterials.2009.02.012
Bjornsson, C. S., Apostolopoulou, M., Tian, Y., and Temple, S. (2015). It takes a village: Constructing the neurogenic niche. Dev. Cell. 32 (4), 435–446. doi:10.1016/j.devcel.2015.01.010
Bjugstad, K. B., Lampe, K., Kern, D. S., and Mahoney, M. (2010). Biocompatibility of poly(ethylene glycol)-based hydrogels in the brain: An analysis of the glial response across space and time. J. Biomed. Mater. Res. - Part A 95 (1), 79–91. doi:10.1002/jbm.a.32809
Blokland, K. E. C., Pouwels, S., Schuliga, M., Knight, D., and Burgess, J. (2020). Regulation of cellular senescence by extracellular matrix during chronic fibrotic diseases. Clin. Sci. 134 (20), 2681–2706. doi:10.1042/CS20190893
Boccafoschi, F., Botta, M., Fusaro, L., Copes, F., Ramella, M., and Cannas, M. (2017). Decellularized biological matrices: An interesting approach for cardiovascular tissue repair and regeneration. J. Tissue Eng. Regen. Med. 11 (5), 1648–1657. doi:10.1002/term.2103
Bohrer, C., Pfurr, S., Mammadzada, K., Schildge, S., Plappert, L., Hils, M., et al. (2015). The balance of Id3 and E47 determines neural stem/precursor cell differentiation into astrocytes. EMBO J. 34 (22), 2804–2819. doi:10.15252/embj.201591118
Boni, R., Ali, A., Shavandi, A., and Clarkson, A. N. (2018). Current and novel polymeric biomaterials for neural tissue engineering. J. Biomed. Sci. 25 (1), 90–21. doi:10.1186/s12929-018-0491-8
Brooker, S. M., Bond, A. M., Peng, C. Y., and Kessler, J. A. (2016). β1-Integrin restricts astrocytic differentiation of adult hippocampal neural stem cells. Glia 64 (7), 1235–1251. doi:10.1002/glia.22996
Budday, S., Sommer, G., Birkl, C., Langkammer, C., Haybaeck, J., Kohnert, J., et al. (2017). Mechanical characterization of human brain tissue. Acta Biomater. 48, 319–340. doi:10.1016/j.actbio.2016.10.036
Cadena, M., Ning, L., King, A., Hwang, B., Jin, L., Serpooshan, V., et al. (2021). 3D bioprinting of neural tissues. Adv. Healthc. Mater. 10 (15), 2001600. doi:10.1002/adhm.202001600
Caliari, S. R., and Burdick, J. A. (2016). A practical guide to hydrogels for cell culture. Nat. Methods 13 (5), 405–414. doi:10.1038/nmeth.3839
Capilla-Gonzalez, V., Cebrian-Silla, A., Guerrero-Cazares, H., Garcia-Verdugo, J. M., and Quinones-Hinojosa, A. (2013). The generation of oligodendroglial cells is preserved in the rostral migratory stream during aging. Front. Cell. Neurosci. 7, 147–149. doi:10.3389/fncel.2013.00147
Capilla-Gonzalez, V., Herranz-Pérez, V., and García-Verdugo, J. M. (2015). The aged brain: Genesis and fate of residual progenitor cells in the subventricular zone. Front. Cell. Neurosci. 9, 365–411. doi:10.3389/fncel.2015.00365
Cavanaugh, M., Silantyeva, E., Pylypiv Koh, G., Malekzadeh, E., Lanzinger, W. D., Willits, R. K., et al. (2019). RGD-modified nanofibers enhance outcomes in rats after sciatic nerve injury. J. Funct. Biomaterials 10 (2), 24. doi:10.3390/jfb10020024
Chai, Y., Long, Y., Dong, X., Liu, K., Wei, W., Chen, Y., et al. (2022). Improved functional recovery of rat transected spinal cord by peptide-grafted PNIPAM based hydrogel. Colloids Surfaces B Biointerfaces 210, 112220. doi:10.1016/j.colsurfb.2021.112220
Chandy, T. (2022). Nerve tissue engineering on degradable scaffold’, tissue engineering: Current Status and challenges, 363–398. doi:10.1016/B978-0-12-824064-9.00011-3
Chavali, M., Klingener, M., Kokkosis, A. G., Garkun, Y., Felong, S., Maffei, A., et al. (2018). Non-canonical Wnt signaling regulates neural stem cell quiescence during homeostasis and after demyelination. Nat. Commun. 9 (1), 36–17. doi:10.1038/s41467-017-02440-0
Christopherson, G. T., Song, H., and Mao, H. Q. (2009). The influence of fiber diameter of electrospun substrates on neural stem cell differentiation and proliferation. Biomaterials 30 (4), 556–564. doi:10.1016/j.biomaterials.2008.10.004
Conover, J. C., and Todd, K. L. (2017). Development and aging of a brain neural stem cell niche. Exp. Gerontol. 94, 9–13. doi:10.1016/j.exger.2016.11.007
Coronel, M. M., Martin, K. E., Hunckler, M. D., Kalelkar, P., Shah, R. M., and Garcia, A. J. (2022). Hydrolytically degradable microgels with tunable mechanical properties modulate the host immune response. Small 18 (36), 2106896. doi:10.1002/smll.202106896
Cutler, R. R., and Kokovay, E. (2020). Rejuvenating subventricular zone neurogenesis in the aging brain. Curr. Opin. Pharmacol. 50, 1–8. doi:10.1016/j.coph.2019.10.005
Daly, A. C., Riley, L., Segura, T., and Burdick, J. A. (2020). Hydrogel microparticles for biomedical applications. Nat. Rev. Mater. 5 (1), 20–43. doi:10.1038/s41578-019-0148-6
Daynac, M., Morizur, L., Chicheportiche, A., Mouthon, M. A., and Boussin, F. D. (2016). Age-related neurogenesis decline in the subventricular zone is associated with specific cell cycle regulation changes in activated neural stem cells. Sci. Rep. 6, 21505–21510. doi:10.1038/srep21505
Delgado, A. C., Ferron, S., Vicente, D., Porlan, E., Perez-Villalba, A., Trujillo, C., et al. (2014). Endothelial NT-3 delivered by vasculature and CSF promotes quiescence of subependymal neural stem cells through nitric oxide induction. Neuron 83 (3), 572–585. doi:10.1016/j.neuron.2014.06.015
Ding, W. Y., Huang, J., and Wang, H. (2020). Waking up quiescent neural stem cells: Molecular mechanisms and implications in neurodevelopmental disorders. PLoS Genet. 16 (4), 10086533–e1008726. doi:10.1371/journal.pgen.1008653
Distler, T., Lauria, I., Detsch, R., Sauter, C. M., Bendt, F., Kapr, J., et al. (2021). Neuronal differentiation from induced pluripotent stem cell-derived neurospheres by the application of oxidized alginate-gelatin-laminin hydrogels. Biomedicines 9 (3), 261. doi:10.3390/biomedicines9030261
Doblado, L. R., Martínez-Ramos, C., and Pradas, M. M. (2021). Biomaterials for neural tissue engineering. Front. Nanotechnol. 3. doi:10.3389/fnano.2021.643507
Doi, D., Magotani, H., Kikuchi, T., Ikeda, M., Hiramatsu, S., Yoshida, K., et al. (2020). Pre-clinical study of induced pluripotent stem cell-derived dopaminergic progenitor cells for Parkinson’s disease. Nat. Commun. 11 (1), 3369. doi:10.1038/s41467-020-17165-w
Emsley, J. G., and Hagg, T. (2003). α6β1 integrin directs migration of neuronal precursors in adult mouse forebrain. Exp. Neurol. 183 (2), 273–285. doi:10.1016/S0014-4886(03)00209-7
Fernández, D., Guerra, M., Lisoni, J. G., Hoffmann, T., Araya-Hermosilla, R., Shibue, T., et al. (2019). Fibrous materials made of poly(ε-caprolactone)/poly(ethylene oxide)-b-Poly(ε-caprolactone) blends support neural stem cells differentiation. Polymers 11 (10), 1621. doi:10.3390/polym11101621
Fon, D., Al-Abboodi, A., Chan, P. P. Y., Zhou, K., Crack, P., Finkelstein, D. I., et al. (2014). Effects of GDNF-loaded injectable gelatin-based hydrogels on endogenous neural progenitor cell migration. Adv. Healthc. Mater. 3 (5), 761–774. doi:10.1002/adhm.201300287
Fujioka, T., Kaneko, N., Ajioka, I., Nakaguchi, K., Omata, T., Ohba, H., et al. (2017). β1 integrin signaling promotes neuronal migration along vascular scaffolds in the post-stroke brain. EBioMedicine 16, 195–203. doi:10.1016/j.ebiom.2017.01.005
Fujioka, T., Kaneko, N., and Sawamoto, K. (2019). Blood vessels as a scaffold for neuronal migration. Neurochem. Int. 126, 69–73. doi:10.1016/j.neuint.2019.03.001
Galderisi, U., Peluso, G., Di Bernardo, G., Calarco, A., D'Apolito, M., Petillo, O., et al. (2013). Efficient cultivation of neural stem cells with controlled delivery of FGF-2. Stem Cell. Res. 10 (1), 85–94. doi:10.1016/j.scr.2012.09.001
George, J., Hsu, C. C., Nguyen, L. T. B., Ye, H., and Cui, Z. (2020). Neural tissue engineering with structured hydrogels in CNS models and therapies. Biotechnol. Adv. 42, 107370. doi:10.1016/j.biotechadv.2019.03.009
Gómez-Gaviro, M. V., Scott, C. E., Sesay, A. K., Matheu, A., Booth, S., Galichet, C., et al. (2012). Betacellulin promotes cell proliferation in the neural stem cell niche and stimulates neurogenesis. Proc. Natl. Acad. Sci. U. S. A. 109 (4), 1317–1322. doi:10.1073/pnas.1016199109
Gonzalez-Perez, O., and Alvarez-Buylla, A. (2011). Oligodendrogenesis in the subventricular zone and the role of epidermal growth factor. Brain Res. Rev. 67 (1–2), 147–156. doi:10.1016/j.brainresrev.2011.01.001
Hall, P. E., Lathia, J. D., Miller, N. G. A., Caldwell, M. A., and Ffrench-Constant, C. (2006). Integrins are markers of human neural stem cells. Stem Cells 24 (9), 2078–2084. doi:10.1634/stemcells.2005-0595
Ham, T. R., Pukale, D. D., Hamrangsekachaee, M., and Leipzig, N. D. (2020). Subcutaneous priming of protein-functionalized chitosan scaffolds improves function following spinal cord injury. Mater. Sci. Eng. C 110, 110656. doi:10.1016/j.msec.2020.110656
Hamrangsekachaee, M., Baumann, H. J., Pukale, D. D., Shriver, L. P., and Leipzig, N. D. (2022). Investigating mechanisms of subcutaneous preconditioning incubation for neural stem cell embedded hydrogels. ACS Appl. Bio Mater. 5, 2176–2184. doi:10.1021/acsabm.2c00017
Han, J., Calvo, C. F., Kang, T., Baker, K., Park, J. H., Parras, C., et al. (2015). Vascular endothelial growth factor receptor 3 controls neural stem cell activation in mice and humans. Cell. Rep. 10 (7), 1158–1172. doi:10.1016/j.celrep.2015.01.049
Hiraoka, M., Kato, K., Nakaji-Hirabayashi, T., and Iwata, H. (2009). Enhanced survival of neural cells embedded in hydrogels composed of collagen and laminin-derived cell adhesive peptide. Bioconjugate Chem. 20 (5), 976–983. doi:10.1021/bc9000068
Hiscox, L. V., Johnson, C. L., Barnhill, E., McGarry, M. D. J., Huston, J., van Beek, E. J. R., et al. (2016). Magnetic resonance elastography (MRE) of the human brain: Technique, findings and clinical applications. Phys. Med. Biol. 61 (24), R401–R437. doi:10.1088/0031-9155/61/24/R401
Hodge, R. D., Joseph D’Ercole, A., and O’Kusky, J. R. (2004). Insulin-like growth factor-I accelerates the cell cycle by decreasing G1 phase length and increases cell cycle reentry in the embryonic cerebral cortex. J. Neurosci. 24 (45), 10201–10210. doi:10.1523/JNEUROSCI.3246-04.2004
Holloway, P. M., Willaime-Morawek, S., Siow, R., Barber, M., Owens, R. M., Sharma, A. D., et al. (2021). Advances in microfluidic in vitro systems for neurological disease modeling. J. Neurosci. Res. 99 (5), 1276–1307. doi:10.1002/jnr.24794
Hsieh, F. Y., Lin, H. H., and Hsu, S. H. (2015). 3D bioprinting of neural stem cell-laden thermoresponsive biodegradable polyurethane hydrogel and potential in central nervous system repair. Biomaterials 71, 48–57. doi:10.1016/j.biomaterials.2015.08.028
Hsieh, J. (2012). Orchestrating transcriptional control of adult neurogenesis. Genes. Dev. 26 (10), 1010–1021. doi:10.1101/gad.187336.112
Huettner, N., Dargaville, T. R., and Forget, A. (2018). Discovering cell-adhesion peptides in tissue engineering: Beyond RGD. Trends Biotechnol. 36 (4), 372–383. doi:10.1016/j.tibtech.2018.01.008
Humphries, J. D., Byron, A., and Humphries, M. J. (2006). Integrin ligands at a glance. J. Cell. Sci. 119 (19), 3901–3903. doi:10.1242/jcs.03098
Hwang, D. H., Lee, H. J., Park, I. H., Seok, J. I., Kim, B. G., Joo, I. S., et al. (2009). Intrathecal transplantation of human neural stem cells overexpressing VEGF provide behavioral improvement, disease onset delay and survival extension in transgenic ALS mice. Gene Ther. 16 (10), 1234–1244. doi:10.1038/gt.2009.80
Hynes, R. O. (2009). The extracellular matrix: Not just pretty fibrils. Science 326 (5957), 1216–1219. doi:10.1126/science.1176009
Isomursu, A., Lerche, M., Taskinen, M. E., Ivaska, J., and Peuhu, E. (2019). Integrin signaling and mechanotransduction in regulation of somatic stem cells. Exp. Cell. Res. 378 (2), 217–225. doi:10.1016/j.yexcr.2019.01.027
Jackson, E. L., and Alvarez-Buylla, A. (2008). Characterization of adult neural stem cells and their relation to brain tumors. Cells Tissues Organs 188 (1–2), 212–224. doi:10.1159/000114541
Jorfi, M., D’Avanzo, C., Tanzi, R. E., Kim, D. Y., and Irimia, D. (2018). Human neurospheroid arrays for in vitro studies of alzheimer’s disease. Sci. Rep. 8 (1), 2450–2513. doi:10.1038/s41598-018-20436-8
Kamatar, A., Gunay, G., and Acar, H. (2020). Natural and synthetic biomaterials for engineering multicellular tumor spheroids. Polymers 12 (11), 2506–2523. doi:10.3390/polym12112506
Kang, Y. J., and Cho, H. (2021). Human brain organoids in Alzheimer’s disease. Organoid 1, e5. doi:10.51335/organoid.2021.1.e5
Karakatsani, A., Shah, B., and Ruiz de Almodovar, C. (2019). Blood vessels as regulators of neural stem cell properties. Front. Mol. Neurosci. 12, 85–11. doi:10.3389/fnmol.2019.00085
Kazanis, I., Lathia, J. D., Vadakkan, T. J., Raborn, E., Wan, R., Mughal, M. R., et al. (2010). Quiescence and activation of stem and precursor cell populations in the subependymal zone of the mammalian brain are associated with distinct cellular and extracellular matrix signals. J. Neurosci. 30 (29), 9771–9781. doi:10.1523/JNEUROSCI.0700-10.2010
Kerever, A., Nagahara, F., Keino-Masu, K., Masu, M., van Kuppevelt, T. H., Vives, R. R., et al. (2021). Regulation of fractone heparan sulfate composition in young and aged subventricular zone neurogenic niches. Glycobiology 31 (11), 1531–1542. doi:10.1093/glycob/cwab081
Khaing, Z. Z., and Seidlits, S. K. (2015). Hyaluronic acid and neural stem cells: Implications for biomaterial design. J. Mater. Chem. B 3 (40), 7850–7866. doi:10.1039/c5tb00974j
Kim, H. J., Lee, E., Nam, M., Chung, J. K., Joo, S., Nam, Y., et al. (2021). Contribution of extracellular matrix component landscapes in the adult subventricular zone to the positioning of neural stem/progenitor cells. Exp. Neurobiol. 30 (4), 275–284. doi:10.5607/EN21012
Kjell, J., Fischer-Sternjak, J., Thompson, A. J., Friess, C., Sticco, M. J., Salinas, F., et al. (2020). Defining the adult neural stem cell niche proteome identifies key regulators of adult neurogenesis. Cell. Stem Cell. 26 (2), 277–293.e8. e8. doi:10.1016/j.stem.2020.01.002
Lancaster, M. A., Renner, M., Martin, C. A., Wenzel, D., Bicknell, L. S., Hurles, M. E., et al. (2013). Cerebral organoids model human brain development and microcephaly. Nature 501 (7467), 373–379. doi:10.1038/nature12517
Larripa, K., and Gallegos, A. (2017). A mathematical model of Noggin and BMP densities in adult neural stem cells. Lett. Biomathematics 4 (1), 219–243. doi:10.30707/lib4.1larripa
Latchoumane, C.-F. V., Chopra, P., Sun, L., Ahmed, A., Palmieri, F., Wu, H. F., et al. (2022). Synthetic heparan sulfate hydrogels regulate neurotrophic factor signaling and neuronal network activity. ACS Appl. Mater. Interfaces 14, 28476–28488. doi:10.1021/acsami.2c01575
Lee, B., Clarke, D., Al Ahmad, A., Kahle, M., Parham, C., Auckland, L., et al. (2011). Perlecan domain V is neuroprotective and proangiogenic following ischemic stroke in rodents. pdf’ 121 (8), 3005–3023. doi:10.1172/jci46358
Leipzig, N. D., and Shoichet, M. S. (2009). The effect of substrate stiffness on adult neural stem cell behavior. Biomaterials 30 (36), 6867–6878. doi:10.1016/j.biomaterials.2009.09.002
Lévesque, S. G., and Shoichet, M. S. (2007). Synthesis of enzyme-degradable, peptide-cross-linked dextran hydrogels. Bioconjugate Chem. 18 (3), 874–885. doi:10.1021/bc0602127
Li, M., Gao, L., Zhao, L., Zou, T., Xu, H., et al. (2023). Toward the next generation of vascularized human neural organoids. Med. Res. Rev., 31–54. doi:10.1002/med.21922
Li, X., Liu, X., Josey, B., Chou, C. J., Tan, Y., Zhang, N., et al. (2014). Short laminin peptide for improved neural stem cell growth. Stem Cells Transl. Med. 3 (5), 662–670. doi:10.5966/sctm.2013-0015
Li, Y., et al. (2015). “Fibrin gel as an injectable biodegradable scaffold and cell carrier for tissue engineering,” in The scientific world journal (R. Adhikari), 685690. doi:10.1155/2015/685690
Licht, T., Rothe, G., Kreisel, T., Wolf, B., Benny, O., Rooney, A. G., et al. (2016). VEGF preconditioning leads to stem cell remodeling and attenuates age-related decay of adult hippocampal neurogenesis. Proc. Natl. Acad. Sci. U. S. A. 113 (48), E7828-E7836. –E7836. doi:10.1073/pnas.1609592113
Lim, D., and Alvarez-Buylla, A. (2016). The adult ventricular–subventricular zone (V-svz) and olfactory bulb (OB) neurogenesis. Cold Spring Harb. Perspect. Biol. 8 (5), a018820. Available at:. doi:10.1101/cshperspect.a018820
Lim, D. A., Tramontin, A. D., Trevejo, J. M., Herrera, D. G., Garcia-Verdugo, J. M., and Alvarez-Buylla, A. (2000). Noggin antagonizes BMP signaling to create a niche for adult neurogenesis. Neuron 28 (3), 713–726. doi:10.1016/s0896-6273(00)00148-3
Liu, S., et al. (2022) ‘Three-dimensional bioprinting sodium alginate/gelatin scaffold combined with neural stem cells and oligodendrocytes markedly promoting nerve regeneration after spinal cord injury. Regenerative Biomaterials 9, rbac038. doi:10.1093/rb/rbac038
Liu, W., Ye, P., O'Kusky, J. R., and D'Ercole, A. J. (2009). Type 1 insulin-like growth factor receptor signaling is essential for the development of the hippocampal formation and dentate gyrus. J. Neurosci. Res. 87 (13), 2821–2832. doi:10.1002/jnr.22129
Lledo, P. M., Merkle, F. T., and Alvarez-Buylla, A. (2008). Origin and function of olfactory bulb interneuron diversity. Trends Neurosci. 31 (8), 392–400. doi:10.1016/j.tins.2008.05.006
Lutolf, M. P., Lauer-Fields, J. L., Schmoekel, H. G., Metters, A. T., Weber, F. E., Fields, G. B., et al. (2003). Synthetic matrix metalloproteinase-sensitive hydrogels for the conduction of tissue regeneration: Engineering cell-invasion characteristics. Proc. Natl. Acad. Sci. U. S. A. 100 (9), 5413–5418. doi:10.1073/pnas.0737381100
Ma, D. K., Bonaguidi, M. A., Ming, G. l., and Song, H. (2009). Adult neural stem cells in the mammalian central nervous system. Cell. Res. 19 (6), 672–682. doi:10.1038/cr.2009.56
Ma, D. K., Ming, G. L., and Song, H. (2005). Glial influences on neural stem cell development: Cellular niches for adult neurogenesis. Curr. Opin. Neurobiol. 15, 514–520. doi:10.1016/j.conb.2005.08.003
Mahuzier, A., Shihavuddin, A., Fournier, C., Lansade, P., Faucourt, M., Menezes, N., et al. (2018). Ependymal cilia beating induces an actin network to protect centrioles against shear stress. Nat. Commun. 9 (1), 2279. doi:10.1038/s41467-018-04676-w
Malekzadeh, E., and Zhang Newby, B. M. (2020). Thermoresponsive poly(vinyl methyl ether) (PVME) retained by 3-aminopropyltriethoxysilane (APTES) network. ACS Biomater. Sci. Eng. 6 (12), 7051–7060. doi:10.1021/acsbiomaterials.0c01376
Manabe, R. I., Tsutsui, K., Yamada, T., Kimura, M., Nakano, I., Shimono, C., et al. (2008). Transcriptome-based systematic identification of extracellular matrix proteins. Proc. Natl. Acad. Sci. U. S. A. 105 (35), 12849–12854. doi:10.1073/pnas.0803640105
Mauri, E., Sacchetti, A., Vicario, N., Peruzzotti-Jametti, L., Rossi, F., and Pluchino, S. (2018). Evaluation of RGD functionalization in hybrid hydrogels as 3D neural stem cell culture systems. Biomaterials Sci. 6 (3), 501–510. doi:10.1039/c7bm01056g
Melrose, J. (2022). Fractone stem cell niche components provide intuitive clues in the design of new therapeutic procedures/biomatrices for neural repair. Int. J. Mol. Sci. 23 (9), 5148. doi:10.3390/ijms23095148
Mercier, F. (2016). Fractones: Extracellular matrix niche controlling stem cell fate and growth factor activity in the brain in health and disease. Cell. Mol. Life Sci. 73 (24), 4661–4674. doi:10.1007/s00018-016-2314-y
Mercier, F., Kitasako, J. T., and Hatton, G. I. (2002). Anatomy of the brain neurogenic zones revisited: Fractones and the fibroblast/macrophage network. J. Comp. Neurology 451 (2), 170–188. doi:10.1002/cne.10342
Mercier, F., Schnack, J., and Chaumet, M. S. G. (2011). “Fractones: Home and conductors of the neural stem cell niche,” in Neurogenesis in the adult brain I: Neurobiology. Editor T. Sekiet al. (Tokyo: Springer Japan), 109–133. doi:10.1007/978-4-431-53933-9_4
Merkle, F. T., Tramontin, A. D., Garcia-Verdugo, J. M., and Alvarez-Buylla, A. (2004). Radial glia give rise to adult neural stem cells in the subventricular zone. Proc. Natl. Acad. Sci. U. S. A. 101 (50), 17528–17532. doi:10.1073/pnas.0407893101
Mirzadeh, Z., Merkle, F. T., Soriano-Navarro, M., Garcia-Verdugo, J. M., and Alvarez-Buylla, A. (2008). Neural stem cells confer unique pinwheel architecture to the ventricular surface in neurogenic regions of the adult brain. Cell. Stem Cell. 3 (3), 265–278. doi:10.1016/j.stem.2008.07.004
Mishra, S., Kelly, K. K., Rumian, N. L., and Siegenthaler, J. A. (2018). Retinoic acid is required for neural stem and progenitor cell proliferation in the adult Hippocampus. Stem Cell. Rep. 10 (6), 1705–1720. doi:10.1016/j.stemcr.2018.04.024
Morell, M., Tsan, Y., and O’Shea, K. S. (2015). Inducible expression of noggin selectively expands neural progenitors in the adult SVZ. Stem Cell. Res. 14 (1), 79–94. doi:10.1016/j.scr.2014.11.001
Mori, H., and Hara, M. (2016). Clusters of neural stem/progenitor cells cultured on a soft poly(vinyl alcohol) hydrogel crosslinked by gamma irradiation. J. Biosci. Bioeng. 121 (5), 584–590. doi:10.1016/j.jbiosc.2015.09.010
Motta, C. M. M., Endres, K. J., Wesdemiotis, C., Willits, R. K., and Becker, M. L. (2019). Enhancing Schwann cell migration using concentration gradients of laminin-derived peptides. Biomaterials 218, 119335. doi:10.1016/j.biomaterials.2019.119335
Mouthon, M. A., Morizur, L., Dutour, L., Pineau, D., Kortulewski, T., and Boussin, F. D. (2020). Syndecan-1 stimulates adult neurogenesis in the mouse ventricular-subventricular zone after injury. J. Science 23 (12), 101784. doi:10.1016/j.isci.2020.101784
Mukherjee, N., Adak, A., and Ghosh, S. (2020). Recent trends in the development of peptide and protein-based hydrogel therapeutics for the healing of CNS injury. Soft Matter 16 (44), 10046–10064. doi:10.1039/d0sm00885k
Murphy, W. L., McDevitt, T. C., and Engler, A. J. (2014). Materials as stem cell regulators. Nat. Mater. 13 (6), 547–557. doi:10.1038/nmat3937
Nascimento, M. A., Sorokin, L., and Coelho-Sampaio, T. (2018). Fractone bulbs derive from ependymal cells and their laminin composition influence the stem cell niche in the subventricular zone. J. Neurosci. 38 (16), 3880–3889. doi:10.1523/JNEUROSCI.3064-17.2018
Nih, L. R., Sideris, E., Carmichael, S. T., and Segura, T. (2017). Injection of microporous annealing particle (MAP) hydrogels in the stroke cavity reduces gliosis and inflammation and promotes NPC migration to the lesion. Adv. Mater. Deerf. Beach, Fla.) 29 (32), 1606471. doi:10.1002/adma.201606471
Nishiuchi, R., Takagi, J., Hayashi, M., Ido, H., Yagi, Y., Sanzen, N., et al. (2006). Ligand-binding specificities of laminin-binding integrins: A comprehensive survey of laminin-integrin interactions using recombinant α3β1, α6β1, α7β1 and α6β4 integrins. Matrix Biol. 25 (3), 189–197. doi:10.1016/j.matbio.2005.12.001
Nottebohm, F. (1981). A brain for all seasons: Cyclical anatomical changes in song control nuclei of the canary brain. Science 214 (4527), 1368–1370. doi:10.1126/science.7313697
Nottebohm, F. (2004). The road we travelled: Discovery, choreography, and significance of brain replaceable neurons. Ann. N. Y. Acad. Sci. 1016, 628–658. doi:10.1196/annals.1298.027
O’Brien, F. J. (2011). Biomaterials & scaffolds for tissue engineering. Mater. Today 14 (3), 88–95. doi:10.1016/S1369-7021(11)70058-X
O’Donnell, J. C., Purvis, E. M., Helm, K. V. T., Adewole, D. O., Zhang, Q., et al. (2021). An implantable human stem cell-derived tissue-engineered rostral migratory stream for directed neuronal replacement. Commun. Biol. 4 (1), 879–916. doi:10.1038/s42003-021-02392-8
Oikari, L. E., Okolicsanyi, R. K., Qin, A., Yu, C., Griffiths, L. R., and Haupt, L. M. (2016). Cell surface heparan sulfate proteoglycans as novel markers of human neural stem cell fate determination. Stem Cell. Res. 16 (1), 92–104. doi:10.1016/j.scr.2015.12.011
Omiya, H., Yamaguchi, S., Watanabe, T., Kuniya, T., Harada, Y., Kawaguchi, D., et al. (2021). BMP signaling suppresses Gemc1 expression and ependymal differentiation of mouse telencephalic progenitors. Sci. Rep. 11 (1), 613–614. doi:10.1038/s41598-020-79610-6
Panchision, D. M., and McKay, R. D. G. (2002). The control of neural stem cells by morphogenic signals. Curr. Opin. Genet. Dev. 12 (4), 478–487. doi:10.1016/S0959-437X(02)00329-5
Pandamooz, S., Jafari, A., Salehi, M. S., Jurek, B., Ahmadiani, A., Safari, A., et al. (2020). Substrate stiffness affects the morphology and gene expression of epidermal neural crest stem cells in a short term culture. Biotechnol. Bioeng. 117 (2), 305–317. doi:10.1002/bit.27208
Papadimitriou, C., Celikkaya, H., Cosacak, M. I., Mashkaryan, V., Bray, L., Bhattarai, P., et al. (2018). 3D culture method for alzheimer’s disease modeling reveals interleukin-4 rescues aβ42-induced loss of human neural stem cell plasticity. Dev. Cell. 46 (1), 85–101.e8. e8. doi:10.1016/j.devcel.2018.06.005
Peressotti, S., Koehl, G. E., Goding, J. A., and Green, R. A. (2021). Self-assembling hydrogel structures for neural tissue repair. ACS Biomaterials Sci. Eng. 7 (9), 4136–4163. doi:10.1021/acsbiomaterials.1c00030
Pfau, M. R., and Grunlan, M. A. (2021). Smart scaffolds: Shape memory polymers (SMPs) in tissue engineering. J. Mater. Chem. B 9 (21), 4287–4297. doi:10.1039/d1tb00607j
Platel, J. C., and Bordey, A. (2016). The multifaceted subventricular zone astrocyte: From a metabolic and pro-neurogenic role to acting as a neural stem cell. Neuroscience 323, 20–28. doi:10.1016/j.neuroscience.2015.10.053
Que, R. A., Arulmoli, J., Da Silva, N. A., Flanagan, L. A., and Wang, S. (2018). Recombinant collagen scaffolds as substrates for human neural stem/progenitor cells. J. Biomed. Mater. Res. - Part A 106 (5), 1363–1372. doi:10.1002/jbm.a.36343
Reynolds, B. A., and Weiss, S. (1992). Generation of neurons and astrocytes from isolated cells of the adult mammalian central nervous system author (s): Brent A. Reynolds and samuel weiss. Science 255 (5052), 1707–1710. Published by : American Association for the Advancement of Science Stable URL : http://www.jstor.org/s’. doi:10.1126/science.1553558
Riegert, J., Topel, A., Schieren, J., Coryn, R., Dibenedetto, S., Braunmiller, D., et al. (2021). Guiding cell adhesion and motility by modulating cross-linking and topographic properties of microgel arrays. PLoS ONE 16 (9), 02574955–e257527. doi:10.1371/journal.pone.0257495
Riley, L., Schirmer, L., and Segura, T. (2019). Granular hydrogels: Emergent properties of jammed hydrogel microparticles and their applications in tissue repair and regeneration. Curr. Opin. Biotechnol. 60, 1–8. doi:10.1016/j.copbio.2018.11.001
Rodin, S., Domogatskaya, A., Strom, S., Hansson, E. M., Chien, K. R., Inzunza, J., et al. (2010). Long-term self-renewal of human pluripotent stem cells on human recombinant laminin-511. Nat. Biotechnol. 28 (6), 611–615. doi:10.1038/nbt.1620
Rodin, S., Kozin, S. A., Kechko, O. I., Mitkevich, V. A., and Makarov, A. A. (2020). Aberrant interactions between amyloid-beta and alpha5 laminins as possible driver of neuronal disfunction in Alzheimer’s disease. Biochimie 174, 44–48. doi:10.1016/j.biochi.2020.04.011
Rosa, A. I., Grade, S., Santos, S. D., Bernardino, L., Chen, T. C., Relvas, J., et al. (2016). Heterocellular contacts with mouse brain endothelial cells via laminin and α6β1 integrin sustain subventricular zone (SVZ) stem/progenitor cells properties. Front. Cell. Neurosci. 10 (2016), 284–314. doi:10.3389/fncel.2016.00284
Ryu, Y., Iwashita, M., Lee, W., Uchimura, K., and Kosodo, Y. (2021). A shift in tissue stiffness during hippocampal maturation correlates to the pattern of neurogenesis and composition of the extracellular matrix. Front. Aging Neurosci. 13, 709620–709710. doi:10.3389/fnagi.2021.709620
Saha, K., Keung, A. J., Irwin, E. F., Li, Y., Little, L., Schaffer, D. V., et al. (2008). Substrate modulus directs neural stem cell behavior. Biophysical J. 95 (9), 4426–4438. doi:10.1529/biophysj.108.132217
Sakiyama-Elbert, S. E. (2014). Incorporation of heparin into biomaterials. Acta Biomater. 10 (4), 1581–1587. doi:10.1016/j.actbio.2013.08.045
Sato, Y., Kiyozumi, D., Futaki, S., Nakano, I., Shimono, C., Kaneko, N., et al. (2019). Ventricular–subventricular zone fractones are speckled basement membranes that function as a neural stem cell niche. Mol. Biol. Cell. 30 (1), 56–68. doi:10.1091/mbc.E18-05-0286
Sawada, M., Matsumoto, M., and Sawamoto, K. (2014). Vascular regulation of adult neurogenesis under physiological and pathological conditions. Front. Neurosci. 8 (8 MAR), 53–57. doi:10.3389/fnins.2014.00053
Schmid, R. S., Shelton, S., Stanco, A., Yokota, Y., Kreidberg, J. A., and Anton, E. S. (2004). α3β1 integrin modulates neuronal migration and placement during early stages of cerebral cortical development. Development 131 (24), 6023–6031. doi:10.1242/dev.01532
Schmidt, S. I., Bogetofte, H., Ritter, L., Agergaard, J. B., Hammerich, D., Kabiljagic, A. A., et al. (2021). Microglia-secreted factors enhance dopaminergic differentiation of tissue- and iPSC-derived human neural stem cells. Stem Cell. Rep. 16 (2), 281–294. doi:10.1016/j.stemcr.2020.12.011
Scott, E. A., Nichols, M. D., Kuntz-Willits, R., and Elbert, D. L. (2010a). Modular scaffolds assembled around living cells using poly(ethylene glycol) microspheres with macroporation via a non-cytotoxic porogen. Acta Biomater. 6 (1), 29–38. doi:10.1016/j.actbio.2009.07.009
Scott, R. A., Elbert, D. L., and Willits, R. K. (2011). Modular poly(ethylene glycol) scaffolds provide the ability to decouple the effects of stiffness and protein concentration on PC12 cells. Acta Biomater. 7 (11), 3841–3849. doi:10.1016/j.actbio.2011.06.054
Scott, R., Marquardt, L., and Willits, R. K. (2010b). Characterization of poly(ethylene glycol) gels with added collagen for neural tissue engineering. J. Biomed. Mater. Res. - Part A 93 (3), 817–823. doi:10.1002/jbm.a.32775
Segel, M., Neumann, B., Hill, M. F. E., Weber, I. P., Viscomi, C., Zhao, C., et al. (2019). Niche stiffness underlies the ageing of central nervous system progenitor cells. Nature 573 (7772), 130–134. doi:10.1038/s41586-019-1484-9
Seidlits, S. K., Liang, J., Bierman, R. D., Sohrabi, A., Karam, J., Holley, S. M., et al. (2019). Peptide-modified, hyaluronic acid-based hydrogels as a 3D culture platform for neural stem/progenitor cell engineering. J. Biomed. Mater. Res. - Part A 107 (4), 704–718. doi:10.1002/jbm.a.36603
Seo, J-.S., Mantas, I., Svenningsson, P., and Greengard, P. (2021). Ependymal cells-CSF flow regulates stress-induced depression. Mol. Psychiatry 26 (12), 7308–7315. doi:10.1038/s41380-021-01202-1
Shen, Q., Wang, Y., Kokovay, E., Lin, G., Chuang, S. M., Goderie, S. K., et al. (2008). Adult SVZ stem cells lie in a vascular niche: A quantitative analysis of niche cell-cell interactions. Cell. stem Cell. 3 (3), 289–300. doi:10.1016/j.stem.2008.07.026
Shi, Y., Zhou, L., Tian, J., and Wang, Y. (2009). Transplantation of neural stem cells overexpressing glia-derived neurotrophic factor promotes facial nerve regeneration. Acta Oto-Laryngologica 129 (8), 906–914. doi:10.1080/00016480802468153
Silantyeva, E. A., Nasir, W., Carpenter, J., Manahan, O., Becker, M. L., and Willits, R. K. (2018). Accelerated neural differentiation of mouse embryonic stem cells on aligned GYIGSR-functionalized nanofibers. Acta Biomater. 75, 129–139. doi:10.1016/j.actbio.2018.05.052
Silantyeva, E. A., Willits, R. K., and Becker, M. L. (2019). Postfabrication tethering of molecular gradients on aligned nanofibers of functional poly(ϵ-caprolactone)s. Biomacromolecules 20 (12), 4494–4501. doi:10.1021/acs.biomac.9b01264
Silva, J., Bento, A. R., Barros, D., Laundos, T. L., Sousa, S. R., Quelhas, P., et al. (2017). Fibrin functionalization with synthetic adhesive ligands interacting with α6β1 integrin receptor enhance neurite outgrowth of embryonic stem cell-derived neural stem/progenitors. Acta Biomater. 59, 243–256. doi:10.1016/j.actbio.2017.07.013
Solano Fonseca, R., Mahesula, S., Apple, D. M., Raghunathan, R., Dugan, A., Cardona, A., et al. (2016). Neurogenic niche microglia undergo positional remodeling and progressive activation contributing to age-associated reductions in neurogenesis. Stem cells Dev. 25 (7), 542–555. doi:10.1089/scd.2015.0319
Soliman, M., Chowdhury, M. E. H., Islam, M. T., Musharavati, F., Nabil, M., Hafizh, M., et al. (2022). A review of biomaterials and associated performance metrics analysis 1198 in pre-clinical finite element model and in implementation stages for total hip implant system. Polymers 14 (20), 4308. doi:10.3390/polym14204308
Sosa, M. A. G., De Gasperi, R., Rocher, A. B., Perez, G. M., Simons, K., Cruz, D. E., et al. (2007). Interactions of primary neuroepithelial progenitor and brain endothelial cells: Distinct effect on neural progenitor maintenance and differentiation by soluble factors and direct contact. Cell. Res. 17 (7), 619–626. doi:10.1038/cr.2007.53
Stefaniak, K., and Masek, A. (2021). Green copolymers based on poly(lactic acid)—Short review. Materials 14 (18), 5254. doi:10.3390/ma14185254
Stukel, J. M., and Willits, R. K. (2018). The interplay of peptide affinity and scaffold stiffness on neuronal differentiation of neural stem cells. Biomed. Mater. (Bristol) 13 (2), 024102. doi:10.1088/1748-605X/aa9a4b
Sun, J., Zhou, W., Ma, D., and Yang, Y. (2010). Endothelial cells promote neural stem cell proliferation and differentiation associated with VEGF activated Notch and Pten signaling. Dev. Dyn. 239 (9), 2345–2353. doi:10.1002/dvdy.22377
Sun, Z., Guo, S. S., and Fässler, R. (2016). Integrin-mediated mechanotransduction. J. Cell. Biol. 215 (4), 445–456.doi:10.1083/jcb.201609037
Takamura, T., Motosugi, U., Sasaki, Y., Kakegawa, T., Sato, K., Glaser, K. J., et al. (2020). Influence of age on global and regional brain stiffness in young and middle-aged adults. J. Magnetic Reson. Imaging 51 (3), 727–733. doi:10.1002/jmri.26881
Tate, C. C., Shear, D. A., Tate, M. C., Archer, D. R., Stein, D. G., and LaPlaca, M. C. (2009). Laminin and fibronectin scaffolds enhance neural stem cell transplantation into the injured brain. J. tissue Eng. Regen. Med. 3 (3), 208–217. doi:10.1002/term.154
Urbán, N., Blomfield, I. M., and Guillemot, F. (2019). Quiescence of adult mammalian neural stem cells: A highly regulated rest. Neuron 104 (5), 834–848. doi:10.1016/j.neuron.2019.09.026
Urbán, N., and Guillemot, F. (2014). Neurogenesis in the embryonic and adult brain: Same regulators, different roles. Front. Cell. Neurosci. 8 (NOV), 396–419. doi:10.3389/fncel.2014.00396
Uyar Tamer, K. E. (2017). “13Electrospun scaffolds for neural tissue engineering,” in Electrospun materials for tissue engineering and biomedical applications - research, design and commercialization. (Elsevier). Available at: https://app.knovel.com/hotlink/khtml/id:kt011FUM31/electrospun-materials/electrospu-electrospun-7.
Vagaska, B., Gillham, O., and Ferretti, P. (2020). Modelling human CNS injury with human neural stem cells in 2- and 3-Dimensional cultures. Sci. Rep. 10 (1), 6785–6814. doi:10.1038/s41598-020-62906-y
Vay, S. U., Flitsch, L. J., Rabenstein, M., Rogall, R., Blaschke, S., Kleinhaus, J., et al. (2018). The plasticity of primary microglia and their multifaceted effects on endogenous neural stem cells in vitro and in vivo. J. Neuroinflammation 15 (1), 226–321. doi:10.1186/s12974-018-1261-y
Wang, J., Chu, R., Ni, N., and Nan, G. (2020). The effect of Matrigel as scaffold material for neural stem cell transplantation for treating spinal cord injury. Sci. Rep. 10 (1), 2576–2611. doi:10.1038/s41598-020-59148-3
Wang, T.-W., Zhang, H., and Parent, J. M. (2005). Retinoic acid regulates postnatal neurogenesis in the murine subventricular zone-olfactory bulb pathway. Development 132 (12), 2721–2732. doi:10.1242/dev.01867
Wang, Y. A. Z., Plane, J. M., Jiang, P., Zhou, C. J., and Deng, W. (2011). Concise review: Quiescent and active states of endogenous adult neural stem cells: Identification and characterization. Stem Cells 29 (6), 907–912. doi:10.1002/stem.644
Willerth, S. M., and Sakiyama-Elbert, S. E. (2019). Combining stem cells and biomaterial scaffolds for constructing tissues and cell delivery. StemJournal 1 (1), 1–25. doi:10.3233/stj-180001
Wilson, K. L., Perez, S. C. L., Naffaa, M. M., Kelly, S. H., and Segura, T. (2022). Stoichiometric post modification of hydrogel microparticles dictates neural stem cell fate in microporous annealed particle scaffolds. Adv. Mater. 2201921, 2201921. doi:10.1002/adma.202201921
Winkelman, M. A., Koppes, A. N., Koppes, R. A., and Dai, G. (2021). Bioengineering the neurovascular niche to study the interaction of neural stem cells and endothelial cells. Apl. Bioeng. 5 (1), 011507. doi:10.1063/5.0027211
Wood, M. D., Hunter, D., Mackinnon, S. E., and Sakiyama-Elbert, S. E. (2010). Heparin-binding-affinity-based delivery systems releasing nerve growth factor enhance sciatic nerve regeneration. J. Biomaterials Sci. Polym. Ed. 21 (6–7), 771–787. doi:10.1163/156856209X445285
Xin, S., Wyman, O. M., and Alge, D. L. (2018). Assembly of PEG microgels into porous cell-instructive 3D scaffolds via thiol-ene click chemistry. Adv. Healthc. Mater. 7 (11), 1800160–1800216. doi:10.1002/adhm.201800160
Yamada, M., and Sekiguchi, K. (2015). “Chapter six - molecular basis of laminin–integrin interactions,” in Basement membranes. Editor J. H. Miner (Academic Press Current Topics in Membranes), 197–229. doi:10.1016/bs.ctm.2015.07.002
Yang, H., Zhu, Q., Cheng, J., Wu, Y., Fan, M., Zhang, J., et al. (2019). Opposite regulation of Wnt/β-catenin and Shh signaling pathways by Rack1 controls mammalian cerebellar development. Proc. Natl. Acad. Sci. U. S. A. 116 (10), 4661–4670. doi:10.1073/pnas.1813244116
Yap, L., Tay, H. G., Nguyen, M. T., Tjin, M. S., and Tryggvason, K. (2019). Laminins in cellular differentiation. Trends Cell. Biol. 29 (12), 987–1000. doi:10.1016/j.tcb.2019.10.001
Yin, Y., Wang, W., Shao, Q., Li, B., Yu, D., Zhou, X., et al. (2021). Pentapeptide IKVAV-engineered hydrogels for neural stem cell attachment. Biomaterials Sci. 9 (8), 2887–2892. doi:10.1039/d0bm01454k
Yousef, H., Morgenthaler, A., Schlesinger, C., Bugaj, L., Conboy, I. M., and Schaffer, D. V. (2015). Age-associated increase in BMP signaling inhibits hippocampal neurogenesis. Stem Cells 33 (5), 1577–1588. doi:10.1002/stem.1943
Yousif, L. F., Di Russo, J., and Sorokin, L. (2013). Laminin isoforms in endothelial and perivascular basement membranes. Cell. Adhesion Migr. 7 (1), 101–110. doi:10.4161/cam.22680
Yu, C., Griffiths, L. R., and Haupt, L. M. (2017). Exploiting heparan sulfate proteoglycans in human neurogenesis—Controlling lineage specification and fate. Front. Integr. Neurosci. 11, 28–15. doi:10.3389/fnint.2017.00028
Zappaterra, M. W., and Lehtinen, M. K. (2012). The cerebrospinal fluid: Regulator of neurogenesis, behavior, and beyond. Cell. Mol. Life Sci. 69 (17), 2863–2878. doi:10.1007/s00018-012-0957-x
Zekonyte, J., Sakai, K., Nicoll, J. A., Weller, R. O., and Carare, R. O. (2016). Quantification of molecular interactions between ApoE, amyloid-beta (Aβ) and laminin: Relevance to accumulation of Aβ in Alzheimer’s disease. Biochimica Biophysica Acta - Mol. Basis Dis. 1862 (5), 1047–1053. doi:10.1016/j.bbadis.2015.08.025
Zhang, D., Yang, S., Toledo, E. M., Gyllborg, D., Salto, C., Carlos Villaescusa, J., et al. (2017). Niche-derived laminin-511 promotes midbrain dopaminergic neuron survival and differentiation through YAP. Sci. Signal. 10 (493), eaal4165. doi:10.1126/scisignal.aal4165
Zhang, J., Liu, X., Ma, K., Chen, M., Xu, H., Niu, X., et al. (2021). Collagen/heparin scaffold combined with vascular endothelial growth factor promotes the repair of neurological function in rats with traumatic brain injury. Biomaterials Sci. 9 (3), 745–764. doi:10.1039/c9bm01446b
Zhang, J., Wang, R. J., Wang, R. J., Chen, M., Liu, X. Y., et al. (2021). Collagen/heparan sulfate porous scaffolds loaded with neural stem cells improve neurological function in a rat model of traumatic brain injury. Neural Regen. Res. 16 (6), 1068–1077. doi:10.4103/1673-5374.300458
Zhao, X., and Moore, D. L. (2018). Neural stem cells: Developmental mechanisms and disease modeling. Cell. tissue Res., 1–6. doi:10.1007/s00441-017-2738-1
Zhao, X., Wang, Y., Wait, E., Mankowski, W., Bjornsson, C. S., Cohen, A. R., et al. (2021). 3D image analysis of the complete ventricular-subventricular zone stem cell niche reveals significant vasculature changes and progenitor deficits in males versus females with aging. Stem Cell. Rep. 16 (4), 836–850. doi:10.1016/j.stemcr.2021.03.012
Zhou, W., Stukel, J. M., Cebull, H. L., and Willits, R. K. (2016). Tuning the mechanical properties of poly(ethylene glycol) microgel-based scaffolds to increase 3D schwann cell proliferation. Macromol. Biosci. 16 (4), 535–544. doi:10.1002/mabi.201500336
Zhu, J. (2010). Bioactive modification of poly(ethylene glycol) hydrogels for tissue engineering. Biomaterials 31 (17), 4639–4656. doi:10.1016/j.biomaterials.2010.02.044
Zhu, M, M., Wang, Y., Ferracci, G., Zheng, J., Cho, N. J., and Lee, B. H. (2019). Gelatin methacryloyl and its hydrogels with an exceptional degree of controllability and batch-to-batch consistency. Sci. Rep. 9 (1), 6863–6913. doi:10.1038/s41598-019-42186-x
Zhu, Y, Y., Li, X., Janairo, R. R. R., Kwong, G., Tsou, A. D., Chu, J. S., et al. (2019). Matrix stiffness modulates the differentiation of neural crest stem cells in vivo. J. Cell. Physiology 234 (5), 7569–7578. doi:10.1002/jcp.27518
Keywords: subventricular zone, neural stem cells, stem cell niche, extracellular matrix, fractone, neural tissue repair, biomaterials
Citation: Yazdani N and Willits RK (2023) Mimicking the neural stem cell niche: An engineer’s view of cell: material interactions. Front. Chem. Eng. 4:1086099. doi: 10.3389/fceng.2022.1086099
Received: 01 November 2022; Accepted: 28 December 2022;
Published: 16 January 2023.
Edited by:
Siti Shawalliah Idris, Universiti Teknologi MARA, MalaysiaReviewed by:
Yoke Chin Chai, Interuniversity Microelectronics Centre (IMEC), BelgiumNoor Fitrah Abu Bakar, Universiti Teknologi MARA, Malaysia
Copyright © 2023 Yazdani and Willits. This is an open-access article distributed under the terms of the Creative Commons Attribution License (CC BY). The use, distribution or reproduction in other forums is permitted, provided the original author(s) and the copyright owner(s) are credited and that the original publication in this journal is cited, in accordance with accepted academic practice. No use, distribution or reproduction is permitted which does not comply with these terms.
*Correspondence: Rebecca Kuntz Willits, r.willits@northeastern.edu