- 1Tecnologico de Monterrey, School of Engineering and Sciences, Monterrey, Mexico
- 2School of Chemistry, University of St Andrews, St Andrews, United Kingdom
- 3Tecnologico de Monterrey, Institute of Advanced Materials for Sustainable Manufacturing, Monterrey, Mexico
The annual global fish production reached a record 178 million tonnes in 2020, which continues to increase. Today, 49% of the total fish is harvested from aquaculture, which is forecasted to reach 60% of the total fish produced by 2030. Considering that the wastes of fishing industries represent up to 75% of the whole organisms, the fish industry is generating a large amount of waste which is being neglected in most parts of the world. This negligence can be traced to the ridicule of the value of this resource as well as the many difficulties related to its valorisation. In addition, the massive expansion of the aquaculture industry is generating significant environmental consequences, including chemical and biological pollution, disease outbreaks that increase the fish mortality rate, unsustainable feeds, competition for coastal space, and an increase in the macroalgal blooms due to anthropogenic stressors, leading to a negative socio-economic and environmental impact. The establishment of integrated multi-trophic aquaculture (IMTA) has received increasing attention due to the environmental benefits of using waste products and transforming them into valuable products. There is a need to integrate and implement new technologies able to valorise the waste generated from the fish and aquaculture industry making the aquaculture sector and the fish industry more sustainable through the development of a circular economy scheme. This review wants to provide an overview of several approaches to valorise marine waste (e.g., dead fish, algae waste from marine and aquaculture, fish waste), by their transformation into biofuels (biomethane, biohydrogen, biodiesel, green diesel, bioethanol, or biomethanol) and recovering biomolecules such as proteins (collagen, fish hydrolysate protein), polysaccharides (chitosan, chitin, carrageenan, ulvan, alginate, fucoidan, and laminarin) and biosurfactants.
1 Introduction
The demand for fish meat is increasing, however, capture fisheries production remains static or is diminishing, depending on the species in question, during the last decades. The aquaculture industry has been recognised to have a critical role in food production, so it is growing at the fastest rate in the world. Up today, the aquaculture industry produces 48% of the total fish produced and it is estimated that by 2030 the demand for aquaculture will reach 57% of the total global production (OECD-FAO, 2021). This industry involves cultures of fish or aquatic organisms, either in freshwater or marine culture. It is commonly associated with fish farming since it is the most relevant one for human consumption, but it also covers other forms of aquatic animal and plant production, such as crustaceans, molluscs, algae and seaweed, and others (Ahmad et al., 2021). Nevertheless, the fast growth of the aquaculture industry and its intensive activities are creating a negative impact on the environment caused of the excessive release of nutrients into the sea, causing eutrophication of coastal areas and other aquatic systems (Sarà et al., 2018). The excessive formation of algae decreases the oxygen level in the water and increases the level of toxins in the water due to their degradation affecting the mortality rate of the wild population (Van Osch et al., 2017; Mangano et al., 2019). Oliviera et al. (2021) reported that more than 10 million fish die annually around the world for environmental factors which directly affect the increase of infectious agents and parasites. The high mortality generates a massive amount of low-value waste that is difficult to recycle due to restricted legislation.
The significant environmental issues caused by intensive aquaculture increased the interest in alternative sustainable practices, such as integrated multi-trophic aquaculture (IMTA) (Alexander et al., 2016; Sarà et al., 2018). Integrated multi-trophic aquaculture aims to incorporate the production of aquaculture species of different trophic levels under a circular economy approach, minimising energy losses, environmental deterioration and valorising the waste products (Buck et al., 2018). This novel system uses algae to capture nutrient and inorganic solid waste and convert them into feed, fertiliser and possible substrate to produce biofuel (Correia et al., 2020). The use of this novel aquaculture concept could reduce the fish mortality both for the aquaculture and for the wild fish up to 5.5% which is closer to mortality rates on egg-laying hen farms. It has been calculated for Norwegian farmers that reach the level of mortality mentioned above, it could generate an annual saving of over $892 million USD (Just Economy, 2021).
Despite the environmental benefits of integrated multi-trophic aquaculture (IMTA), aquaculture and the fisheries industry produce a large amount of waste which is not included in the circular economy of the process and takes into account dead fish, algae waste, and all the waste generated by fish manufactured (e.g., viscera, skin, bonds, and heads). The transformation of waste products into biofuel and bioderivatives will help to further reduce the carbon footprint of the aquaculture industry (4-75KgCO2/Kg protein), replacing the utilization of fossil fuel resources. In 2018, world fish production reached about 179 million tonnes with a total first sale value estimated at USD 401 billion, 82.1 million tonnes of that fish production, which is the largest in aquaculture industry, of which 82 million tonnes, valued at USD 250 billion, came from aquaculture production, followed by aquatic algae production, dominated by seaweed, with 32.4 million tonnes produced (Figure 1).
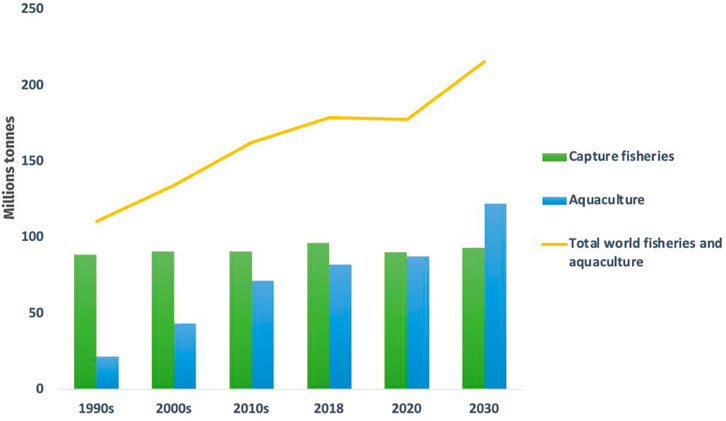
FIGURE 1. Global capture fisheries and aquaculture production, 1990–2030 Adapted from FAO, 2022b.
These two products had a total farmgate value of USD 263.6 billion. Of the total global fish production, about 88% was used for human consumption, equivalent to an estimated annual supply of 20.5 kg per capita (FAO, 2020). Considering that 50%–60% of the whole organism is wasted annually, we generate a waste stream of 134 million tons/yr. While the waste generates from fish mortality is difficult to quantify due to the main factors that affect the value. Oliviera et al. (2021) reported that in Norway, the salmon industry generated 50 million tonnes per year of dead fish in 2018, while in Scotland 8.3 million tonnes per year. A recent report published by Just Economy (2021) focuses on the main aquaculture industry in Norway, Scotland, Chile and Canada reported that the fish mortality due to different causes is the main cost for the salmon aquaculture industry (15,539 MUSD). The mortality also generate of Environmental and social costs which account for 19,195 MUSD.
With respect to the waste represented by the fisheries and aquaculture, it is estimated that 35% of the global harvest is either lost or wasted every year, meaning that in the year of 2018 about 28.7 million tonnes of the aquaculture production were lost, which translates to the loss of USD 87.5 billion. In most regions of the world, total fish loss and waste lies between 30%–35%. With the rapid growth of this industry and the expansion of fish processing, the number of by-products obtained from aquaculture has also increased (Khawli et al., 2019), these may represent up to 70% of processed fish (FAO, 2020). In the past, these by-products were treated as marine waste, used directly as feed for aquaculture, livestock, or employed to produce fish oil, fertilizer, pet food and fish silage, most of these recycled products possess low economic value allowing only a minimal amount of the capital invested in the aquaculture production to be recovered. However, this waste can now be used more efficiently as a source of marine biomass with a great diversity of biotechnological applications. Recent studies have identified a number of bioactive compounds from remaining fish muscle proteins, collagen and gelatin, fish oil, fish bone, internal organs and shellfish and crustacean shells. These bioactive compounds can be extracted, purified, transformed, and exploited as a consequence of the improved processing technologies varying from simple to complex techniques. Such compounds may include the preparation and isolation of bioactive peptides, polyphenols, polysaccharides, oligosaccharides, fatty acids, enzymes and biopolymers for biotechnological applications (Kim and Mendis, 2006; Khawli et al., 2019; Rudovica et al., 2021) such as the synthesis of biofuels, biosurfactants, biochar and other bioproducts. Global food loss and waste is one of the main concerns regarding sustainable development, for this reason, it is the focus of Sustainable Development Goal (SDG) Target 12.3, which aims at halving wastage by 2030 (FAO, 2020). The most extensively used and promising alternative to achieve this SDG goal is the use the exploitation of marine biomass and valorisation of seafood by-products either directly or by the extraction of bioproducts, leading to more environmentally sustainable uses of marine resources and higher economic benefits, in line with the circular economy concepts (Rudovica et al., 2021) that can provide a continued supply of aquatic products beneficial for human consumption without harming existing ecosystems or exceeding the ability of the planet to renew the natural resources required for aquaculture production.
Regarding the aquatic algae production, algae play a very important role in many ecosystems, providing food and shelter for many different species of aquatic animals. However, when uncontrolled growth occurs, their impact on the ecosystem can be harmful. The excessive growth of marine algae is called “algal blooms” there are several factors that contribute to algal blooms, including limiting nutrients, climate change, and pollution. Although some blooms occur naturally, others are caused by human intervention. Algae bloom affects not only ecological balances but also fundamental economic activities in the territory, such as fishing and tourism, potentially even affecting public health (Jena and Hoekman, 2017). Beach wrack is another concerning algae bloom, it consists of organic material like seagrass or seaweed biomass which accumulates on beaches due to the action of waves, tides, and non-periodical water level fluctuations. Despite the natural origin of most of this material and its significant ecological role, beach wrack often becomes an environmental issue if accumulated in excessive amounts (Rudovica et al., 2021). Although the negative impact that algal blooms can have on an ecosystem and the environment due to their overproduction and degradation, they could represent a solution and resource if they are collected and reused for the production of biofuel (The Climate Institute, 2017).
Indeed, algae waste from marine and aquaculture is a sustainable and optimal solution for producing third-generation fuel. Seaweeds (U. lactuca) collected from the beach could become profitable as it gathers in blooms that need to be removed from beaches. Thus, harvest cost is cheap, and it may even attract a gate fee. Allen et al. (2015) reported that the potential Gross energy yields can be on the order of 186 GJ ha−1 yr−1, which is higher than the first-generation liquid biofuel like ethanol (135 GJ ha−1 yr−1) produced from sugarcane or biodiesel (120 GJ ha−1 yr−1) from palm oil.
Algae biomass has enormous potential to also high-value products like nutraceuticals, proteins and other functional ingredients (Jena and Hoekman, 2017). This review aims to highlight the importance of using marine and aquaculture waste and assessing current technologies to convert this waste into high-value products in line with new sustainability trends in industries such as the circular economy by using what was once waste and converting it into new valuable products for human use.
2 Bio-based circularity economy: Exploring the impact fish-aquaculture-marine waste conversion into products
The circular bio-based economy target to reuse all types of sustainable sourced biomass and degradable waste from many sectors and transforming it into a wide-spectrum of high value products including biofuels, biomaterials, bioactive compounds, among others (Vishwakarma et al., 2022).
The circular economy has gain momentum by returning materials and waste into sources towards making a sustainable and zero-waste environment. The global energy demand is estimated to increase approximately 28% by the year 2040 and there is much interest in biofuels production under sustainable circular bioeconomy because they are eco-friendly and are preferred for their carbon neutral character, production flexibility from several and versatile sources and renewability (Ranjbari et al., 2022).
The use of the biofuels as an alternative to fossil fuel and technologies for its production is improving day by day. The main source of biofuels is the organic matter called biomass, it could be plants, waste, farming residue, waste from sewage, manure, etc. Recently, the biofuels from crops and plants have been taken relevance. Additional to plants, algae, microalgae seaweeds and seagrass founded to be potential reserves and solution in the energetic transition. The suitable biofuels production from third and fourth generation is not competing with land and food leading to lot benefits in transport sector (Priya et al., 2021).
The rapid growth of aquaculture sector has resulted in massive waste, residuals products and environmental concerns has been conflicted also with the amount of water utilised (Azwar et al., 2022). Considering that 35% of the global harvest in fisheries and aquaculture is lost as waste every year, there is a gap of opportunities to valorise and minimize the total discarded. The sociological and environmental impact complain to construct regulatory frameworks, services and infrastructure to reduce fish lost and waste (Rudovica et al., 2021).
For example, alternative valorisation routes for scale, skin, bones, tails, and fins are needed. The conversion of these residuals into valuable materials has numerous advantages. Fish has high oil content which one has been shown to be suitable source for biofuel production, also can be converted into biodiesel via chemical catalysis and enzymatic approaches. Additionally, this kind of biowaste permits the methane production derived from its composition (Lee et al., 2022).
Algae circular economy has gained interest in the last years in the bioplastic production. In case of microalgae, is a renewable resource with high potential for bioplastic production, several strains have been evaluated on their functionality for production of polyhydroxyalkanoates (PHA′s). Macroalgae and seaweed are consider a low-cost source of bioderivatives such as alginate, carrageenan, fucoidan and ulvan which ones after their extraction and purification could be competitive and commercially expensive products (Dang et al., 2022).
Food loses and waste not only means a reductions on the quantity of the marine products from catch and harvest, it means also the decrease of its quality and nutritional values, additional on the impact on the economic sector. To avoid and prevent issues related to food and safety and fish loss, is priority to recognize and validate how to implement all stages of the fisheries and aquaculture value chains. Training on food safety and requirements should be provided to all the actors in the value chain of fishering and aquaculture and ensure the safe aquatic products on the market and fish loss reduction globally (FAO, 2022a).
Contributing to the significant progress made in the reuse of marine biomass, research, and innovation on technology for scale-up must be establish a nexus and collaboration between industry and government to promote sustainability and a circular economy in order to enhance the aquaculture productivity and efficiency on supply chains.
3 Advanced production of biofuels from fish-aquaculture waste, and algae bloom
Nowadays, 80% of the energy supply is produced by fossil fuels (Renewables, 2021). Energy demands are increasing worldwide due to industrialisation, population growth and modernisation, leading to the over-exploitation of limited available natural fossil fuel reserves (Kumar et al., 2020a). The high utilization of fossil fuels represents a massive issue due to gas emissions, which are the main cause of global warming. To mitigate this issue is fundamental to replace fossil fuels with third-generation biofuels, which use feedstocks that do not compete with food, reduce pressure on the land due to the low amount available, and need to be abundant to satisfy the current oil demand (Jamil et al., 2018; Mansir et al., 2018, Singh et al., 2019). To achieve this, it is necessary to use waste sources. Despite agricultural, industrial, and household, organic waste has been used for a long time as feedstocks to generate power and small quantities of synthetic oils that are not enough to satisfy the global demand (Skaggs et al., 2018). Today, there is a large number of unexplored waste sources generated from aquaculture, marine and fish industry waste but also use of biomass growth generated by photosynthesis, like algae, that could help to match the energy demand and the use of these additional wastes (Tsukahara et al., 2001; Kumar et al., 2020b). Using waste biomass represents a sustainable strategy because it reduces the utilization of food crops, optimises waste management, and reduces the gas emissions generated by waste disposal in landfills and the combustion of fossil fuels. Hence, waste biorefineries are attracting significant interest worldwide because required energy needs are met, and a solution to the waste management problem is found in the circular economy context (Tuck et al., 2012; Ahrens et al., 2017; Yuvaraj et al., 2019). The industrial processes focused on valorising terrestrial biomass are well established, but marine sources still represent an untapped resource.
The fish industry is one of the world’s largest industries, where tons of fish are used daily. Every year a billion tons of fish are utilised for edible purposes, but it also generates a large amount of waste fish derived from a high mortality rate in the marine environment of the aquaculture industry and non-edible parts (e.g., head, viscera, dorsal fins, tail, skin, and liver) derived from the fish processing. This non-edible waste is considered worthless garbage and discarded without recovering valuable products by dumping on land or hauling it into the ocean (Milano et al., 2016).
The marine and aquaculture industry also produces a large amount of algae waste. Lipid-rich sources offer an attractive choice for a biofuel feedstock due to its high CO2-fixing capabilities (Milano et al., 2016). Algae absorb about 183 gigatons of CO2 while growing about 100 gigatons of algal-cell biomass (Dumay et al., 2004; Schenk et al., 2008).
Generally, 70% of the Earth’s surface is occupied by oceans and seas that are intensively used by the fishery and aquaculture industries. The amount of waste generated is massive, mainly composed of seaweed, dead fish and fish waste derived from the manufacturing industry. This waste is rich in compounds that can be converted into biofuel using biological and chemical processes. Different conversion technologies are used to produce biofuels, such as biochemical–anaerobic digestion (biogas) and fermentation (bioethanol) and chemical conversion–extraction and transesterification (biodiesel) (Figure 2).
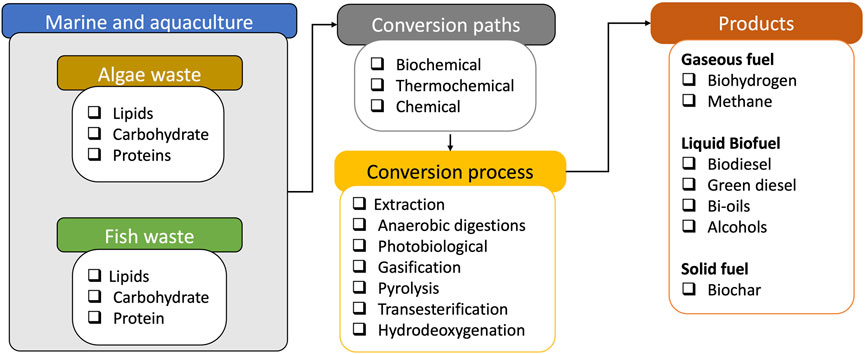
FIGURE 2. Schematic description of marine waste and fish waste products conversion path and products.
In this section will evaluate the advantages and disadvantages of the technologies to convert fish and algae waste into gaseous fuel (biomethane and bio-hydrogen) used to decarbonise the domestic and industrial sector and liquid (biodiesel, green diesel) used to decarbonise the heavy transportation sector.
3.1 Biogas and biomethane
Anaerobic digestion is a well-established technology that converts organic waste into clean bioenergy. Biogas and digested substrate (digestate) are the products of anaerobic digestion. The biogas usually contains 55%–65% CH4, 35%–45% CO2, 0%–3% N2, 0%–1% H2, and 0%–1% H2S (Milono et al., 1981).
The anaerobic digestion of organic waste is a complex process composed of a series of bio-metabolism steps, which include hydrolysis, acidogenesis, acetogenesis, and methanogenesis, respectively (Figure 3).
The mechanisms reported above are the main ones responsible for the kinetic of reactions, and they are highly dependent on environmental and/or ambient conditions such as temperature, pH, C/N ratio, C/P ratio, particle size, inhibitors, and type of substrate (Mata-Alvarez et al., 2000). The first step of the process is hydrolysis, where enzymes decompose the complex polymeric structures of cellulose, starch and proteins into monomers or oligomers such as glucose, fatty acids, and amino acids. This process is quite fast, but it can be limited by the presence of lignin-rich substrates. Ariunbaatar et al. (2014) report that this step process can be accelerated by introducing specific enzymes.
The second step (acidogenesis) transforms the products of the hydrolytic process into volatile fatty acids (VFAs), alcohols and ketones. This process is fast (30 min, and this causes an accumulation of VFA accumulation in the digester, resulting in digester toxicity if not properly controlled by operational conditions, substrate composition, and microbial population in the anaerobic digestion system (Lukitawesa-Patinvoh et al., 2020). The pH is the operating parameter that affects most the VFA formation, and it was reported that the optimal pH range is 5.5–6.5 (Mao et al., 2015).
The third step (acetogenesis) transforms most products of acidogenesis and some of the long-chain fatty acids from the hydrolysis stage into acetate, CO2, and H2 into (CH3COO-), hydrogen (H2), and carbon dioxide (CO2) and the kinetic of reaction is reported to be in a range of 1.5–4 days (Ramos-Suárez et al., 2015). This step is sensible to the presence of H2 and O2, and the operating pH has to be in a range between 6–6.2 (Geraldi, 2003; Burton et al., 2014; Stronach et al., 2012).
The fourth step (methanogenesis) plays an essential role in generating methane gas by methanogens. There are two primary mechanisms for methane generation, including acetoclastic (CH3 COOH→CH4+CO2) and hydrogenotrophic methanogenesis (CO2+4H2→CH4+2H2O). Typically, methanogens are extremely sensitive to pH conditions, the presence of oxygen, and other factors such as free ammonia (FAN), H2S, and Volatile Fatty Acids (VFAs) (Geraldi, 2003; Mao et al., 2015; Ramos-Suárez et al., 2015; Van et al., 2020).
Most of the literature on anaerobic digestion uses municipal waste and agricultural waste as substrates. However,Xu et al. (2018) reported that the digestion of food waste has more advantages, such as mitigation of climate change, economic benefits and diversion opportunities. For example, Bartocci et al. (2020) reported that by replacing 9,900 tonnes of corn silage with 6,600 tonnes of food waste, it is possible to reduce CO2 emission by up to 42% of the electricity produced from the biogas plant could be achieved. The benefit of the anaerobic digestion of food waste against agricultural waste creates a strong interest in using fish and algae waste as substrates has been growing since 2018 due to the incredible potential of these sources for the production of biogas through anaerobic digestion.
Fish waste is a complex substrate because the composition of solid and liquid fish processing waste depends on the composition of the fish species used, which in turn depends on the sex, feeding habits, season and health of fish.
These wastes contain high levels of protein (up to 60%), fat (up to 20%) and minerals (calcium and hydroxyapatite from bones and scales), and the high content of fat seems to favour the yielding of the biomethane. An optimised model for anaerobic digestion reported that the production of biomethane can vary from .2 to .9 CH4 m3/kg VS. added by using different forms of fish waste (Kaspars et al., 2018) (Table 1)
Despite the methane production shown in Table 1, using fish waste as substrate can cause operational problems. Indeed, fish waste releases high levels of ammonia when digested, which inhibits the digestion of substrates (Achinas et al., 2017). High ammonia concentrations can result in the accumulation of VFAs (acetic acid as the main type in the batch tests). To mitigate the negative effect of ammonia formation co-digestion process is a possible technological solution. One of the best ways to co-digest fish waste is with agricultural waste or algae, as reported in Table 2. Nazurally (2018) reported that a general accumulation of VFAs was observed for co-digestion of algae and fish, and this phenomenon was due to the high content of fatty acid. The co-digestion is not the only way to control free ammonia formation and the accumulation of VFAs, but they can also be controlled by the reactor, type and organic loading rate and pH (Shi et al., 2017).
Table 2 reports that the digestion of pure algae produces much less methane when macroalgae are processed alone. The co-digestion of algae and fish waste seems an optimal solution for an environmentally and economically sustainable process. The use of pure macroalgae waste in the bioreactor is not economically sustainable due to several technical issues, such as the seasonal growth associated with different types of macroalgae, the variability of the feedstock and operational costs (Ward et al., 2014; Milledge et al., 2019). In addition, the use of marine waste macroalgae produce several problems, such as difficulty in processing material such as polyphenols, cellulosic fibres and lignin-type components. This results in the reduced biodegradability of the biomass by bacterial processes and thus a limiting digestibility and gas production (Briand and Morand, 1997). Microalgae waste could be more valuable if microalgae species were grown as part of a wastewater treatment process, but this review will not discuss it.
3.2 Biohydrogen
Biohydrogen generated from a renewable and sustainable source is a clean energy carrier since the combustion process leads to water formation, which creates an attractive energy source compared to other renewable sources. Nowadays, 96% of hydrogen (grey) is produced through carbon-intensive processes, where steam reforming of natural gas accounts for 48% of total production capacity, while petroleum fractionation and coal gasification make up 30% and 18% of production capacity, respectively (Franchi et al., 2020). The remaining 4% of hydrogen is considered green because it is produced by renewable sources and water electrolysis. Considering the actual percentage of green hydrogen, the decarbonisation of hydrogen production (grey hydrogen) is a potential paradigm that can be only solved by implementing carbon capture and storage (CCS) integration (blue hydrogen) or considering the use of clean energy sources (green hydrogen). Using clean energy sources is expected to reduce annual carbon dioxide emissions by nearly 440 million tonnes in 2050 (Nicita et al., 2020).
The conversion of waste biomass represents an inexpensive alternative for bio-hydrogen generation because the technologies used do not require high energy consumption compared to water electrolysis. The electricity cost accounts for 80% of the total cost of hydrogen production by electrolysis (Kapdan and Kargi, 2006).
The methods for biohydrogen production can essentially be categorised into two main classes: The thermochemical conversion (pyrolysis and gasification) route relies on high-temperature operations to degrade biomass wastes to produce biohydrogen, where the types and conditions of feedstocks used can heavily influence the outcome of the products.
The biochemical conversion (dark fermentation and photobiological) fermentation route emphasises more on the physical conditions of the medium (living organisms) and the types of catalysts used. In both processes, the hydrogen must be separated by several technologies, such as absorption, adsorption, membrane separation, and cryogenics separation, irrespective of upstream processing routes (Ren and Toniolo, 2018; Jiménez-Llanos et al., 2020).
Studies on several types of biomass waste reported that hydrogen production via the thermochemical pathway seems techno-economic more viable than the biochemical pathways. The thermochemical pathway is proven at a commercial scale, the cost of the catalysts is lower, and the technical challenges are limited compared to the biochemical pathway (e.g., biomass pre-treatment, bioreactor design and restriction to hydrogen), and they are able to achieve overall efficiency in a range between 50%–70% depending on the operating conditions (Dascomb et al., 2013; Kannah et al., 2021).
The gasification process is the most efficient method of hydrogen production from waste products due to the high biomass conversion in the gas phase. Pyrolysis is an ideal method of hydrogen production only at high operating temperatures (over 600°C) and or at low temperatures if the pyrolysis plant is coupled with a steam reforming system able to hydrocrack the bio-oil produced. The thermochemical pathways required biomass waste with a moisture content not higher than 20%. The biomass with moisture content higher than 20%, such as fish or algae, must be dried. The procedure requires an additional power source or an optimised heat exchange system to reduce the moisture contained in the waste before being fed into the system. Thus, using waste with higher moisture will reduce the efficiency of the process and increase the production of hydrogen costs. On the other side, fish waste and algae are inexpensive biomass waste that produces a third-generation of biofuel at low cost. Recent studies on fish waste and algae are easy to process and obtain bio-oil or syngas as precursors in synthetic fuel production. Rowland et al. (2009) successfully demonstrated that fish waste with high moisture content could easily gasify fish waste with dried pellet wood to control the moisture level upstream of the gasifier reactor. The study also demonstrates that adding fish to the pellet wood reduces the High Heating Value (HHV) of the syngas produced, which is still high enough to make this process feasible in rural areas or for local use. Reza et al. (2022) successfully demonstrated that fish waste could easy be gasified into syngas at high temperatures without reducing the moisture content of the biomass. They reported the high syngas production and, thus, hydrogen was achieved operating at a temperature over 600°C. The high temperature favoured the cracking reaction of bio-oils contained in the fish and produced during the pyrolysis process, consequently increasing the fraction of gas produced up to 43% at 600°C. The percentage can be increased by increasing the temperature and ramp rate moving from conventional pyrolysis to a fast pyrolysis process. However, they also reported that using fish waste as feedstock produces a high amount of ash that must be removed during operations, which can affect the operating costs and, consequentially, the biofuel costs. Cao et al. (2020) reported that steam gasification is one of the most efficient processes to produce syngas and hydrogen using biomass with higher moisture content. The process is suitable for large-scale industrial production with a high gasification rate and low ash production. The utilization of a catalyst in this process reduces the operating temperature and favours the gasification process. The introduction of steam increases hydrogen production. However, the process has challenges that must be faced up, such as decreasing the tar contents, optimising the composition of the catalyst to minimise the deactivation, and reducing the energy and material costs. Duman et al. (2014) reported that the feasibility of steam gasification was affirmed by using micro and macroalgae as feedstock. The studies conducted showed that the hydrogen production capacity of macroalgae was much higher than that of microalgae. The maximum hydrogen production depends on the inorganic content of raw materials in macroalgae (18%), which favours gasification and could reach 1,036 ml/g of hydrogen production against 413 ml/g from microalgae.
The studies on biomass gasification reported successful studies on fish and algae waste. The studies report that gasification is a promising technology for hydrogen production and operating parameters such as steam/biomass, pressure temperature and water contents) that drastically affects hydrogen production, as reported in different studies (Han et al., 2013; Iovane et al., 2013; Zhang et al., 2015a).
Thermochemical processes of biomass are a mature and efficient process for converting both dry and wet biomass, but this requires high temperatures and biomass pre-treatment to feed wet biomass. Hence, the scientific community is focusing on developing a biochemical process that has the advantages of using low operating temperatures and microbial to convert wet biomass such as algae and fish waste into biohydrogen. They are still under investigation and have been proven mainly at the lab scale.
Nowadays, thermochemical conversion is the only one mature technology able to produce large amount of biohydrogen. However, this technology can compete with conventional fossil fuel (price below $2.00/kg) only if the cost of the waste biomass has a gate fee that could range anywhere from $50-$75/tonnes and the size of the gasification plant is 150 MW. Above this value the biohydrogen cost can vary between $2.80/kg (Binder et al., 2018; Shahabuddin et al., 2020). Fish and algae waste are low-cost feedstock which could thermochemically converted into low-cost hydrogen.
Biohydrogen production by biological conversion is a promising technology due to the low operating temperature and by-product formation. However, state of the art, this technology has been proven only in a laboratory-scale, and its efficiency strongly depends on the type of technology used. The highest efficiency achieved with the biological conversions is 80% using Direct Bio-Photosynthesis and 42.80% using dark fermentation.
The predicted cost to produce Biohydrogen by biological conversion are calculated by the assumption, and the hydrogen cost can change from $2.13/kg for direct photosynthesis to $7.53/kg for dark fermentation (Forrunque-Ahmed et al., 2021). Although the predicted hydrogen cost productions are comparable to the thermochemical conversion this technology is still not mature to compete for thine biohydrogen production due to technical challenges to face up.
Indeed, the most developed biochemical processes are dark fermentation (DF) (light independent) and photolysis (light dependent). They are still in the demonstration phase due to the low hydrogen production. It will be essential to reduce the reactor cost and investigate different geometry to improve the performance and reduce the production cost. The feedstock cost is also considered an important parameter in lowering the cost of hydrogen. Among all these processes, photo and dark fermentation are essential to biological hydrogen production technologies. Of the two methods mentioned above, DF is the most studied and promising technology for biohydrogen production owing to its higher production rates and treatment capacity for organic wastes. DF can convert several types of substrates, including waste products rich in carbohydrates and fatty acids. At present, the DF process is not mature, and the development at the industrial scale is limited due to the lower hydrogen yield compared to its theoretical maximum yield of 4 mol of H2 per mole of hexose, as well as the estimated costs associated with the H2 production. The production cost in a scaled-up system can be minimised by pre-treatment of substrates, enrichment of inoculum and low-cost feedstock. Dark fermentation has been suggested as more practical than the other processes as it does not require external energy to drive the process or a large surface area to capture the necessary light. It can take advantage of existing reactor technologies to utilise organic wastes as feedstock (Han and Shin, 2004; Perera et al., 2010) (Table 3).
Dark fermentation is a complex system where environmental factors and bioreactor operation conditions such as temperature, pH and H2 partial pressure control metabolic pathways of hydrogen-producing microorganisms. Furthermore, we need to consider other parameters, such as substrate types and their pre-treatment methods, bioreactor configurations, inoculum sources and enrichments that influence biohydrogen production. The complexity of the system requires that different challenges have to be overcome, such as energy balance and COD conversion and improved solid-state fermentation, which has demonstrated higher hydrogen production (Ghimire et al., 2015a, 2015b). This technology is promising to produce biohydrogen, but it still requires a lot of investigations to be economically viable on a large scale. Many researchers have documented that the H2 production from wastewater, organic waste or biomass by biochemical path has the potential to reduce H2 production costs (Das and Veziroǧlu, 2001; Chang et al., 2011).
Differently from dark fermentation, photo-fermentation and biophotolisys are two light-driven processes in which carbon sources are converted to biohydrogen using photosynthetic bacteria. A key addition to the whole process is light energy, natural or artificial. The photo-fermentation uses photosynthetic bacteria, mainly called purple non-sulphur bacteria (PNS bacteria). Rare, green bacteria and purple bacteria are used too. The light energy is used to oxidise the carbon source and produce electrons. PNS bacteria (and the other bacteria that can be used) synthesise nitrogenase or hydrogenase enzymes. The methods take place in anaerobic conditions, and nitrogenase under anaerobic conditions use electrons and ATP (Adenosine triphosphate) and produces hydrogen and ADP (Adenosine diphosphate), as described by the Eq. 1 (Rahman et al., 2016).
Operating conditions (i.e., pH, temperature and light intensity) depend on the specific bacteria used and the converted feedstock.
Biophotolysis uses microorganisms such as cyanobacteria or microalgae to produce biohydrogen from water. A key factor for this method is sunlight, which is essential for the system to make biohydrogen. Water is not the only reactant that can be used in these processes. Glucose as well as other organic matter, can be used in biophotolytic processes. This method of hydrogen production has been applied in different ways through the years, mostly at the laboratory scale. Biophotolytic processes can be categorised into two main categories: direct biophotolysis and indirect biophotolysis. The general chemical reaction that describes these two processes is given by reaction Eq. 2 (Anto et al., 2020).
In direct biophotolysis, the photosystem absorbs the light energy and transports electrons to ferredoxin, reducing the water molecule. The reduced ferredoxin can transport electrons to hydrogenase (biohydrogen-producing enzyme). After that, hydrogenase catalyses the conversion of a proton to biohydrogen, according to the reaction Eq. 3, (Mona et al., 2020).
The oxygen produced acts as an inhibitor for the hydrogenase enzyme and is a major problem in direct biophotolytic systems.
Indirect biophotolysis is a process that takes place in two stages. In the first stage, the photosynthetic system produces a large amount of biomass to increase the amount of carbohydrates. In the second stage, biomass-rich carbohydrate is utilised as carbon sources. This carbon source plays a similar role to water in direct biophotolysis. This second stage has some similarities with anaerobic fermentation processes. The advantage of indirect photolysis compared to direct photolysis is that oxygen generation is separated from the stage of hydrogen evolution, so oxygen is not inhibiting the H2 evolution (Rahman et al., 2016; Mona et al., 2020). Table 4 summarises the various biological hydrogen production processes with general technical information involved therein broad classification of microorganisms used with their relative advantages.
Photo fermentation was coupled with dark fermentation to convert residue from anaerobic digestion from fish waste into biohydrogen (Melitos et al., 2021). Ghimire et al. (2015c) reported that connecting the DF-Photo Fermentation reduces the control of operating parameters such as pH and the process impacts on the energy yield reaching 55 MJ/kg volatile solid food waste, adding a synergistic effect to the overall energy recovery during the conversion of food waste.
Biophotolysis is the main use to process hydrogen from water. The process has also been applied to wastewater and improved water quality in the aquaculture system. Malara et al. (2017) use photolysis to inactivate vibrio species (pathogens) in aquaculture.
3.3 Biodiesel and renewable diesel
One of the biggest challenges today is to produce a large amount of sustainable biofuel to decarbonise the heavy transportation sector and achieve the zero-emission target by 2050. Biodiesel and renewable diesel seem to be the more suitable fuel for their physical chemistry properties to replace fossil fuels. However, their economic and environmental sustainability is highly affected mainly by the feedstock type and the oil extraction method, which are responsible for the overall biodiesel and the catalyst used and for production methods and the catalyst. The utilization of edible oils (vegetable oil) is too costly and negatively impacts the environment and society (Sumathi et al., 2008). Utilising non-edible oil plants does not provide enough oil to satisfy the energy demand, and the extraction of the oil from the plant seed is complex (Hamza et al., 2020). Studies have shown that waste feedstock rich in oil, such as fat animal waste (e.g., fish waste) and algae, are potential renewable low-cost fuel sources of interest (Aniokete et al., 2022; Douvartzides et al., 2019; Madeen et al., 2021). Fish waste and algae waste from aquaculture are low-cost feedstock and have demonstrated that they can be easily converted into biofuel with minimal economic and environmental impact (Abomohra et al., 2018; Papargyriou et al., 2019).
Fish and algae waste are two sources rich in oils. The amount of oil contained depends on the type of fish and algae. Depending on the extraction methods, it is possible to extract up to 25 wt% of oils in fish waste, and 10 wt% and 60 wt% of oil from macroalgae and microalgae oils (Zhaohui et al., 2020; Gosch et al., 2012). The oil is extracted using several methods, such as wet rendering, enzymatic hydrolysis, autolysis, dry rendering, solvent extraction, and supercritical fluid extraction (Dumay et al., 2004; Falch et al., 2006; Rai et al., 2010; Ghaly et al., 2013; Jayasinghe et al., 2013; Oliveira et al., 2013; Suseno et al., 2015). While the algae oil is extracted using the Soxhlet apparatus and hexane solvent (Aravind et al., 2020). The studies reported showed that supercritical and microwave-assisted technologies are the most appropriate technologies to extract oil from waste. These technologies demonstrated a high oil recovery efficiency, lower cost, and high environmental sustainability (Zulqarnain et al., 2021). The purified oil can then be converted accordingly to the type of fuel desired. The extracted waste can produce third-generation biodiesel (Fatty Acid Methyl Ester) and renewable diesel (long-chain hydrocarbons). There are two main mature routes for the production of biodiesel and renewable diesel from marine, aquaculture, fish industry and algae, which are transesterification and hydrodeoxygenation (Figure 4).
The transesterification reaction of fish oil or algae oil with low molecular weight alcohols in the presence of a catalyst oil produces Biodiesel (FAME), a biodegradable fuel (Wu and Leung, 2011; Sharmila et al., 2021). The utilization of waste oil makes the production of biodiesel more economically and environmentally sustainable biodiesel (Rajendran et al., 2022). However, the process requires a high energy demand for biodiesel purification and recycling of large volumes of alcohol when it is carried out using homogeneous catalysis (Falch et al., 2006; Tanwar et al., 2013). The recent study focused on using a more sustainable method, replacing the homogenous catalyst with a heterogeneous catalyst based on alkaline Earth oxide (Marinković et al., 2016). Papargyriou et al. (2019) and Mahdavi et al. (2015). The heterogeneous catalysis showed comparable performance compared to the homogenous catalysis, but it has the advantage of reducing energy consumption and operating cost, producing high-purity biodiesel by reusing the catalyst for many cycles (Lee et al., 2014; Knothe and Razon, 2017). Enzymatic catalysts have also been investigated as possible heterogeneous catalysts for the conversion of fish waste oil with success. The utilization of enzymes allows the production of very pure biodiesel, which could further reduce the cost of biodiesel production. However, the enzymatic reactions show a slow reaction rate, and the high cost of the catalysts doesn’t make it a commercial pathway for biodiesel production (Angulo et al., 2020).
Biodiesel production is proven at a commercial scale, and the size of the plant does not affect the price of its sustainability. This means that the plant can also be scaled up accordingly with the amount of feedstocks available locally. The main problem associated with the use of biodiesel is its low oxidation stability, poor cold flow properties and low energy content. This required that biodiesel has to be blended with fossil fuel to improve its properties and can be distributed using existing infrastructure (Knothe and Razon, 2017).
Differently from biodiesel, Renewable diesel is a mixture of long-chain hydrocarbons) with a composition like diesel derived from fossil fuels. This makes green diesel highly stable due to the absence of double bonds in its structure, and more valuable than biodiesel because it has a higher cetane number and a higher heating value than FAME biodiesel and has similar fuel properties to petroleum-diesel. This means that green diesel can replace diesel-derived fossil fuel and can be delivered using existing infrastructure (Miller and Kumar, 2014).
Hydrodeoxygenation (HDO) is a catalytic chemical process that uses hydrogen to decarboxylase the methyl ester for a stable long-chain hydrocarbon with the same characteristics as diesel derived from fossil fuel. HDO of bio-oil generally occurs under high pressure (7 MPa–20 MPa) and high temperature (200°C–400°C) conditions to increase the amount of effective hydrogen in bio-oil and reduce the oxygen content, thereby ameliorating the physical and chemical properties of bio-oil. Oxygen is removed in the form of CO2 or H2O, meanwhile, the unsaturated bonds become saturated (Qu et al., 2021).
The hydrodeoxygenation process has the advantages of guaranteeing large conversion, better selectivity, high stability product. However, the process requires highly harsh conditions, a complex equipment system, blockages of the reactor due to the production of high molecular weight wax and deactivation of the catalyst (Qu et al., 2021). The catalysts are the main components of this process because they guide the performance of the process. Transition metals such as Ni, Mo, Co., Zn, Ce, Nb, Fe, and Cu, among which Ni and Mo are generally used as the main active metals (Wang et al., 2015; Hong and Wang, 2017; Yang et al., 2018). The Mo-based catalysts displayed high arene selectivity, while the Ni-based catalysts mainly saturated the aromatic ring in liquid-phase reaction conditions (high hydrogen pressure) (Zhang et al., 2020).
Renewable diesel is produced by the hydro-deoxygenation of vegetable oils, and it is a mature and certified technology at a large scale. However, some gaps still need to be filled, such as selecting the suitable and best catalyst, inexpensive feedstock and studying the mechanism of the reaction. Several studies have been conducted to prove that waste material reduces the production cost, particularly when fat animal waste is used (Toldrá-Reig et al., 2020). However, the hydrodeoxygenation process of algae waste seems not to be economically sustainable due to the highest impact on the water scarcity footprint and the unit production cost (Madeen et al., 2021).
Despite the several challenges and problems related to this technology, the Techno-economic Analysis and Life-Cycle Analysis of Renewable Diesel Fuels Produced with Waste Feedstocks carried out from Longwen et al. (2022) highlight as the use of waste feedstock is still crucial for the production costs and for the Greenhouse gas emissions. However, the main challenge for this technology is the process scalability elated to the viability of large-scale production because distributed waste resources are a well-known feature for any conversion strategy using a waste resource.
4 Production of bioderivatives from fish-aquaculture waste and algae bloom
4.1 Proteins and peptides
4.1.1 Fish hydrolysates for the generation of biologically active peptides
Fish protein hydrolysates (FPH) can be obtained from fish processing waste (skin, muscle, head, viscera, and bones), representing a good source of protein, peptides, and amino acids. FPH contain bioactive peptides with a wide range of biological activities, such as antioxidant, antimicrobial, anti-hypertensive, antitumoral, anti-inflammatory, anticoagulant, and antidiabetic. Furthermore, it has been reported that the nutritional properties of FPH are superior to other protein hydrolysates because fish proteins are abundant in essential amino acids like lysine and valine (López-Pedrouso et al., 2020; Korkmaz and Tokur, 2022). The production of bioactive peptides includes several steps involving chemical or enzymatic hydrolysis, and the purification of the hydrolysate proteins. Fish hydrolysates could be used as commercial sources of bioactivity; however, it has been reported that biological activities are superior in purified peptides. The purification of protein hydrolysates has been investigated by several techniques such as ultrafiltration (UF), ion exchange chromatography, reverse phase high-performance liquid chromatography (RP-HPLC) and gel filtration (GF) (Ishak and Sarbon, 2018).
However, purification at industrial levels could be economically feasible only for high added value products, such as peptides for pharmacology proposes, due to the high production cost.
The common treatments to recover the bioactive compounds from fish wastes are chemical and enzymatic hydrolysis (Table 5) (Idowu et al., 2021). In chemical hydrolysis, acid or alkali conditions are used at high temperatures; the process is low-cost and fast. However, the hydrolyzed products have high variations in the amino acid profile. In enzymatic hydrolysis, lower temperatures and pH values are used. Even though this process is long and expensive, enzymatic hydrolysis produces high-quality FPH. Numerous commercial enzymes have been used, such as alcalase, flavourzyme, papain, protamex, pronase (Siddik et al., 2021). Korkmaz and Tokur (2022) hydrolysed fish wastes from trout, anchovy, and whiting to produce fish proteins. They compared three commercial enzymes (alkaline protease, Protamex, and flavourzyme) and optimized the hydrolysis conditions (time, enzymatic ratio, temperature) for protein production. The highest protein content was obtained from the head and viscera of whiting (95.22%) with alkaline protease. They concluded that the degree of hydrolysis and protein recovery depends on fish species, waste composition, hydrolysis method, and enzyme. Henriques et al. (2021) characterized protein hydrolysates from the most discarded species by the North-West Spain fishing fleet (gurnard, Atlantic horse mackerel, blue whiting, red scorpionfish, pouting, and four-spot megrim). They used whole fish, skin, bones, and head and enzymatic hydrolysis using alcalase. The maximum yield of FPH and protein was achieved with the skins and bones, 97.3% of FPH yield with blue whiting, and 76.6% of protein. Also, the hydrolysates contained all the essential amino acids; glutamic and aspartic acids were the most abundant. Nevertheless, most enzymes used for hydrolysis have different specificities that are difficult to control or standardize. Subsequently, searching for proteases with higher activity and substrate specificity is essential. Further, it is important to mention that almost all the investigations have been confined to small scale because of the high cost of the enzymes (Figure 5).
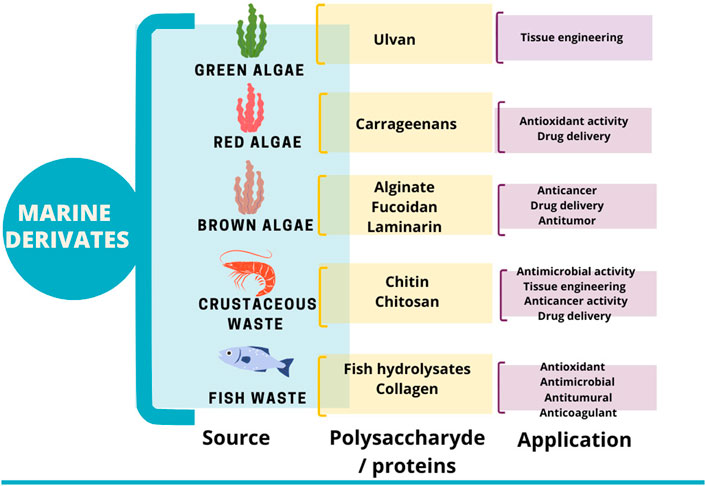
FIGURE 5. Polysaccharides and proteins extracted from aquaculture and marine wastes and their applications.
4.1.2 Collagen
Collagen is a natural material with excellent biocompatibility and biodegradability (Bai et al., 2017). There are at least 29 types of collagens, and among these, the most common is type 1. This collagen has multiple applications in the cosmetic, food, medicine, and pharmaceutical industries (Wang et al., 2018). Collagen can be obtained from pig skin, pork, cattle bones, and other mammalian sources. Nevertheless, this material is also present in marine organisms such as fish and sponges (Subhan et al., 2021). Marine collagen has multiple advantages; for example, it can be consumed by people with religious restrictions because marine animals are not affected by infectious diseases. Also, marine collagen has lower thermal denaturalization temperature than collagen from pigs or cows (Tanaka et al., 2018). In the fishing industry, only about 25% of the total fish weight is used, with 75% of waste containing skin, bone, and scale that could produce collagen (Srikanya et al., 2017). Fish is rich in type I collagen, and the yield and properties depend on the extraction method and the raw material (Table 5). Although the yield from marine collagen is lower than from mammalian sources, different studies have been done on the improvement. For collagen extraction, two principal operations have been reported. In the first one, gelatin is obtained by a chemical pre-treatment with dilute acid or alkali. Afterward, the gelatin’s enzymatic hydrolysis (using pepsin) produces the collagen peptides (Bai et al., 2017). Wang et al. (2018) used skin loach for the extraction of acid-soluble collagen (ASC) and pepsin-soluble collagen (PSC) and obtained yields of 22.42% and 27.32%, respectively. They used .5 mol/L acetic acid and pepsin (1,200 u/g) for the extraction, and the temperature of denaturalization of the obtained collagen was similar to the porcine skin collagen. Tan and Chang (2018) used catfish skins by acid, homogenization-aided, and enzymatic methods, and their recovery rate was 64.19%. However, it is difficult to separate the proteins after the alkali or acid treatment. Besides, the enzymatic process enhances the total cost of production because enzymes are expensive and cannot be reused. Therefore, new methods have been developed. Bai et al. (2017) used deep eutectic solvents for collagen extraction from cod skins. These solvents are biocompatible and environmentally friendly. Choline chloride (ChCl) was used, and under optimal conditions, the extraction efficiencies were up to 91.57%–96.01% of the total collagen peptides in only 2 h.
4.2 Polysaccharides
4.2.1 Fucoidan
Fucoidan is a polysaccharide composed of l-fucose and sulphate groups found in brown macroalgae (Luthuli et al., 2019). Ascophyllum nodusum, Undaria pinnatifida, and Ecklonia cava macroalgae have been reported as the richest sources of fucoidan (Abdel-Latif et al., 2022). The principal application of fucoidan is in the pharmaceutical industry because of its anticancer, anticoagulant, anti-inflammatory, antiproliferative, and immunomodulatory activities (Wijesinghe and Jeon, 2012). Another application of fucoidan is in aquaculture. Multiple authors have reported using fucoidan in the dietary supplementation of aquafeed. Fucoidan could be used as a growth promotor, antiviral agent, antioxidant, toxicity modulator, and to improve resistance against bacterial pathogens (Abdel-Latif et al., 2022). Regarding fucoidan production, the extraction consists of an initial purification using alcohol, an extraction step using acid, and final precipitation using ethanol (Fletcher et al., 2017). However, environmental technologies such as microwave-assisted and enzyme-assisted extraction have also been reported. For example, Ptak et al. (2019) used a microwave-assisted extraction to improve the fucoidan yield from Fucus genus. They found that fucoidan yield is maximized by extraction with 10 mM sulfuric acid, obtaining a yield of 11.10%. Tang et al. (2022) used an enzymatic-assisted extraction using a cocktail of cellulose, and they reported a yield of 4.74% for fucoidan extraction from Kjellmaniella crassifolia. Higher yields have been reported with acidic extractions. Zhang and Row (2015b) identified the best condition for fucoidan extraction from Laminaria japonica, and they obtained a yield of 17 wt% with an acidic treatment using HCl. According to season, specie, maturity and location, it has been reported that fucoidan structure is different. Fletcher et al. (2017) studied the seasonal variation of three species of brown macroalgae: Fucus serratus (FS), Fucus vesiculosus (FV), and Ascophyllum nodosum (AN) harvested on the coastal of Aberystwyth United Kingdom. The average content was 6 wt%–9.8 wt%, with the highest content in autumn. Abraham et al. (2019) developed and optimized a process for the extraction of multiple polysaccharides (fucoidan, alginate, and laminarin) from Durvillaea potatorum (giant bull kelp), using an acidic extraction before the conventional alkali extraction. They reported a higher yield (43.53% w/w) of the total polysaccharides compared with the traditional process for a single alginate product (38.97% w/w).
4.2.2 Alginate
Alginate is the primary cell wall component in brown seaweeds (17%–45% of the dry weight), and it is a polysaccharide formed by two monomeric units [β-D mannuronic acid (M) and α-L guluronic acid (G)] (Abraham et al., 2019; Bojorges et al., 2022). The algae variety and growing conditions affect the structure and composition. The principal sources of alginate are Macrocystis pyrifera from California and Ascophyllum nodosum from the North Atlantic (López-Pedrouso et al., 2020). Alginate is broadly used in different fields such as the cosmetic, pharmaceutical, food, and textile industries due to its gelling, film-forming, and emulsifying properties (Gomaa et al., 2018). It has also been reported that alginate has potential prebiotic activity and can decrease cholesterol levels (López-Pedrouso et al., 2020). Alginate production follows an acid and alkaline treatment by a precipitation or flocculation method, principally by adding sodium chloride and isopropanol. Also, some authors have reported a pre-treatment with formaldehyde (Table 5). For example, Mohammed et al. (2020) extract alginate from Sargassum seaweed using formaldehyde, acid treatment with sulfuric acid, and alkali extraction. They used a response surface methodology to optimize the extraction process; the optimum conditions were Na2CO3 3.75% (w/v) for 6 h at 80°C, with a yield of 21.21%. Even when formaldehyde is used to improve the quality of the polysaccharides or as a preservative, it should be considered toxic, allergenic, and possibly carcinogenic. For these reasons, diverse authors have designed extraction technologies without formaldehyde. Bojorges et al. (2022) extracted alginate from Saccharina latissima and Ascophyllum nodosum. Produced alginates had a comparable composition as commercial alginates; also, after the first treatment, a high potential as a bioactive ingredient was found due to a high content of sulphated fucoidan and polyphenols. Gomaa et al. (2018) extracted alginate from Sargassum latifolium using citric acid for an acid extraction, followed by an alkali treatment using Na2CO3. Under the extraction conditions, the yield of alginate was 44.26%.
4.2.3 Chitin and chitosan
Chitin is a linear polysaccharide formed mainly of β- (1 → 4)- linked 2-acetamido-2-deoxy-β-d-glucopyranose units and partially of β-(1 → 4)-linked 2-amino-2- deoxy-β-d-glucopyranose (El Knidri et al., 2018). It is the second most abundant natural biopolymer, found in fungi, insects, algae, and crustaceans (Lionetto and Corcione, 2021). The composition of chitin is different between organisms. Three crystalline forms, α-, β-, and γ-chitin, differ in orientation, degree of orientation, number of chains, and unit size. In crustaceans and algae, the α-chitin crystalline structure is the most abundant form. This structure is the most stable and has a remarkable stability (Pellis et al., 2022). Advantages such as biodegradability, biocompatibility, and non-toxicity make chitin and its derivative chitosan widely used in medicine, pharmacy, textiles, cosmetic, and food industries (Marzieh et al., 2019).
Crustaceans are the principal source of chitin extraction. Crustacean’s shells contain 20%–30% of chitin, 30%–40% of proteins, 30%–50% of calcium carbonate (Kumari et al., 2015). However, it has been reported that shrimp has a higher percentage of chitin and is a more suitable choice than crabs, in terms of high average molecular weight. Generally, the extraction process is chemical or biological and involves three major steps: demineralization, deproteinization and deacetylation. In the chemical extraction, the demineralization is done by acid treatment using HCl, HNO3, and H2SO4, the deproteinization by alkaline treatment using NaOH or KOH, and the deacetylation by alkaline treatment using a strong NaOH or KOH. The principal advantages of this extraction are a short processing time and a high depolymerization degree of the final product, making them the method used on an industrial scale. However, it is considered environmentally unfriendly due to the solvents employed. Biological extraction treatments are an alternative, using lactic acid in the demineralization, proteases in the deproteinization, and chitin deacetylase for the deacetylation (El Knidri et al., 2018). Pérez et al. (2022) developed an eco-friendly process for chitosan production from waste shrimp shells. They used citric acid and enzymatic deproteinization using papain and bromelain, and their process had a 24% lower water consumption than the traditional process. In addition, an increment of 10% in chitosan yield was achieved. Marzieh et al. (2019) used a biological extraction using lactic-acid and proteolytic enzymes, followed by mild alkali treatment. The combination of these treatments exhibited a yield of 32.12% for the chitosan, making it a good strategy as a greener method for chitosan extraction.
4.2.4 Other polysaccharides: Carrageenan, laminarin, and ulvan
Carrageenan, a sulphated polysaccharide in the cell wall of red seaweeds (30%–75% of dry weight), is formed by alternate units of d-galactose and 3,6-anhydrogalactose. Carrageenan is principally produced from the red seaweed species Eucheuma, Gigartina, Chondrus, and Hypnea. At least 15 different structures have been reported. However, the most relevant are κ-, λ-, and i-. The structure could be modified depending on the seaweed species, growing conditions, and the extraction process (Rudke et al., 2020). Because of their properties, such as gelling and hydrocolloid, they are mainly used in the food industry. Additionally, several biological activities have been reported to exhibit, such as anti-HIV, antithrombotic, anticoagulant, anticancer, and antioxidant (Qureshi et al., 2019). Moreover, properties such as biocompatibility and high adsorption of water made it a good candidate for drug delivery systems (Khan et al., 2017). The common extraction method is under hot alkaline conditions, followed by filtration and alcohol precipitation (Qureshi et al., 2019). However, greener methods have been reported in recent years, such as microwave-assisted extraction, ultrasound-assisted extraction, photobleaching, enzyme-assisted extraction, and pressurized solved extraction (Rudke et al., 2020).
Ulvan is a sulphated polysaccharide extracted from the green algae “Ulva lactuca.” This polysaccharide possesses attractive gelling and antioxidant properties (Guidara et al., 2019; Kidgell et al., 2019). Also, other biological activities, including immunomodulating, anticancer, and antiviral, have been reported. Consequently, ulvan is increasing its interest as a constituent in biomedical products and agriculture (Konasani et al., 2018). Similarly, to other polysaccharides, the yield and quality depend on the extraction and purification procedure and the source. Guidara et al. (2019) compared chemical extraction with enzymatic chemical extraction. They reported a high ulvan yield after the enzymatic chemical extraction 17.95%, whereas the yield was 14.22% with the chemical extraction.
Laminarin is a polysaccharide in brown macroalgae and represents up to 35% of dry weight. However, the average content of laminarin for different species of brown macroalgae is 10% (Rocher et al., 2021). It is a β-1,3-D-glucan that consists of approximately 25 glucosyl residues (Abraham et al., 2019; López-Pedrouso et al., 2020; Sterner and Gröndahl, 2021). There are variations in the structure due to the specie, season, and other environmental factors. Contrasting the other polysaccharides, laminarin does not form gels. Nevertheless, it has been reported their anti-inflammatory, anticoagulant, antitumoral and antioxidant activities (López-Pedrouso et al., 2020).
4.3 Biosurfactants
Surfactants are amphiphilic compounds, composed of so-called hydrophilic head and lipophilic tail that have the ability to reduce surface and interfacial tensions by accumulating at the interface and forming larger structures called micelles, in the first case, and surfactant liquid crystals, in the second (Kronberg and Lindman, 2014). They fall into four categories (neutral, anionic, cationic, and zwitterionic) depending on the nature of their hydrophilic fragment, the lipophilic fragment remaining structurally similar across all categories of surfactants. Gemini surfactants are a separate category of surfactants constituted of two hydrophilic head groups of any type and a single lipophilic tail group.
Most industrially relevant surfactants have found applications in the detergent, cosmetics, emulsification, and anti-foaming industries (Chang, 2016) and are widely derived from petroleum. In order to meet the ever-increasing demand for surfactants as petroleum reserves run out, biosurfactants have emerged as ideal alternatives. They have the characteristic overall amphiphilic structure of synthetic surfactants but vary at the hydrophilic head group, which can be one of several usual motifs encountered in natural molecules.
These are naturally synthesised by microbial sources such as bacteria (typically Bacillus sp. (Sakr et al., 2021), Pseudomonas sp. (Bhosale et al., 2019)., Botyris cinerea (Abidi et al., 2008), Lactobacilli (Gudiña et al., 2011), and Candida utilis (Ribeiro et al., 2019) from renewable sources and perform equally to better than synthetic surfactants in several areas (e.g., oil spill, oil recovery, wastewater treatment, and pharmaceuticals) (Ng et al., 2022). Apart from being more environmentally friendly than their current counterparts, they also present many desirable features such as being less toxic (Akbari et al., 2018), biodegradable (Vijayakumar and Saravanan, 2015), highly specific and effective at extreme temperature, salinity, and pH conditions (Pacwa-Płociniczak et al., 2011). Certainly, their main selling point is that they can be produced at a lower cost from waste (Sáenz-Marta et al., 2015; Martins and Martins, 2018; Jiménez-Peñalver et al., 2020). However, this is conditional on the isolation of the biosurfactant, as the purification process may still represent the main contribution to the overall production cost (Mukherjee et al., 2006) and identifying suitable organic waste material is crucial for the commercialization of biosurfactant. Additionally, parameters such as carbon and nitrogen sources, trace elements, temperature and pH are other variables which can strongly influence the biosurfactant yield (Patel and Desai, 1997).
Biosurfactant production by bacteria or enzymes has been investigated with all sorts of plant biomass and food industry wastes (Makkar et al., 2011; Mohanty et al., 2021). Alternatively, marine environments host a rich diversity of organisms that naturally produce biosurfactants and emulsifiers (Rahman et al., 2019), some of which are already widely used in the food industry (Liao et al., 2021). In general, biosurfactants from marine environments are derived from a wide range of organisms including, but not limited to, macroalgae, microalgae, bacteria, diatoms, and cyanobacteria (Silva et al., 2012). Algae are the most abundant resource in the ocean and represent a virtually endless source of biosurfactants called polysaccharides (Xu et al., 2017). These long polymers [tens to hundreds of kDa (Usman et al., 2017)] occur as the main structural components of marine algae or as microbial secretions, known as exopolysaccharides (EPS) (Manivasagan and Kim, 2014). Polysaccharides can be extracted with hot water (Zhang et al., 2010; Savage, 2012), although removing unwanted species with organic solvents is more efficient and less costly (Lim et al., 2014). Lately, these methods have been replaced with microwave, ultrasonic, and enzyme-assisted extraction. Microwave-assisted extraction has the advantages of short extraction time, low energy, and low cost (Sousa et al., 2010; Hahn et al., 2012; Kadam et al., 2013). Ultrasonication and enzymes are used to break down the cell walls and release the compounds of interest either by cavitation and diffusion through cell walls or by hydrolysis of some of the cell wall structures (Kadam et al., 2013). All these techniques have proved to have higher efficiency compared with conventional methods (Yuan and Macquarrie, 2015). To a lesser extent, liquid extraction methods have been improved using more environment-friendly solvents such as supercritical fluids (Herrero and Ibáñez, 2015) and ionic liquids (Kadam et al., 2013; Martins et al., 2016). Algal polysaccharides have found widespread applications in the food industry as emulsifiers and thickening and stabilizing agents, cosmetics, biomedical industry due to their anti-cancer, anti-viral, anti-inflammatory and antioxidant activities, and heavy-metal removal among others (Anestopoulos et al., 2020).
Exopolysaccharides (EPS), or extracellular polysaccharides, are complex mixtures of anionic biopolymers consisting primarily of polysaccharides as well as proteins, nucleic acids, lipids, and humic substances (Manivasagan and Kim, 2014). The huge variety of organisms (e.g., macro-/microalgae, bacteria) that produce EPS combined with the sometimes drastically different environment in which they grow has been an endless source of new biopolymers with unique properties. This comes to the price of a challenging isolation and purification of the target compounds, as exopolysaccharides are secreted along with other extracellular polymeric substances (Suresh Kumar et al., 2007; Manivasagan and Kim, 2014). Therefore, EPS may exhibit properties and functions that are actually reflected by the collective extracellular polymeric substances’ characteristics of a mixture of compounds (Xiao and Zheng, 2016). In particular, sulphated polysaccharides are a category of biosurfactants exclusively found in the marine environments with additional properties that structural polysaccharides do not possess, owing to the multiple sulphate functional groups, and that slightly vary depending on the degree of sulphation of the polymers (Silva et al., 2012; Raposo et al., 2013). These are as diverse as joint lubrication and targeted drug delivery.
Another non-negligible potential feedstock for biosurfactant production is fish wastes as sources of both peptide and fatty substrates. With 30%–80% of the fish body weight being discarded during industrial processing operations (Dave and Manuel, 2014), the upcycling of these wastes also contributes to solving environmental and health problems. Zhu et al. (2020) took advantage of the richness of these wastes to synthesize lipopeptides. They first hydrolysed blended fish heads and fish livers to produce peptones that Bacillus subtilis transformed into lipopeptides. The lipopeptides were used in the formulation of a bio-dispersant that exhibited particularly good behaviour to treat oil spills. Similarly, Hu et al. (2021) enzymatically hydrolysed tuna fish red meat to obtain peptones that were converted into biosurfactants by Bacillus subtillis. They found that on a laboratory scale, the surface tension and critical micelle dilution were similar to those obtained by Zhu et al. (2020) but insist that results may vary depending on the fish source. Their process was scaled up to a 100-L pilot-scale, which is to date the most advanced process for the production of biosurfactant from fish materials. The same bacteria Bacillus subtilis was used to prepare lipopeptides in 30% yield from more refined materials, such as fish oil and a culture broth (Saranya et al., 2014). They were then immobilized on nanoporous activated carbon and effectively removed Ca2+ (98%) and Cr3+ (92%) from water solutions. By using Ustilago maydis FBD12 instead of Bacillus subtillis. Cortes-Sánchez et al. (2011) produced glycolipids from soybean and fish oils. In optimal conditions, the production of glycolipids was higher with fish oil. Moreover, the production of glycolipids from soybean oil was higher when Candida rugosa (lipase) was added, while this revealed detrimental when fish oil was used, suggesting that fish oil may be a better raw material. Finally, Kaskatepe et al. (2015) used Pseudomonas aeruginosa strains to convert fish meal into rhanmopeptides. The three strains tested (ATCC, H1, and SY1) produced biosurfactants at concentrations of 12.3 g/L, 9.3 g/L, and 10.3 g/L respectively, which decreased when the bacteria were exposed to UV light. Kadam and Savant (2019) compared the production of glycolipids from shrimp shell and fish wastes and plant biomass by Pseudomonas stutzeri. Curiously, fish wastes gave by far the worst yield while shrimp shell wastes were the best substrate and could produce 4 g/L–6 g/L of sucrose-based glycolipids under the optimized conditions. When fish wastes were transformed with Corynebacterium spp. CCT, anionic biosurfactants were produced (Martins and Martins, 2018). When compared to sugarcane bagasse, petroleum sludge, and glycerol, both fish wastes and sugarcane bagasse significantly outperformed the last two feedstocks. Despite the more industrially viable production of artificial surfactants, there seems to be a trend according to which biosurfactant production is higher when unrefined feedstocks from sources other petroleum are used. These combinations of sustainable feedstocks and natural microorganisms is economically promising as they valorise cheap wastes that would otherwise create environmental and health problems, without loss of efficiency compared with artificial surfactants.
5 Conclusion and future prospect
Due to the aquaculture sector is growing fast, it is necessary to implement new technologies to take advantage of the waste generated. Improving waste utilization in aquaculture is necessary to minimize its environmental impact. It has been reported that aquaculture by-products possess multiple properties that make them useful in different fields, such as energy, medicine, and food. Biofuels from aquaculture and marine waste, and macroalgae represent a promising alternative to carbon fuels because they do not compete with food crops for arable land such as the first and second generation of biofuels. Also, the cost of biofuels could be less because marine waste and macroalgae are inexpensive sources. Nowadays, most biofuels cannot compete with fossil fuels and most of them are on a laboratory scale that needs to solve challenges related to large-scale production and yields. Proteins and polysaccharides isolated from marine and aquaculture waste have shown multiple biological and physiological properties. The recovery processes for these biomolecules are now focused on enzyme-assisted processes than chemical-based processes due to their eco-friendly and green aspects. Although there are different alternatives for by-product management, as we discussed before, most of them remain in the development phase or optimization. So, future research must focus on can scale these processes to an industrial level. With this article, we hope to encourage the use of aquaculture and marine waste for the sustainable production of biofuels and bioderivates on a circular and sustainable strategy. Several applications for the by-product of aquaculture and algae are on the table. However, countries and industries must invest in infrastructure and technology for by-product utilization and monitoring the positive impact on that. With investment, by-products will contribute to the sustainable development of the society. The production of different useful products in one refinery could be a more advantageous project rather than the exclusive production of only one product reducing the cost. To secure and guarantee food safety, the integration of aquaculture and marine waste is essential as an alternative on the reuse of nutrients along the productive chains for a sustainable future in next the green world.
Author contributions
LA-R: Write original draft, BS-R: Write original draft; GP: Write original draft; JES-H: Write original draft; HMNI: Review-editing; RP-S: Supervision, Review-editing, Funding acquisition; ADB: Write original draft, Review-editing, Funding acquisition; EMM-M: Write original draft, Review-editing, Supervision.
Funding
This work received the financial support of the project GCRFNGR4/1388 "Algae bloom: waste resource for aquaculture and bioenergy industry in Mexico". This work was supported by the World Universities Network, Research Development Fund.
Acknowledgments
The authors acknowledge the support of the participant institutions for gaining access to scientific journal databases and GCRF to fund the project. CONACyT is thankfully acknowledged for partially supporting this work under Sistema Nacional de Investigadores (SNI) program awarded to EM-M (CVU: 230784), JS-H (CVU: 375202), HI (CVU: 735340), and RP-S (CVU: 35753).
Conflict of interest
The authors declare that the research was conducted in the absence of any commercial or financial relationships that could be construed as a potential conflict of interest.
Publisher’s note
All claims expressed in this article are solely those of the authors and do not necessarily represent those of their affiliated organizations, or those of the publisher, the editors and the reviewers. Any product that may be evaluated in this article, or claim that may be made by its manufacturer, is not guaranteed or endorsed by the publisher.
References
Abdel-Latif, H. M. R., Dawood, M. A. O., Alagawany, M., Faggio, C., Nowosad, J., and Kucharczyk, D. (2022). Health benefits and potential applications of fucoidan (FCD) extracted from Brown seaweeds in aquaculture: An updated review. Fish. Shellfish Immunol. 122, 115–130. doi:10.1016/j.fsi.2022.01.039
Abidi, F., Limam, F., and Nejib, M. M. (2008). Production of alkaline proteases by Botrytis cinerea using economic raw materials: Assay as biodetergent. Process Biochem. 43, 1202–1208. doi:10.1016/j.procbio.2008.06.018
Abomohra, A. E., El-Naggar, A. H., and Baeshen, A. A. (2018). Potential of macroalgae for biodiesel production: Screening and evaluation studies. J. Biosci. Bioeng. 125, 231–237. doi:10.1016/j.jbiosc.2017.08.020
Abraham, R. E., Su, P., Puri, M., Raston, C. L., and Zhang, W. (2019). Optimisation of biorefinery production of alginate, fucoidan and laminarin from Brown seaweed Durvillaea potatorum. Algal Res. 38, 101389. doi:10.1016/j.algal.2018.101389
Achinas, S., Achinas, V., and Euverink, G. J. W. (2017). A technological overview of biogas production from biowaste. Engineering 3, 299–307. doi:10.1016/J.ENG.2017.03.002
Ahmad, A., Sheikh Abdullah, S. R., Hasan, H. A., Othman, A. R., and Ismail, N. I. (2021). Aquaculture industry: Supply and demand, best practices, effluent and its current issues and treatment technology. J. Environ. Manage. 287, 112271. doi:10.1016/j.jenvman.2021.112271
Ahrens, T., Drescher-Hartung, S., and Anne, O. (2017). “Sustainability of the biowaste utilization for energy production,” in Biomass volume estimation and valorization for energy. Editors J. S. Tumuluru London: IntechOpen, 166–185.
Akbari, S., Abdurahman, N. H., Yunus, R. M., Fayaz, F., and Alara, O. R. (2018). Biosurfactants—a new frontier for social and environmental safety: A mini review. Biotechnol. Res. Innov. 2, 81–90. doi:10.1016/j.biori.2018.09.001
Al-Manhel, A. J., Al-Hilphy, A. R. S., and Niamah, A. K. (2018). Extraction of chitosan, characterisation and its use for water purification. J. Saudi Soc. Agric. Sci. 17, 186–190. doi:10.1016/j.jssas.2016.04.001
Alavi-Borazjani, S. A., Capela, I., and Tarelho, L. A. C. (2019). Dark fermentative hydrogen production from food waste: Effect of biomass ash supplementation. Int. J. Hydrogen Energy. 44, 26213–26225. doi:10.1016/j.ijhydene.2019.08.091
Alexander, K. A., Angel, D., Freeman, S., Israel, D., Johansen, J., Kletou, D., et al. (2016). Improving sustainability of aquaculture in europe: Stakeholder dialogues on integrated multi-trophic aquaculture (IMTA). Environ. Sci. Policy. 55, 96–106. doi:10.1016/j.envsci.2015.09.006
Ali, A. M. M., Benjakul, S., Prodpran, T., and Kishimura, H. (2018). Extraction and characterisation of collagen from the skin of golden carp (probarbus jullieni), a processing by-product. Waste Biomass Valorization 9, 783–791. doi:10.1007/s12649-017-9841-0
Allen, E., Wall, D. M., Herrmann, C., Xia, A., and Murphy, J. D. (2015). What is the gross energy yield of third-generation gaseous biofuel sourced from seaweed? Energy 81, 352–360. doi:10.1016/j.energy.2014.12.048
Alzate-Gaviria, L. M., Sebastian, P. J., Pérez-Hernández, A., and Eapen, D. (2007). Comparison of two anaerobic systems for hydrogen production from the organic fraction of municipal solid waste and synthetic wastewater. Int. J. Hydrogen Energy. 32, 3141–3146. doi:10.1016/j.ijhydene.2006.02.034
Anestopoulos, I., Kiousi, D. E., Klavaris, A., Maijo, M., Serpico, A., Suarez, A., et al. (2020). Marine-Derived surface active agents: Health-promoting properties and blue biotechnology-based applications. Biomolecules 10, 885. doi:10.3390/biom10060885
Angulo, B., Fraile, J. M., Gil, L., and Herrerías, C. I. (2020). Comparison of chemical and enzymatic methods for the transesterification of waste fish oil fatty ethyl esters with different alcohols. ACS Omega 5, 1479–1487. doi:10.1021/acsomega.9b03147
Aniokete, T. C., Sadare, O. O., and Daramola, M. O. (2022). “Prospects of biodiesel production from waste animal fats,” in Waste and biodiesel (Elsevier), 17.
Anto, S., Mukherjee, S. S., Muthappa, R., Mathimani, T., Deviram, G., Kumar, S. S., et al. (2020). Algae as green energy reserve: Technological outlook on biofuel production. Chemosphere 242, 125079. doi:10.1016/j.chemosphere.2019.125079
Araujo, J., Sica, P., Costa, C., and Márquez, M. C. (2021). Enzymatic hydrolysis of fish waste as an alternative to produce high value-added products. Waste Biomass Valorization 12, 847–855. doi:10.1007/s12649-020-01029-x
Aravind, S., Barik, D., Ragupathi, P., and Vignesh, G. (2020). Investigation on algae oil extraction from algae Spirogyra by Soxhlet extraction method. Mat. Today Proc. 43, 308–313. doi:10.1016/j.matpr.2020.11.668
Ariunbaatar, J., Panico, A., Esposito, G., Pirozzi, F., and Lens, P. N. L. (2014). Pretreatment methods to enhance anaerobic digestion of organic solid waste. Appl. Energy. 123, 143–156. doi:10.1016/j.apenergy.2014.02.035
Azwar, E., Wan Mahari, W. A., Rastegari, H., Tabatabaei, M., Peng, W., Tsang, Y. F., et al. (2022). Progress in thermochemical conversion of aquatic weeds in shellfish aquaculture for biofuel generation: Technical and economic perspectives. Bioresour. Technol. 344, 126202. doi:10.1016/j.biortech.2021.126202
Bai, C., Wei, Q., and Ren, X. (2017). Selective extraction of collagen peptides with high purity from cod skins by deep eutectic solvents. ACS Sustain. Chem. Eng. 5, 7220–7227. doi:10.1021/acssuschemeng.7b01439
Bartocci, P., Zampilli, M., Liberti, F., Pistolesi, V., Massoli, S., Bidini, G., et al. (2020). LCA analysis of food waste co-digestion. Sci. Total Environ. 709, 136187. doi:10.1016/j.scitotenv.2019.136187
Bhosale, S. S., Rohiwal, S. S., Chaudhary, L. S., Pawar, K. D., Patil, P. S., and Tiwari, A. P. (2019). Photocatalytic decolorization of methyl violet dye using Rhamnolipid biosurfactant modified iron oxide nanoparticles for wastewater treatment. J. Mat. Sci. Mat. Electron. 30, 4590–4598. doi:10.1007/s10854-019-00751-0
Binder, M., Kraussier, M., Kuba, M., and Luisser, M. (2018). Hydrogen for biomass gasification. IEA Bioenergy. Available at: https://www.ieabioenergy.com/wp-content/uploads/2019/01/Wasserstoffstudie_IEA-final.pdf.
Bojorges, H., Fabra, M. J., López-Rubio, A., and Martínez-Abad, A. (2022). Alginate industrial waste streams as a promising source of value-added compounds valorization. Sci. Total Environ. 838, 156394. doi:10.1016/j.scitotenv.2022.156394
Briand, X., and Morand, P. (1997). Anaerobic digestion of Ulva sp.1. Relationship between Ulva composition and mechanization. J. Appl. Phycol. 9, 511–524. doi:10.1023/a:1007972026328
Buck, B. H., Troell, M. F., Krause, G., Angel, D. L., Grote, B., and Chopin, T. (2018). Carbon stable isotope values in plankton and mussels reflect changes in carbonate chemistry associated with nutrient enhanced net production. Front. Mar. Sci. 5, 1–15. doi:10.3389/fmars.2018.00043
Bücker, F., Marder, M., Peiter, M. R., Lehn, D. N., Mendoça-Esquerdo, V., de Almeida Pinto, L. A., et al. (2020). Fish waste: An efficient alternative to biogas and methane production in an anaerobic mono-digestion system. Renew. Energy 147, 798–805. doi:10.1016/j.renene.2019.08.140
Burton, F. L., Stensel, H. D., and Tchobanoglous, G. (2014). Wastewater engineering: Treatment and resource recovery. New York: McGraw-Hill.
Cadavid-Rodríguez, L. S., Vargas-Muñoz, M. A., and Plácido, J. (2019). Biomethane from fish waste as a source of renewable energy for artisanal fishing communities. Sustain. Energy Technol. Assessments 34, 110–115. doi:10.1016/j.seta.2019.05.006
Cao, L., Yu, I. K. M., Xiong, X., Tsang, D. C. W., Zhang, S., Clark, J. H., et al. (2020). Biorenewable hydrogen production through biomass gasification: A review and future prospects. Environ. Res. 186, 109547. doi:10.1016/j.envres.2020.109547
Chang, P. L., Hsu, C. W., Lin, C. Y., and Hsiung, C. M. (2011). Constructing a new business model for fermentative hydrogen production from wastewater treatment. Int. J. Hydrogen Energy. 36, 13914–13921. doi:10.1016/j.ijhydene.2011.03.066
Chang, Q. (2016). “Surface of solution,” in Colloid and interface chemistry for water quality control. Editor Q Chang (Academic Press), 161.
Chinh, N. T., Manh, V. Q., Trung, V. Q., Lam, T. D., Huynh, M. D., Tung, N. Q., et al. (2019). Characterization of collagen derived from tropical freshwater carp fish scale wastes and its amino acid sequence. Nat. Prod. Commun. 14, 1934578X1986628. doi:10.1177/1934578X19866288
Choe, U., Mustafa, A. M., Lin, H., Choe, U., and Sheng, K. (2020). Anaerobic co-digestion of fish processing waste with a liquid fraction of hydrothermal carbonization of bamboo residue. Bioresour. Technol. 297, 122542. doi:10.1016/j.biortech.2019.122542
Correia, M., Costa-Azevedo, I., Peres, H., Oliva-Teles, A., and Almeida, R. (2020). Integrated multi-trophic aquaculture: A laboratory and hands-on experimental activity to promote environmental sustainability awareness and value of aquaculture products. Sec. Mar. Fish. Aquac. Living Resour. 7, 156. doi:10.3389/fmars.2020.00156
Cortes-Sánchez, A., Hernández-Sánchez, H., and Jaramillo-Flores, M. (2011). Production of glycolipids with antimicrobial activity by Ustilago maydis FBD12 in submerged culture. Afr. J. Microbiol. Res. 5, 17. doi:10.5897/AJMR10.814
Dang, B. T., Bui, X. T., Tran, D. P., Ngo, H. H., Nghiem, L. D., Nguyen, P. T., et al. (2022). Current application of algae derivatives for bioplastic production: A review. Bioresour. Technol. 347, 126698. doi:10.1016/j.biortech.2022.126698
Das, D., and Veziroǧlu, T. N. (2001). Hydrogen production by biological processes: A survey of literature. Int. J. Hydrogen Energy. 26, 13–28. doi:10.1016/S0360-3199(00)00058-6
Dascomb, J., Krothapalli, A., and Fakhrai, R. (2013). Thermal conversion efficiency of producing hydrogen enriched syngas from biomass steam gasification. Int. J. Hydrogen Energy 38, 27. doi:10.1016/j.ijhydene.2013.07.022
Dave, D., and Manuel, H. (2014). The potential of fish processing wastes for biodiesel production. Available at: https://mmsb.nl.ca/wp-content/uploads/2021/01/The-Potential-of-Fish-Processing-Wastes-for-Biodiesel-Production_Dave-Manuel_2014.pdf (Accessed December 17, 2022).
Douvartzides, S. L., Charisiou, N. D., Papageridis, K. N., and Goula, M. A. (2019). Green diesel: Biomass feedstocks, production technologies, catalytic research, fuel properties and performance in compression ignition internal combustion engines. Energies 12, 5. doi:10.3390/en12050809
Duman, G., Uddin, M. A., and Yanik, J. (2014). Hydrogen production from algal biomass via steam gasification. Bioresour. Technol. 166, 24–30. doi:10.1016/j.biortech.2014.04.096
Dumay, J., Barthomeuf, C., and Berge, J. P. (2004). How enzymes may be helpful for upgrading fish by-products: Enhancement of fat extraction. J. Aquat. Food Prod. Technol. 13, 69–84. doi:10.1300/J030v13n02_07
Eiroa, M., Costa, J. C., Alves, M. M., Kennes, C., and Veiga, M. C. (2012). Evaluation of the biomethane potential of solid fish waste. Waste Manag. 32, 1347–1352. doi:10.1016/j.wasman.2012.03.020
El Knidri, H., Belaabed, R., Addaou, A., Laajeb, A., and Lahsini, A. (2018). Extraction, chemical modification and characterization of chitin and chitosan. Int. J. Biol. Macromol. 120, 1181–1189. doi:10.1016/j.ijbiomac.2018.08.139
El-Dalatony, M. M., Zheng, Y., Ji, M. K., Li, X., and Salama, E. S. (2020). Metabolic pathways for microalgal biohydrogen production: Current progress and future prospectives. Bioresour. Tech. 318, 124253. doi:10.1016/j.biortech.2020.124253
Estevez, M. M., Tomczak-Wandzel, R., and Kvamme, K. (2022). Fish sludge as a co-substrate in the anaerobic digestion of municipal sewage sludge- maximizing the utilization of available organic resources. EFB Bioeconomy J. 2, 100027. doi:10.1016/j.bioeco.2022.100027
Falch, E., Rustad, T., and Aursand, M. (2006). By-products from gadiform species as raw material for production of marine lipids as ingredients in food or feed. Process Biochem. 41, 666–674. doi:10.1016/j.procbio.2005.08.015
FAO (2022b). Food loss and waste in fish value chains. Available at: https://www.fao.org/flw-in-fish-value-chains/resources/articles/the-importance-of-food-safety-in-food-loss-and-waste-reduction/en/(Accessed September 15, 2022).
FAO (2020). Sustainability in action. Available at: https://www.fao.org/documents/card/en/c/ca9229en/(Accessed September 15, 2022).The state of world fisheries and aquaculture 2020
FAO (2022a). Towards blue transformation, a vision for transforming food systems. Available at: https://www.fao.org/state-of-fisheries-aquaculture (Accessed September 15, 2022).
Fletcher, H. R., Biller, P., Ross, A. B., and Adams, J. M. M. (2017). The seasonal variation of fucoidan within three species of Brown macroalgae. Algal Res. 22, 79–86. doi:10.1016/j.algal.2016.10.015
Forruque-Ahmed, S., Rafa, N., Mofijur, M., Anjum-Badruddin, I., Inayat, A., Sawkat-Ali, M., et al. (2021). Biohydrogen production from biomass sources: Metabolic pathways and economic analysis. Front. Energy Res. 9. doi:10.3389/fenrg.2021.753878
Franchi, G., Capocelli, M., De Falco, M., Piemonte, V., and Barba, D. (2020). Hydrogen production via steam reforming: A critical analysis of mr and rmm technologies. Membr. (Basel) 10, 10. doi:10.3390/membranes10010010
Ghaly, A. E., Ramakrishnan, V. V., Brooks, M. S., Budge, S. M., and Dave, D. (2013). Fish processing wastes as a potential source of proteins, amino acids and oils: A critical review. J. Microb. Biochem. Technol. 5, 4. doi:10.4172/1948-5948.1000110
Ghimire, A., Frunzo, L., Pirozzi, F., Trably, E., Escudie, R., Lens, P. N. L., et al. (2015a). A review on dark fermentative biohydrogen production from organic biomass: Process parameters and use of by-products. Appl. Energy . 144, 73–95. doi:10.1016/j.apenergy.2015.01.045
Ghimire, A., Frunzo, L., Pontoni, L., d’Antonio, G., Lens, P. N. L., Esposito, G., et al. (2015b). Dark fermentation of complex waste biomass for biohydrogen production by pretreated thermophilic anaerobic digestate. J. Environ. Manage. 152, 43–48. doi:10.1016/j.jenvman.2014.12.049
Ghimire, A., Valentino, S., Frunzo, L., Trably, E., Escudié, R., Pirozzi, F., et al. (2015c). Biohydrogen production from food waste by coupling semi-continuous dark-photofermentation and residue post-treatment to anaerobic digestion: A synergy for energy recovery. Int. J. Hydrogen Energy. 40, 16045–16055. doi:10.1016/j.ijhydene.2015.09.117
Gomaa, M., Fawzy, M. A., Hifney, A. F., and Abdel-Gawad, K. M. (2018). Use of the Brown seaweed Sargassum latifolium in the design of alginate-fucoidan based films with natural antioxidant properties and kinetic modeling of moisture sorption and polyphenolic release. Food Hydrocoll. 82, 64–72. doi:10.1016/j.foodhyd.2018.03.053
Gosch, B., Magnusson, M., Paul, N. A., and de Nys, R. (2012). Total lipid and fatty acid composition of seaweeds for the selection of species for oil-based biofuel and bioproducts. Glob. Change Biol. Bioenerg. 4, 919–930. doi:10.1111/j.1757-1707.2012.01175.x
Greggio, N., Carlini, C., Contin, A., Soldano, M., and Marazza, D. (2018). Exploitable fish waste and stranded beach debris in the Emilia-Romagna Region (Italy). Waste Manag. 78, 566–575. doi:10.1016/j.wasman.2018.06.034
Gruduls, A., Balina, K., Ivanovs, K., and Romagnoli, F. (2018). Low temperature BMP tests using fish waste from invasive Round goby of the Baltic Sea. Agron. Res. 16, 2. doi:10.15159/ar.18.07
Gudiña, E. J., Teixeira, J. A., and Rodrigues, L. R. (2011). Biosurfactant-Producing lactobacilli: Screening, production profiles, and effect of medium composition. Appl. Environ. Soil Sci. 2011, 1–9. doi:10.1155/2011/201254
Guidara, M., Yaich, H., Richel, A., Blecker, C., Boufi, S., Attia, H., et al. (2019). Effects of extraction procedures and plasticizer concentration on the optical, thermal, structural and antioxidant properties of novel ulvan films. Int. J. Biol. Macromol. 135, 647–658. doi:10.1016/j.ijbiomac.2019.05.196
Hadiyarto, A., Budiyono, B., Djohari, S., Hutama, I., and Hasyim, W. (2015). The effect of F/M ratio to the anaerobic decomposition of biogas production from fish offal waste. Waste Technol. 3, 2. doi:10.12777/wastech.3.2.58-61
Hahn, T., Lang, S., Ulber, R., and Muffler, K. (2012). Novel procedures for the extraction of fucoidan from Brown algae. Process Biochem. 47, 1691–1698. doi:10.1016/j.procbio.2012.06.016
Hamza, M., Ayoub, M., Bin Shamsuddin, R., Mukhtar, A., Saqib, S., Zahid, I., et al. (2021). A review on the waste biomass derived catalysts for biodiesel production. Environ. Technol. Innov. 21, 101200. doi:10.1016/j.eti.2020.101200
Han, L., Wang, Q., Luo, Z., Rong, N., and Deng, G. (2013). H2 rich gas production via pressurized fluidized bed gasification of sawdust with in situ CO2 capture. Appl. Energy. 109, 36–43. doi:10.1016/j.apenergy.2013.03.035
Han, S. K., and Shin, H. S. (2004). Performance of an innovative two-stage process converting food waste to hydrogen and methane. J. Air Waste Manage. Assoc. 54, 242–249. doi:10.1080/10473289.2004.10470895
Hanifa-Jannat, M. A., Park, S. H., Chairattanawat, C., Yulisa, A., and Hwang, S. (2022). Effect of different microbial seeds on batch anaerobic digestion of fish waste. Bioresour. Technol. 349, 126834. doi:10.1016/j.biortech.2022.126834
Henriques, A., Vázquez, J. A., Valcarcel, J., Mendes, R., Bandarra, N. M., and Pires, C. (2021). Characterization of protein hydrolysates from fish discards and by-products from the north-west Spain fishing fleet as potential sources of bioactive peptides. Mar. Drugs. 19, 338. doi:10.3390/md19060338
Herrero, M., and Ibáñez, E. (2015). Green processes and sustainability: An overview on the extraction of high added-value products from seaweeds and microalgae. J. Supercrit. Fluids. 96, 211–216. doi:10.1016/j.supflu.2014.09.006
Hong, Y., and Wang, Y. (2017). Elucidation of reaction mechanism for m-cresol hydrodeoxygenation over Fe based catalysts: A kinetic study. Catal. Commun. 100, 43–47. doi:10.1016/j.catcom.2017.06.028
Hu, J., Luo, J., Zhu, Z., Chen, B., Ye, X., Zhu, P., et al. (2021). Multi-Scale biosurfactant production by Bacillus subtilis using tuna fish waste as substrate. Catalysts 11, 456. doi:10.3390/catal11040456
Idowu, A. T., Igiehon, O. O., Idowu, S., Olatunde, O. O., and Benjakul, S. (2021). Bioactivity potentials and general applications of fish protein hydrolysates. Int. J. Pept. Res. Ther. 27, 109–118. doi:10.1007/s10989-020-10071-1
Iovane, P., Donatelli, A., and Molino, A. (2013). Influence of feeding ratio on steam gasification of palm shells in a rotary kiln pilot plant. Experimental and numerical investigations. Exp. Numer. investigations. Biomass Bioenergy 56, 423–431. doi:10.1016/j.biombioe.2013.05.025
Ishak, N., and Sarbon, N. A. (2018). A review of protein hydrolysates and bioactive peptides deriving from wastes generated by fish processing. Food Bioprocess Technol. 11, 2–16. doi:10.1007/s11947-017-1940-1
Jamil, F., Al-Muhatseb, A. H., Myint, M. T. Z., Al-Hinai, M., Al-Haj, L., Baawain, M., et al. (2018). Biodiesel production by valorizing waste Phoenix dactylifera L. Kernel oil in the presence of synthesized heterogeneous metallic oxide catalyst (Mn@MgO-ZrO2). Energy Convers. Manag. 155, 128–137. doi:10.1016/j.enconman.2017.10.064
Jayasinghe, P., Adeoti, I., and Hawboldt, K. (2013). A study of process optimization of extraction of oil from fish waste for use as A low-grade fuel. JAOCS, J. Am. Oil Chem. Soc. 90, 1903–1915. doi:10.1007/s11746-013-2321-1
Jena, U., and Hoekman, S. K. (2017). Editorial: Recent advancements in algae-to-biofuels research: Novel growth technologies, conversion methods, and assessments of economic and environmental impacts. Front. Energy Res. 25, 11. doi:10.3389/fenrg.2017.00002
Jiménez-Llanos, J., Ramírez-Carmona, M., Rendón-Castrillón, L., and Ocampo-López, C. (2020). Sustainable biohydrogen production by chlorella sp. microalgae: A review. Int. J. Hydrogen Energy. 45, 8310–8328. doi:10.1016/j.ijhydene.2020.01.059
Jiménez-Peñalver, P., Koh, A., Gross, R., Gea, T., and Font, X. (2020). Biosurfactants from waste: Structures and interfacial properties of sophorolipids produced from a residual oil cake. J. Surfactants Deterg. 23, 481–486. doi:10.1002/jsde.12366
Just Economy (2022). Dead Loss: The high cost of poor farming practices and mortalities on salmon farms. Available at: https://www.justeconomics.co.uk/uploads/reports/Aquaculture-Report-v5.pdf (Accessed November 15, 2022).
Kadam, D., and Savant, D. (2019). Biosurfactant production from shrimp shell waste by Pseudomonas stutzeri. Indian J. Geo-Marine Sci. 48, 1411–1418.
Kadam, S. U., Tiwari, B. K., and O’Donnell, C. P. (2013). Application of novel extraction technologies for bioactives from marine algae. J. Agric. Food Chem. 61, 4667–4675. doi:10.1021/jf400819p
Kafle, G. K., Kim, S. H., and Sung, K. I. (2013). Ensiling of fish industry waste for biogas production: A lab scale evaluation of biochemical methane potential (bmp) and kinetics. Bioresour. Technol. 127, 326–336. doi:10.1016/j.biortech.2012.09.032
Kannah, R., Kavitha, S., PreethiParthiba-Karthikeyan, O., Kumar, G., Dai-Viet, N. V., et al. (2021). Techno-economic assessment of various hydrogen production methods – a review. Bioresour. Technol. 319, 124175. doi:10.1016/j.biortech.2020.124175
Kapdan, I. K., and Kargi, F. (2006). Bio-hydrogen production from waste materials. Enzyme Microb. Technol. 38, 569–582. doi:10.1016/j.enzmictec.2005.09.015
Kargi, F., Eren, N. S., and Ozmihci, S. (2012). Bio-hydrogen production from cheese whey powder (CWP) solution: Comparison of thermophilic and mesophilic dark fermentations. Int. J. Hydrogen Energy. 37, 8338–8342. doi:10.1016/j.ijhydene.2012.02.162
Kaskatepe, B., Yildiz, S., Gumustas, M., and Ozkan, S. A. (2015). Biosurfactant production by <italic>Pseudomonas aeruginosa</italic>in kefir and fish meal. Braz. J. Microbiol. 46, 855–859. doi:10.1590/S1517-838246320140727
Kaspars, I., Kriss, S., and Dagnija, B. (2018). Approach for modelling anaerobic digestion processes of fish waste. Energy Procedia 147, 390–396. doi:10.1016/j.egypro.2018.07.108
Kébé, N., Rieker, C., Fall, P., Diouf, D., Ndiaye, D., Mockenhaupt, T., et al. (2021). Anaerobic Co-digestion of fish processing waste with cow manure and waste of market (rests of fruits and vegetables): A lab scale batch test. J. Sustain. Bioenergy Syst. 11, 45–59. doi:10.4236/jsbs.2021.111004
Khan, A. K., Saba, A. U., Nawazish, S., Akhtar, F., Rashid, R., Mir, S., et al. (2017). Carrageenan based bionanocomposites as drug delivery tool with special emphasis on the influence of ferromagnetic nanoparticles. Oxid. Med. Cell. Longev. 2017, 1–13. doi:10.1155/2017/8158315
Khawli, F. Al, Pateiro, M., Domínguez, R., Lorenzo, J. M., Gullón, P., Kousoulaki, K., et al. (2019). Innovative green technologies of intensification for valorization of seafood and their by-products. Mar. Drugs 17, 689. doi:10.3390/md17120689
Kidgell, J. T., Magnusson, M., de Nys, R., and Glasson, C. R. K. (2019). Ulvan: A systematic review of extraction, composition and function. Algal Res. 39, 101422. doi:10.1016/j.algal.2019.101422
Kim, J. Y., Lee, S. M., and Lee, J. H. (2012). Biogas production from moon jellyfish (Aurelia aurita) using of the anaerobic digestion. J. Ind. Eng. Chem. 18, 2147–2150. doi:10.1016/j.jiec.2012.06.010
Kim, S. K., and Mendis, E. (2006). Bioactive compounds from marine processing byproducts - a review. Food Res. Int. 39, 383–393. doi:10.1016/j.foodres.2005.10.010
Knothe, G., and Razon, L. F. (2017). Biodiesel fuels. Prog. Energy Combust. Sci. 58, 36–59. doi:10.1016/j.pecs.2016.08.001
Konasani, V. R., Jin, C., Karlsson, N. G., and Albers, E. (2018). Ulvan lyase from Formosa agariphila and its applicability in depolymerisation of ulvan extracted from three different Ulva species. Algal Res. 36, 106–114. doi:10.1016/j.algal.2018.10.016
Korkmaz, K., and Tokur, B. (2022). Optimization of hydrolysis conditions for the production of protein hydrolysates from fish wastes using response surface methodology. Food Biosci. 45, 101312. doi:10.1016/j.fbio.2021.101312
Kotsopoulos, T. A., Fotidis, I. A., Tsolakis, N., and Martzopoulos, G. G. (2009). Biohydrogen production from pig slurry in a CSTR reactor system with mixed cultures under hyper-thermophilic temperature (70 °C). Biomass Bioenergy 33, 1168–1174. doi:10.1016/j.biombioe.2009.05.001
Kronberg, B.,K. H., and Lindman, B. (2014). “Types of surfactants, their synthesis, and applications,” in Surface chemistry of surfactants and polymers. Editors B. Kronberg, K. Holmberg, and B. Lindman (Chichester, United Kingdom: John Wiley and son), 1–47.
Kumar, A., Yau, Y., Ogita, S., and Scheibe, R. (2020b). Climate change, photosynthesis and advanced biofuels. Singapore: Springer.
Kumar, M., Sun, Y., Rathour, R., Pandey, A., Thakur, I. S., and Tsang, D. C. W. (2020a). Algae as potential feedstock for the production of biofuels and value-added products: Opportunities and challenges. Sci. Total Environ. 716, 137116. doi:10.1016/j.scitotenv.2020.137116
Kumari, S., Rath, P., Sri Hari Kumar, A., and Tiwari, T. N. (2015). Extraction and characterization of chitin and chitosan from fishery waste by chemical method. Environ. Technol. Innov. 3, 77–85. doi:10.1016/j.eti.2015.01.002
Lee, A. F., Bennett, J. A., Manayil, J. C., and Wilson, K. (2014). Heterogeneous catalysis for sustainable biodiesel production via esterification and transesterification. Chem. Soc. Rev. 43, 7887–7916. doi:10.1039/c4cs00189c
Lee, T. C., Mohd Pu’ad, N. A. S., Alipal, J., Muhamad, M. S., Basri, H., Idris, M. I., et al. (2022). Tilapia wastes to valuable materials: A brief review of biomedical, wastewater treatment, and biofuel applications. Mat. Today Proc. 57, 1389–1395. doi:10.1016/j.matpr.2022.03.174
Liao, Y. C., Chang, C. C., Nagarajan, D., Chen, C. Y., and Chang, J. S. (2021). Algae-derived hydrocolloids in foods: Applications and health-related issues. Bioengineered 12, 3787–3801. doi:10.1080/21655979.2021.1946359
Lim, S. J., Wan Aida, W. M., Maskat, M. Y., Mamot, S., Ropien, J., and Mazita Mohd, D. (2014). Isolation and antioxidant capacity of fucoidan from selected Malaysian seaweeds. Food Hydrocoll. 42, 280–288. doi:10.1016/j.foodhyd.2014.03.007
Lionetto, F., and Corcione, C. E. (2021). Recent applications of biopolymers derived from fish industry waste in food packaging. Polym. (Basel) 13, 2337. doi:10.3390/polym13142337
Longwen, O., Shuyun, Li., Tao, L., Phillips, S., Hawkins, T., Singh, A., et al. (2022). Techno-economic analysis and life-cycle analysis of renewable diesel fuels produced with waste feedstocks. ACS Sustain. Chem. Eng. 10, 382–393. doi:10.1021/acssuschemeng.1c06561
López-Pedrouso, M., Lorenzo, J. M., Cantalapiedra, J., Zapata, C., Franco, J. M., and Franco, D. (2020). Aquaculture and by-products: Challenges and opportunities in the use of alternative protein sources and bioactive compounds. Adv. Food Nutr. Res. 92, 127–185. doi:10.1016/bs.afnr.2019.11.001
Lu, C., Li, W., Zhang, Q., Liu, L., Zhang, N., Qu, B., et al. (2021). Enhancing PhotoFermentation biohydrogen production by strengthening the beneficial metabolic products with catalysts. J. Clean. Prod. 317, 128437. doi:10.1016/j.jclepro.2021.128437
Lukitawesa-Patinvoh, R. J., Millati, R., Sárvári-Horváth, I., and Taherzadeh, M. J. (2020). Factors influencing volatile fatty acids production from food wastes via anaerobic digestion. Bioengineered 11, 39–52. doi:10.1080/21655979.2019.1703544
Luthuli, S., Wu, S., Cheng, Y., Zheng, X., Wu, M., and Tong, H. (2019). Therapeutic effects of fucoidan: A review on recent studies. Mar. Drugs. 17, 487. doi:10.3390/md17090487
Madeen, S., Alles, K., and Demirel, Y. (2021). Measuring sustainability of renewable diesel production using a multi-criteria decision matrix. Biofuels, Bioprod. Biorefinering 15, 1621–1637. doi:10.1002/bbb.2272
Mahdavi, M., Abedini, E., and Darabi, A. (2015). Biodiesel synthesis from oleic acid by nano-catalyst (ZrO2/Al2O3) under high voltage conditions. RSC Adv. 5, 55027–55032. doi:10.1039/C5RA07081C
Makkar, R. S., Cameotra, S. S., and Banat, I. M. (2011). Advances in utilization of renewable substrates for biosurfactant production. Amb. Express 1, 5. doi:10.1186/2191-0855-1-5
Malara, D., Mielke, C., Oelgemöller, M., Senge, M. O., and Heimann, K. (2017). Sustainable water treatment in aquaculture – photolysis and photodynamic therapy for the inactivation of Vibrio species. Aquac. Res. 48, 2954–2962. doi:10.1111/are.13128
Mangano, M. C., Giacoletti, A., and Sarà, G. (2019). Dynamic energy budget provides mechanistic derived quantities to implement the ecosystem based management approach. J. Sea Res. 143, 272–279. doi:10.1016/j.seares.2018.05.009
Manivasagan, P., and Kim, S. K. (2014). “Chapter five - extracellular polysaccharides produced by marine bacteria,” in Marine carbohydrates: Fundamentals and applications, Part A. Editor N. R. Kim (Academic Press), 79.
Mansir, N., Teo, S. H., Rashid, U., Saiman, M. I., Tan, Y. P., Alsultan, G. A., et al. (2018). Modified waste egg shell derived bifunctional catalyst for biodiesel production from high FFA waste cooking oil. A review. Renew. Sustain. Energy Rev. 82, 3645–3655. doi:10.1016/j.rser.2017.10.098
Mao, C., Feng, Y., Wang, X., and Ren, G. (2015). Review on research achievements of biogas from anaerobic digestion. Renew. Sustain. Energy Rev. 45, 540–555. doi:10.1016/j.rser.2015.02.032
Marinković, D. M., Stanković, M. V., Veličković, A. V., Avramović, J. M., Miladinović, M. R., Stamenković, O. O., et al. (2016). Calcium oxide as a promising heterogeneous catalyst for biodiesel production: Current state and perspectives. Renew. Sustain. Energy Rev. 56, 1387–1408. doi:10.1016/j.rser.2015.12.007
Martins, M., Vieira, F. A., Correia, I., Ferreira, R. A. S., Abreu, H., Coutinho, J. A. P., et al. (2016). Recovery of phycobiliproteins from the red macroalga Gracilaria sp. using ionic liquid aqueous solutions. Green Chem. 15, 4287–4296. doi:10.1039/C6GC01059H
Martins, P. C., and Martins, V. G. (2018). Biosurfactant production from industrial wastes with potential remove of insoluble paint. Int. Biodeterior. Biodegrad. 127, 10–16. doi:10.1016/j.ibiod.2017.11.005
Marzieh, M. N., Zahra, F., Tahereh, E., and Sara, K. N. (2019). Comparison of the physicochemical and structural characteristics of enzymatic produced chitin and commercial chitin. Int. J. Biol. Macromol. 139, 270–276. doi:10.1016/j.ijbiomac.2019.07.217
Mata-Alvarez, J., Macé, S., and Llabrés, P. (2000). Anaerobic digestion of organic solid wastes. An overview of research achievements and perspectives. Bioresour. Technol. 74, 3–16. doi:10.1016/S0960-8524(00)00023-7
Melitos, G., Voulkopoulos, X., and Zabaniotou, A. (2021). Waste to sustainable biohydrogen production via photo-fermentation and biophotolysis − A systematic review. Renew. Energy Environ. Sustain. 6, 45. doi:10.1051/rees/2021047
Milano, J., Ong, H. C., Masjuki, H. H., Chong, W. T., Lam, M. K., Loh, P. K., et al. (2016). Microalgae biofuels as an alternative to fossil fuel for power generation. Renew. Sustain. Energy Rev. 58, 180–197. doi:10.1016/j.rser.2015.12.150
Milledge, J. J., Nielsen, B. V., Maneein, S., and Harvey, P. J. (2019). A brief review of anaerobic digestion of algae for bioenergy. Energies 12, 1166. doi:10.3390/en12061166
Miller, P., and Kumar, A. (2014). Techno-economic assessment of hydrogenation-derived renewable diesel production from canola and camelina. Sustain Energy Technol. Assess. 6, 105–115. doi:10.1016/j.seta.2014.01.008
Milono, P., Lindajati, T., and Aman, S. (1981). Biogas production from agricultural organic residues. Internat. J. Sci. Eng. 1 (2), 33
Mohammed, A., Rivers, A., Stuckey, D. C., and Ward, K. (2020). Alginate extraction from Sargassum seaweed in the Caribbean region: Optimization using response surface methodology. Carbohydr. Polym. 245, 116419. doi:10.1016/j.carbpol.2020.116419
Mohanty, S. S., Koul, Y., Varjani, S., Pandey, A., Ngo, H. H., Chang, J. S., et al. (2021). A critical review on various feedstocks as sustainable substrates for biosurfactants production: A way towards cleaner production. Microb. Cell. Fact. 20, 120. doi:10.1186/s12934-021-01613-3
Mona, S., Kumar, S. S., Kumar, V., Parveen, K., Saini, N., Deepak, B., et al. (2020). Green technology for sustainable biohydrogen production (waste to energy): A review. Sci. Total Environ. 728, 138481. doi:10.1016/j.scitotenv.2020.138481
Morimura, S., Nagata, H., Uemura, Y., Fahmi, A., Shigematsu, T., and Kida, K. (2002). Development of an effective process for utilization of collagen from livestock and fish waste. Process Biochem. 37, 1403–1412. doi:10.1016/S0032-9592(02)00024-9
Mukherjee, S., Das, P., and Sen, R. (2006). Towards commercial production of microbial surfactants. Trends Biotechnol. 24, 509–515. doi:10.1016/j.tibtech.2006.09.005
Nalinga, Y., and Legonda, I. (2016). Experimental investigation on biogas production from anaerobic co-digestion of water hyacinth and fish waste. Int. J. Innov. Res. Technol. Sci. 4, 1–8.
Narudin, N. A. H., Rosman, N. A., Shahrin, E. W. E., Sofyan, N., Hanif Mahadi, A., Kusrini, E., et al. (2022). Extraction, characterization, and kinetics of N-deacetylation of chitin obtained from mud crab shells. Polym. Polym. Compos. 30, 096739112211096. doi:10.1177/09673911221109611
Nazurally, N. (2018). Anaerobic digestion of fish waste and seagrass/macroalgae: Potential sustainable waste management for tropical small island developing states. J. Mater Cycles Waste Manag. 20, 1724–1735. doi:10.1007/s10163-018-0738-1
Ng, Y. J., Lim, H. R., Khoo, K. S., Chew, K. W., Chan, D. J. C., Bilal, M., et al. (2022). Recent advances of biosurfactant for waste and pollution bioremediation: Substitutions of petroleum-based surfactants. Environ. Res. 212, 113126. doi:10.1016/j.envres.2022.113126
Nges, I. A., Mbatia, B., and Björnsson, L. (2012). Improved utilization of fish waste by anaerobic digestion following omega-3 fatty acids extraction. J. Environ. Manage. 110, 159–165. doi:10.1016/j.jenvman.2012.06.011
Nicita, A., Maggio, G., Andaloro, A. P. F., and Squadrito, G. (2020). Green hydrogen as feedstock: Financial analysis of a photovoltaic-powered electrolysis plant. Int. J. Hydrogen Energy. 45. doi:10.1016/j.ijhydene.2020.02.062
Oliveira, L. E., Barboza, J. C. S., and Da Silva, M. L. C. P. (2013). Production of ethylic biodiesel from Tilápia visceral oil. Int. Conf. Renew. Energy Power Qual. 11, 683–686. doi:10.24084/repqj11.412
Oliveira, V. H. S., Dean, K. R., Qviller, L., Kirkeby, C., and Bang Jensen, B. (2021). Factors associated with baseline mortality in Norwegian Atlantic salmon farming. Sci. Rep. 11, 14702. doi:10.1038/s41598-021-93874-6
Pacwa-Płociniczak, M., Płaza, G. A., Piotrowska-Seget, Z., and Cameotra, S. S. (2011). Environmental applications of biosurfactants: Recent advances. Int. J. Mol. Sci. 12, 633–654. doi:10.3390/ijms12010633
Paone, E., Fazzino, F., Pizzone, D. M., Scurria, A., Pagliaro, M., Ciriminna, R., et al. (2021). Towards the anchovy biorefinery: Biogas production from anchovy processing waste after fish oil extraction with biobased limonene. Sustainability 13, 2428. doi:10.3390/su13052428
Papargyriou, D., Broumidis, E., de Vere-Tucker, M., Gavrielides, S., Hilditch, P., Irvine, J. T. S., et al. (2019). Investigation of solid base catalysts for biodiesel production from fish oil. Renew. Energy 139, 661–669. doi:10.1016/j.renene.2019.02.124
Patel, R. M., and Desai, A. J. (1997). Biosurfactant production by Pseudomonas aeruginosa GS3 from molasses. Lett. Appl. Microbiol. 25, 91–94. doi:10.1046/j.1472-765X.1997.00172.x
Pellis, A., Guebitz, G. M., and Nyanhongo, G. S. (2022). Chitosan: Sources, processing and modification techniques. Gels 8, 393. doi:10.3390/gels8070393
Perera, K. R. J., Ketheesan, B., Gadhamshetty, V., and Nirmalakhandan, N. (2010). Fermentative biohydrogen production: Evaluation of net energy gain. Int. J. Hydrogen Energy 35, 12224–12233. doi:10.1016/j.ijhydene.2010.08.037
Pérez, W. A., Marín, J. A., López, J. N., Burgos, M. A., and Rios, L. A. (2022). Development of a pilot-ecofriendly process for chitosan production from waste shrimp shells. Environ. Process. 9, 55. doi:10.1007/s40710-022-00605-8
Priya, P. S., Verma, Y., Muhal, R. A., Goswami, C., and Singh, T. (2021). Biofuels: An alternative to conventional fuel and energy source. Mat. Today Proc. 48, 1178–1184. doi:10.1016/j.matpr.2021.08.227
Ptak, S. H., Christensen, K. V., Meichßner, R., and Fretté, X. (2019). Improving fucoidan yield from Fucus Brown algae by microwave extraction. Chem. Eng. Trans. 74. doi:10.3303/CET1974019
Qu, L., Jiang, X., Zhang, Z., Zhang, X., Song, G., Wang, H., et al. (2021). A review of hydrodeoxygenation of bio-oil: Model compounds, catalysts, and equipment. Green Chem. 23, 9348–9376. doi:10.1039/D1GC03183J
Qureshi, D., Nayak, S. K., Maji, S., Kim, D., Banerjee, I., and Pal, K. (2019). Carrageenan: A wonder polymer from marine algae for potential drug delivery applications. Curr. Pharm. Des. 25, 1172–1186. doi:10.2174/1381612825666190425190754
Rahman, P. K. S. M., Mayat, A., Harvey, J. G. H., Randhawa, K. S., Relph, L. E., and Armstrong, M. C. (2019). “Biosurfactants and bioemulsifiers from marine algae BT.” in The role of microalgae in wastewater treatment Editor. L. B. Sukla, E. Subudhi, and D. Pradhan (Singapore: Springer Singapore), 169–188.
Rahman, S. N. A., Masdar, M. S., Rosli, M. I., Majlan, E. H., Husaini, T., Kamarudin, S. K., et al. (2016). Overview biohydrogen technologies and application in fuel cell technology. Renew. Sustain. Energy Rev. 66, 137–162. doi:10.1016/j.rser.2016.07.047
Rai, A. K., Swapna, H. C., Bhaskar, N., Halami, P. M., and Sachindra, N. M. (2010). Effect of fermentation ensilaging on recovery of oil from fresh water fish viscera. Enzyme Microb. Technol. 46, 9–13. doi:10.1016/j.enzmictec.2009.09.007
Rajendran, N., Pandey, A., Gnansounou, E., Gurunathan, B., and Han, J. (2022). Chapter 25 - techno-economic analysis of biodiesel production from nonedible biooil using catalytic transesterification”Biofuels and Bioenergy. Editors B. Gurunathan, and R. Sahadevan (Elsevier), 601–626.
Ramos-Suárez, J. L., Arroyo, N. C., and González-Fernández, C. (2015). “The role of anaerobic digestion in algal biorefineries: Clean energy production, organic waste treatment, and nutrient loop closure,” in Algae and environmental sustainability. Editors B. Singh, K. Bauddh, and F. Bux (New Delhi: Springer India), 53.
Ranjbari, M., Shams Esfandabadi, Z., Ferraris, A., Quatraro, F., Rehan, M., Nizami, A. S., et al. (2022). Biofuel supply chain management in the circular economy transition: An inclusive knowledge map of the field. Chemosphere 296, 133968. doi:10.1016/j.chemosphere.2022.133968
Raposo, M. F., De Morais, R. M., and Bernardo de Morais, A. M. (2013). Bioactivity and applications of sulphated polysaccharides from marine microalgae. Mar. Drugs. 11, 233–252. doi:10.3390/md11010233
Ren, J., and Toniolo, S. (2018). Life cycle sustainability decision-support framework for ranking of hydrogen production pathways under uncertainties: An interval multi-criteria decision making approach. J. Clean. Prod. 175, 222–236. doi:10.1016/j.jclepro.2017.12.070
Renewables (2021). Global status report. Available at: https://www.ren21.net/five-takeaways-from-ren21s-renewables-2021-global-status-report/(Accessed September 20, 2022).
Reza, M. S., Azad, A. K., Bakar, M. S. A., Karim, M. R., Sharifpur, M., and Taweekun, J. (2022). Evaluation of thermochemical characteristics and pyrolysis of fish processing waste for renewable energy feedstock. Sustain 14, 1203. doi:10.3390/su14031203
Ribeiro, B. G., dos Santos, M. M., Pinto, M. I. S., Meira, H. M., Durval, I. J. B., and Guerra, J. M. C. (2019). Production and optimization of the extraction conditions of the biosurfactant from Candida utilis UFPEDA1009 with potential application in the food industry. Chem. Eng. Trans. 74, 4247. doi:10.3303/CET1974247
Rocher, D. F., Cripwell, R. A., and Viljoen-Bloom, M. (2021). Engineered yeast for enzymatic hydrolysis of laminarin from Brown macroalgae. Algal Res. 54, 102233. doi:10.1016/j.algal.2021.102233
Rowland, S., Bower, C. K., Patil, K. N., and DeWitt, C. A. M. (2009). Updraft gasification of salmon processing waste. J. Food Sci. 74, E426–E431. doi:10.1111/j.1750-3841.2009.01312.x
Rudke, A. R., de Andrade, C. J., and Ferreira, S. R. S. (2020). Kappaphycus alvarezii macroalgae: An unexplored and valuable biomass for green biorefinery conversion. Trends Food Sci. Technol. 103, 214–224. doi:10.1016/j.tifs.2020.07.018
Rudovica, V., Rotter, A., Gaudêncio, S. P., Novoveská, L., Akgül, F., Akslen-Hoel, L. K., et al. (2021). Valorization of marine waste: Use of industrial by-products and beach wrack towards the production of high added-value products. Front. Mar. Sci. 8, 333. doi:10.3389/fmars.2021.723333
Sáenz-Marta, C. I., Ballinas-Casarrubias, M. de L., Rivera-Chavira, B. E., and Nevárez-Moorillón, G. V. (2015). “Biosurfactants as useful tools in bioremediation,” in Advances in bioremediation of wastewater and polluted soil. Editor N. Shiomi (London: IntechOpen).
Sakr, E. A. E., Ahmed, H. A. E., and Abo Saif, F. A. A. (2021). Characterization of low-cost glycolipoprotein biosurfactant produced by Lactobacillus plantarum 60 FHE isolated from cheese samples using food wastes through response surface methodology and its potential as antimicrobial, antiviral, and anticancer activities. Int. J. Biol. Macromol. 170, 94–106. doi:10.1016/j.ijbiomac.2020.12.140
Sarà, G., Mangano, M. C., Johnson, M., and Mazzola, A. (2018). Integrating multiple stressors in aquaculture to build the blue growth in a changing sea. Hydrobiologia 809, 5–17. doi:10.1007/s10750-017-3469-8
Saranya, P., Swarnalatha, S., and Sekaran, G. (2014). Lipoprotein biosurfactant production from an extreme acidophile using fish oil and its immobilization in nanoporous activated carbon for the removal of Ca2+ and Cr3+ in aqueous solution. RSC Adv. 4, 34144–34155. doi:10.1039/C4RA03101F
Savage, P. E. (2012). Algae under pressure and in hot water. Science 80, 1039–1040. doi:10.1126/science.1224310
Schenk, P. M., Thomas-Hall, S. R., Stephens, E., Marx, U. C., Mussgnug, J. H., Posten, C., et al. (2008). Second generation biofuels: High-efficiency microalgae for biodiesel production. BioEnergy Res. 1, 20–43. doi:10.1007/s12155-008-9008-8
Serrano, A., Siles, J. A., Gutiérrez, M. C., and Martín, M. Á. (2014). Optimization of anaerobic co-digestion of strawberry and fish waste. Appl. Biochem. Biotechnol. 173, 1391–1404. doi:10.1007/s12010-014-0942-y
Shahabuddin, M., Krishna, B. B., Bhaskar, T., and Perkins, G. (2020). Advances in the thermo-chemical production of hydrogen from biomass and residual wastes: Summary of recent techno-economic analyses. Bioresour. Technol. 299, 122557. doi:10.1016/j.biortech.2019.122557
Sharmila, G., Kumar, D., Pugazhendi, A., Bajhaiya, A. K., Gugulothu, P., and Banu, R. (2021). Biofuel production from macroalgae: Present scenario and future scope. Bioengineered 12, 9216–9238. doi:10.1080/21655979.2021.1996019
Shi, X., Lin, J., Zuo, J., Li, P., Li, X., and Guo, X. (2017). Effects of free ammonia on volatile fatty acid accumulation and process performance in the anaerobic digestion of two typical bio-wastes. J. Environ. Sci. (China) 55, 49–57. doi:10.1016/j.jes.2016.07.006
Shin, H., Youn, J., and Kim, S. (2004). Hydrogen production from food waste in anaerobic mesophilic and thermophilic acidogenesis. Int. J. Hydrogen Energy. 29, 1355–1363. doi:10.1016/j.ijhydene.2003.09.011
Siddik, M. A. B., Howieson, J., Fotedar, R., and Partridge, G. J. (2021). Enzymatic fish protein hydrolysates in finfish aquaculture: A review. Rev. Aquac. 13, 406–430. doi:10.1111/raq.12481
Silva, T. H., Alves, A., Popa, E. G., Reys, L. L., Gomes, M. E., Sousa, R. A., et al. (2012). Marine algae sulfated polysaccharides for tissue engineering and drug delivery approaches. Biomatter 2, 278–289. doi:10.4161/biom.22947
Singh, D., Sharma, D., Soni, S. L., Sharma, S., and Kumari, D. (2019). Chemical compositions, properties, and standards for different generation biodiesels: A review. Fuel 253, 60–71. doi:10.1016/j.fuel.2019.04.174
Skaggs, R. L., Coleman, A. M., Seiple, T. E., and Milbrandt, A. R. (2018). Waste-to-Energy biofuel production potential for selected feedstocks in the conterminous United States. Renew. Sustain. Energy Rev. 82, 2640–2651. doi:10.1016/j.rser.2017.09.107
Solli, L., Bergersen, O., Sørheim, R., and Briseid, T. (2014). Effects of a gradually increased load of fish waste silage in co-digestion with cow manure on methane production. Waste Manag. 34, 1553–1559. doi:10.1016/j.wasman.2014.04.011
Song, W., Ding, L., Liu, M., Cheng, J., Zhou, J., and Li, Y. Y. (2020). Improving biohydrogen production through dark fermentation of steam-heated acid pretreated Alternanthera philoxeroides by mutant Enterobacter aerogenes ZJU1. ZJU1. Sci. Total Environ. 716, 134695. doi:10.1016/j.scitotenv.2019.134695
Sousa, A. M. M., Alves, V. D., Morais, S., Delerue-Matos, C., and Gonçalves, M. P. (2010). Agar extraction from integrated multitrophic aquacultured Gracilaria vermiculophylla: Evaluation of a microwave-assisted process using response surface methodology. Bioresour. Technol. 101, 3258–3267. doi:10.1016/j.biortech.2009.12.061
Srikanya, A., Dhanapal, K., Sravani, K., Madhavi, K., and Kumar, G. P. (2017). A study on optimization of fish protein hydrolysate preparation by enzymatic hydrolysis from Tilapia fish waste mince. Int. J. Curr. Microbiol. Appl. Sci. 6, 3220–3229. doi:10.20546/ijcmas.2017.612.375
Srinivasan, H., Kanayairam, V., and Ravichandran, R. (2018). Chitin and chitosan preparation from shrimp shells Penaeus monodon and its human ovarian cancer cell line, PA-1. Int. J. Biol. Macromol. 107, 662–667. doi:10.1016/j.ijbiomac.2017.09.035
Sterner, M., and Gröndahl, F. (2021). Extraction of laminarin from Saccharina latissima seaweed using cross-flow filtration. J. Appl. Phycol. 33, 1825–1844. doi:10.1007/s10811-021-02398-z
Stronach, S. M., Rudd, T., and Lester, J. N. (2012). “The biochemestry of anaerobic digestion,” in Anaerobic digestion processes in industrial wastewater treatment. Editors S. Aiba, L. T. Fan, A. Fiechter, J. de Klein, and K. Schügerl (Berlin, Germany: Biotechnology monographsSpringer Science and Business Media), 1.
Subhan, F., Hussain, Z., Tauseef, I., Shehzad, A., and Wahid, F. (2021). A review on recent advances and applications of fish collagen. Crit. Rev. Food Sci. Nutr. 61, 1027–1037. doi:10.1080/10408398.2020.1751585
Sumathi, S., Chai, S., and Mohamed, A. (2008). Utilization of oil palm as a source of renewable energy in Malaysia. Renew. Sustain. Energy Rev. 12, 2404–2421. doi:10.1016/j.rser.2007.06.006
Suresh Kumar, A., Mody, K., and Jha, B. (2007). Bacterial exopolysaccharides – A perception. J. Basic Microbiol. 47, 103–117. doi:10.1002/jobm.200610203
Suseno, S. H., Nurjanah, N., Yoshiara, Y., and Saraswati, S. (2015). Determination of extraction temperature and period of fish oil from tilapia (oreochromis niloticus) by product using wet rendering method Sugeng. 1st Int. Symp. Aquat. Prod. Process. 1. doi:10.18502/kls.v1i0.96
Tan, Y., and Chang, S. K. C. (2018). Isolation and characterization of collagen extracted from channel catfish (Ictalurus punctatus) skin. Food Chem. 242, 147–155. doi:10.1016/j.foodchem.2017.09.013
Tanaka, T., Takahashi, K., Tsubaki, K., Hirata, M., Yamamoto, K., Biswas, A., et al. (2018). Isolation and characterization of acid-soluble bluefin tuna (Thunnus orientalis) skin collagen. Fish. Aquat. Sci. 21, 7. doi:10.1186/s41240-018-0084-1
Tang, S., Ma, Y., Dong, X., Zhou, H., He, Y., Ren, D., et al. (2022). Enzyme-assisted extraction of fucoidan from Kjellmaniella crassifolia based on kinetic study of enzymatic hydrolysis of algal cellulose. Algal Res. 66, 102795. doi:10.1016/j.algal.2022.102795
Tanwar, D., Ajayta, Dilip, S., Mathur, Y. P., Khatri, K. K., and Soni, S. S. (2013). Production and characterization of fish oil methyl ester. Int. J. Innov. Technol. Res., 209–217.
The Climate Institute (2017). Algae, cyanobacteria blooms, and climate change. Available at: http://climate.org/algae-cyanobacteria-blooms-and-climate-change/(Accessed November 15, 2022).
Toldrá-Reig, F., Mora, L., and Toldrá, F. (2020). Trends in biodiesel production from animal fat waste. Appl. Sci. 10, 3644. doi:10.3390/app10103644
Tsukahara, K., Kimura, T., Minowa, T., Sawayama, S., Yagishita, T., Inoue, S., et al. (2001). Microalgal cultivation in a solution recovered from the low-temperature catalytic gasification of the microalga. J. Biosci. Bioeng. 91, 311–313. doi:10.1016/S1389-1723(01)80140-7
Tuck, C. O., Pérez, E., Horváth, I. T., Sheldon, R. A., and Poliakoff, M. (2012). Valorization of biomass: Deriving more value from waste. Science 8037, 695–699. doi:10.1126/science.1218930
Usman, A., Khalid, S., Usman, A., Hussain, Z., and Wang, Y. (2017). “Chapter 5 - algal polysaccharides, novel application, and outlook,” in Algae based polymers, blends and composites. Editors K. M. Zia, M. Zuber, and M. Ali (Elsevier), 115.
Valdez-Vazquez, I., Ríos-Leal, E., Esparza-García, F., Cecchi, F., and Poggi-Varaldo, H. M. (2005). Semi-continuous solid substrate anaerobic reactors for H2 production from organic waste: Mesophilic versus thermophilic regime. Int. J. Hydrogen Energy. 30. doi:10.1016/j.ijhydene.2004.09.016
Van, D. P., Fujiwara, T., Tho, L., Song Toan, P. P., and Hoang Minh, G. (2020). A review of anaerobic digestion systems for biodegradable waste: Configurations, operating parameters, and current trends. Environ. Eng. Res. 25, 1–17. doi:10.4491/eer.2018.334
Van Osch, S., Hynes, S., O’Higgins, T., Hanley, N., Campbell, D., and Freeman, S. (2017). Estimating the Irish public’s willingness to pay for more sustainable salmon produced by integrated multi-trophic aquaculture. Mar. Policy. 84, 220–227. doi:10.1016/j.marpol.2017.07.005
Varun, T. K., Senani, S., Jayapal, N., Chikkerur, J., Roy, S., Tekulapally, V. B., et al. (2017). Extraction of chitosan and its oligomers from shrimp shell waste, their characterization and antimicrobial effect. Vet. World. 10, 170–175. doi:10.14202/vetworld.2017.170-175
Venkata Subhash, G., and Venkata Mohan, S. (2014). Deoiled algal cake as feedstock for dark fermentative biohydrogen production: An integrated biorefinery approach. Int. J. Hydrogen Energy 39, 9573–9579. doi:10.1016/j.ijhydene.2014.04.003
Vijayakumar, S., and Saravanan, V. (2015). Biosurfactants-types, sources and applications. Res. J. Microbiol. 10, 181–192. doi:10.3923/jm.2015.181.192
Vishwakarma, R., Dhaka, V., Ariyadasa, T. U., and Malik, A. (2022). Exploring algal technologies for a circular bio-based economy in rural sector. J. Clean. Prod. 354, 131653. doi:10.1016/j.jclepro.2022.131653
Wang, J., Pei, X., Liu, H., and Zhou, D. (2018). Extraction and characterization of acid-soluble and pepsin-soluble collagen from skin of loach (Misgurnus anguillicaudatus). Int. J. Biol. Macromol. 106, 544–550. doi:10.1016/j.ijbiomac.2017.08.046
Wang, W., Li, L., Wu, K., Zhu, G., Tan, S., Li, W., et al. (2015). Hydrothermal synthesis of bimodal mesoporous MoS2 nanosheets and their hydrodeoxygenation properties. RSC Adv. 5, 61799–61807. doi:10.1039/c5ra09690a
Ward, A. J., Lewis, D. M., and Green, F. B. (2014). Anaerobic digestion of algae biomass: A review. Algal Res. 5, 204–214. doi:10.1016/j.algal.2014.02.001
Wijesinghe, W. A. J. P., and Jeon, Y. J. (2012). Biological activities and potential industrial applications of fucose rich sulfated polysaccharides and fucoidans isolated from Brown seaweeds: A review. Carbohydr. Polym. 88, 13–20. doi:10.1016/j.carbpol.2011.12.029
Wu, X., and Leung, D. Y. C. (2011). Optimization of biodiesel production from camelina oil using orthogonal experiment. Appl. Energy 88, 3615–3624. doi:10.1016/j.apenergy.2011.04.041
Xiao, R., and Zheng, Y. (2016). Overview of microalgal extracellular polymeric substances (EPS) and their applications. Biotechnol. Adv. 34, 1225–1244. doi:10.1016/j.biotechadv.2016.08.004
Xu, F., Li, Y., Ge, X., Yang, L., and Li, Y. (2018). Anaerobic digestion of food waste – challenges and opportunities. Bioresour. Technol. 247, 1047–1058. doi:10.1016/j.biortech.2017.09.020
Xu, S. Y., Huang, X., and Cheong, K. L. (2017). Recent advances in marine algae polysaccharides: Isolation, structure, and activities. Mar. Drugs. 15, 388. doi:10.3390/md15120388
Yang, F., Liu, D., Zhao, Y., Wang, H., Han, J., Ge, Q., et al. (2018). Size dependence of vapor phase hydrodeoxygenation of m-cresol on Ni/SiO2 catalysts. ACS Catal. 8, 1672–1682. doi:10.1021/acscatal.7b04097
Yuan, Y., and Macquarrie, D. (2015). Microwave assisted extraction of sulfated polysaccharides (fucoidan) from Ascophyllum nodosum and its antioxidant activity. Carbohydr. Polym. 129, 101–107. doi:10.1016/j.carbpol.2015.04.057
Yuvaraj, D., Bharathiraja, B., Rithika, J., Dhanasree, S., Ezhilarasi, V., Lavanya, A., et al. (2019). Production of biofuels from fish wastes: An overview. Biofuels 10, 301–307. doi:10.1080/17597269.2016.1231951
Zhang, B., Zhang, L., Yang, Z., Yan, Y., Pu, G., and Guo, M. (2015a). Hydrogen-rich gas production from wet biomass steam gasification with CaO/MgO. Int. J. Hydrogen Energy. 40, 8816–8823. doi:10.1016/j.ijhydene.2015.05.075
Zhang, H., and Row, K. H. (2015b). Extraction and separation of polysaccharides from laminaria japonica by size-exclusion chromatography. J. Chromatogr. Sci. 53, 498–502. doi:10.1093/chromsci/bmu073
Zhang, J., Sun, J., and Wang, Y. (2020). Recent advances in the selective catalytic hydrodeoxygenation of lignin-derived oxygenates to arenes. Green Chem. 22, 1072–1098. doi:10.1039/C9GC02762A
Zhang, Z., Wang, F., Wang, X., Liu, X., Hou, Y., and Zhang, Q. (2010). Extraction of the polysaccharides from five algae and their potential antioxidant activity in vitro. Carbohydr. Polym. 82, 118–121. doi:10.1016/j.carbpol.2010.04.031
Zhaohui, X., Yue, Y., Wancong, Y., Xin, G., Yixia, Z., and Xiaohong, K. (2020). Development prospect and preparation technology of edible oil from microalgae. Front. Mar. Sci. 7, 402. doi:10.3389/fmars.2020.00402
Zhu, Z., Zhang, B., Cai, Q., Ling, J., Lee, K., and Chen, B. (2020). Fish waste based lipopeptide production and the potential application as a bio-dispersant for oil spill control. Front. Bioeng. Biotechnol. 8, 734. doi:10.3389/fbioe.2020.00734
Keywords: biofuels, bioderivatives, aquaculture, marine waste, circular economy
Citation: Alvarado-Ramírez L, Santiesteban-Romero B, Poss G, Sosa-Hernández JE, Iqbal HMN, Parra-Saldívar R, Bonaccorso AD and Melchor-Martínez EM (2023) Sustainable production of biofuels and bioderivatives from aquaculture and marine waste. Front. Chem. Eng. 4:1072761. doi: 10.3389/fceng.2022.1072761
Received: 17 October 2022; Accepted: 13 December 2022;
Published: 04 January 2023.
Edited by:
Muhammad Sajid, Yibin University, ChinaReviewed by:
Nur Izyan Wan Azelee, University of Technology Malaysia, MalaysiaAyesha Shahid, University of Agriculture, Pakistan
Shahi Mulk, Shenzhen University, China
Copyright © 2023 Alvarado-Ramírez, Santiesteban-Romero, Poss, Sosa-Hernández, Iqbal, Parra-Saldívar, Bonaccorso and Melchor-Martínez. This is an open-access article distributed under the terms of the Creative Commons Attribution License (CC BY). The use, distribution or reproduction in other forums is permitted, provided the original author(s) and the copyright owner(s) are credited and that the original publication in this journal is cited, in accordance with accepted academic practice. No use, distribution or reproduction is permitted which does not comply with these terms.
*Correspondence: Roberto Parra-Saldívar, ci5wYXJyYUB0ZWMubXg=; Alfredo D. Bonaccorso, YWRiNTZAc3QtYW5kcmV3cy5hYy51aw==; Elda M. Melchor-Martínez, ZWxkYS5tZWxjaG9yQHRlYy5teA==, ZW0zNzhAc3QtYW5kcmV3cy5hYy51aw==