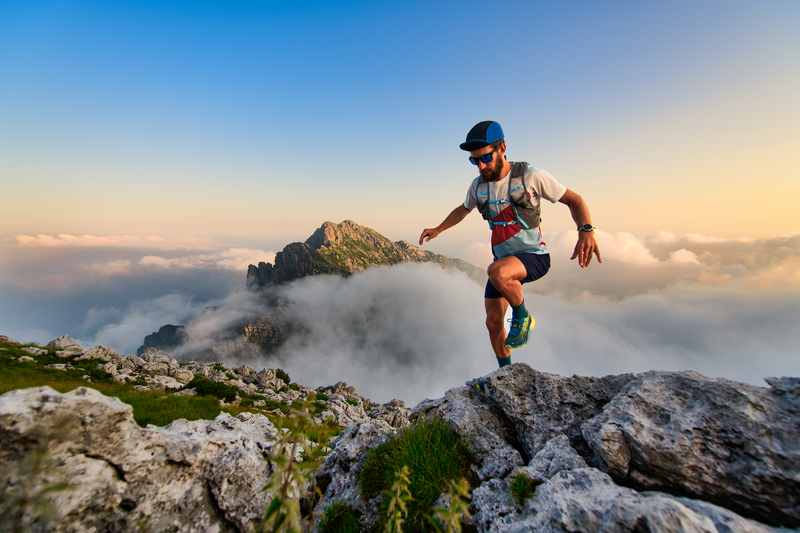
94% of researchers rate our articles as excellent or good
Learn more about the work of our research integrity team to safeguard the quality of each article we publish.
Find out more
REVIEW article
Front. Chem. Eng. , 12 October 2021
Sec. Environmental Chemical Engineering
Volume 3 - 2021 | https://doi.org/10.3389/fceng.2021.737710
Biofilm-based algal cultivation has many advantages over the conventional suspended growth methods and has received increased attention as a potential platform for algal production, wastewater treatment (nutrient removal), and a potential pathway to supply feedstock for microalgae-based biorefinery attempts. However, the attached cultivation by definition and application is a result of a complex interaction between the biotic and abiotic components involved. Therefore, the entire understanding of the biofilm nature is still a research challenge due to the need for real-time analysis of the system. In this review, the state of the art of biofilm definition, its life cycle, the proposed designs of bioreactors, screening of carrier materials, and non-destructive techniques for the study of biofilm formation and performance are summarized. Perspectives for future research needs are also discussed to provide a primary reference for the further development of microalgal biofilm systems.
Microalgae are a vast group of oxygen producing photosynthetic organisms that live using autotrophic, mixotrophic or heterotrophic metabolic strategies. Many of the microalgae species have been used for producing valuable bioactives since the 1950’s (Goodwin and Jamikorn, 1954). In recent decades, the requirements for environmental protection and renewable energy substitution have inspired the renaissance of research on microalgae (Mata et al., 2010; Wang et al., 2017).
Several studies have been focused on the use of microalgal biomass as a source of biofuel and other valuable products in biotechnology, i.e., food, cosmetic, aquaculture and pharmaceutical industries (Borowitzka, 1999; Spolaore et al., 2006; Mata et al., 2010; Wiley et al., 2011; Abdel-Raouf et al., 2012; Wijffels et al., 2013; Ravindran et al., 2016; Chen et al., 2018; Dixon and Wilken, 2018) as well as on the recovery of compounds, e.g., phosphorous (Mukherjee et al., 2015) and iodine (Han et al., 2016). Microalgae are considered living cell factories (Ravindran et al., 2016), and the ability of microalgae to adjust their metabolic activity to different culture conditions provides a wide range of opportunities for biotechnological applications (Bumbak et al., 2011; Mohammad Mirzaie et al., 2016). The ability to switch the biomass production from batch to continuous mode is another significant reason for implementing microalgal biofilms in bioprocesses since more efficient processes may be arranged (Strieth et al., 2018).
Microalgae are able to use polluted municipal or industrial wastewater as a growth medium, have lower nutrient requirements as compared to terrestrial crops (Boelee et al., 2011; Moreno Osorio et al., 2018) and can be cultivated on land not suitable for food production (Berner et al., 2015). Bio-product production from microalgae can be more environmentally sustainable, cost-effective and profitable, if these are coupled with processes such as wastewater treatment (Figure 1) (Ravindran et al., 2016). Even so these advantages, the production of microalgae for biofuel has been hardly scaled up due to it is not economically competitive compared to the production of other fuels (Christenson and Sims, 2011). The reasons for this include some challenges associated with industrial-scale production, particularly for bioproducts with a large market volume but low value (Abdel-Hameed and Hammouda, 2007; Gross et al., 2015); lower growth rates due to photoinhibition, contamination with less productive species, poor harvesting efficiency, mixing/transportation costs and high water requirement. These can all be traced back to a fundamental characteristic of microalgal suspended cultures: low biomass concentration leading to high harvesting costs (Richmond, 2004; Hu et al., 2008; Berner et al., 2015). In the last decade, natural photoautotrophic conditions for biomass production have been implemented with CO2 supplementation (Gupta et al., 2016) and considerable research is going on into improving cultivation of microalgae in suspension, both through increasing the biomass concentration and through improved process and bioreactor designs, especially more efficient biomass-recovery technologies (Acien et al., 2012; Berner et al., 2015). Besides, great interest lies in investigating a fundamentally different approach: the potential of attached cultivation of microalgae as biofilms (Irving and Allen, 2011).
FIGURE 1. Principles of production and recovery of valuable biomolecules using microalgae integrated with wastewater treatment. DSP: Downstream Processing.
Attached microalgae cultivation enlightened an alternative pathway to solve the problem of efficient production of microalgae biomass for industrial applications (Lee et al., 2014; Cheng et al., 2017; Wang et al., 2017). The key advantages of microalgal biofilms for biomass production are the higher biomass concentration (i.e., high number of cells per unit volume) and the simple way with which the attached cells can be separated from their surrounding growth medium (Abdel-Hameed and Hammouda, 2007; de-Bashan and Bashan, 2010; Ozkan et al., 2012) without using additional electrical energy or chemical methods (Johnson and Wen, 2010; Ozkan et al., 2012). The harvesting/dewatering process is simpler for biofilms as the cells can be easily scraped off the surface (Johnson and Wen, 2010; Shi et al., 2014) without requiring costly separation techniques like centrifugation or filtration, and there is no need to flocculate and settle/float the biomass (Christenson and Sims, 2011; Berner et al., 2015). High biomass concentration results in reduced cultivation medium requirements, compared to production of the same amount of biomass via suspended growth systems (Barros et al., 2015).
There are several recent reviews by worldwide leading research groups in microalgal attached cultivation (Table 1). Some reference reviews on the topic are those focused on the use of microalgal biofilms for wastewater treatment, especially removal of nitrogen, phosphorous and metal (Mallick, 2002; Wang et al., 2016a; Han et al., 2017; Mohsenpour et al., 2021), but a big amount of information about all these and other uses for algal biofilms still remains scattered.
TABLE 1. Compilation of featured informative published reviews in the research field of microalgae biofilms on topics as cultivation, reactor designs, analysis techniques, applications and promising potentialities.
Microalgal biofilm cultivation is an active research field in the early stage. The experimental results are promising compared to suspended microalgae cultivation. Considering that this review is focused on relevant aspects of current research and research not fully discussed before; it provides forward perspectives on emerging systems and attached microalgae cultivation.
Tampion and Tampion (1987) defined an immobilized cell as a living cell that, by natural or artificial means, is prevented from moving freely from its original location to all parts of an aqueous phase of a system. On the other hand, the term “biofilm” comprises both organisms growing attached to a surface and organisms which grow as aggregates, where the cells are sticked together by extracellular polymeric substances (EPS) (Strieth et al., 2018). These are molecules produced by both algae and other microorganisms in response to physiological stresses encountered in the natural environment (Romaní et al., 2008). EPS serve to protect the cell from the surrounding environment and to bind the cell to a surface. EPS are composed of polysaccharides, proteins, nucleic acids, and phospholipids (Sheng et al., 2010).
Besides these recent definitions, many terms have been used in the literature by different research fields during the last decades. This section will give a glance of the extensive work on the topic. Two common approaches are used for increasing cell mass concentrations inside reactors: one is the use of a permeable membrane to retain cells; and the second one is using immobilized cell techniques. Membranes allow retention of the cells, while the liquid, substrate, and product out pass through the reactor. Thus, high cell concentrations can be achieved (Gao et al., 2015). However, the interest in the use of entrapped biomass is increasing because reactors with these types of biomass retention may offer high reaction rates, and solve some drawbacks that membrane methods have, as harvesting and maintenance (Qureshi et al., 2005).
Six different immobilization types have been proposed: covalent coupling, affinity immobilization, adsorption, confinement in liquid–liquid emulsion, entrapment in polymers and capture behind semi-permeable membranes (Mallick, 2002). These types of immobilization can be grouped into two groups: “passive and active,” using the natural ability of microorganisms to attach to (natural or synthetic) substrates or using flocculant agents, chemical attachment, and gel encapsulation, respectively (Moreno-Garrido, 2008). Other authors grouped them in three techniques, namely, “adsorption, entrapment, and covalent bond formation” (Qureshi et al., 2005). These two definitions are compatible because the techniques cited by Qureshi et al. (2005) can be included in the groups defined by Moreno-Garrido (2008) (Figure 2). Nevertheless, it should be considered that in some cases it may be difficult to classify a certain method as belonging to a specific group.
The entrapment and covalent bond formation techniques are active immobilization techniques. They require the use of chemicals, i.e., flocculant agents, chemical attachment or gel entrapment. This increases the cost of production and restricts further propagation or increase in cell concentration inside the reactor. With this technique, the biomass grows around the particles or surrounded by them and the size of the granules grows with time usually to several mm in diameter (de-Bashan and Bashan, 2010). The polymer is mixed with the microalgae cells and consequently stabilized with divalent ions to form immobilized microalgae beads through a nozzle (Lam and Lee, 2012). The density of the support particles is higher than the growing broth and bioparticles thus tend to remain in the lower section of the reactor. Since the immobilized beads are relatively large in size compared free cell cultures, a simple filtration method (e.g., sieving) is sufficient to separate the beads from water without significant energy input. Hence, handling of microalgae biomass is easy and feasible to be implemented at commercial scale (Lam and Lee, 2012; Castro-Ceseña et al., 2015). Some immobilization media, such as alginates, carrageenans and polacrylamide gel have been used for several purposes (Muñoz et al., 2009; de-Bashan and Bashan, 2010; He and Xue, 2010; He et al., 2014; Gagliano et al., 2017). Immobilization based on these polymeric matrices provides on low mechanical strength and can result in restrictive diffusion of substrate (Qureshi et al., 2005; Moreno-Garrido, 2008).
Many microalgae, bacteria, archaea, fungi, and protozoa cells have a natural tendency to attach (adsorb/adhere) to surfaces and grow on them (Huang et al., 2016). This technique, called adsorption, has been extensively used to attach microbial cells and is what was defined by Moreno-Garrido (2008) as “passive” immobilization. Normally, these processes are easily reversible. Adsorbent materials (carriers) for passive immobilization can be natural or synthetic (Ozkan and Berberoglu, 2013a; Gross et al., 2015; Katarzyna et al., 2015; Podola et al., 2017). The application of surface-attached growing algae is an interesting option, where algae are continuously harvested or extracted to obtain target compounds. Katarzyna et al. (2015) categorized this kind of immobilization as non-enclosure methods. Wimpenny et al. (2000) grouped in the term “aggregates” those accumulations of microorganisms that are developed on surfaces and those on interfaces were named as “biofilms.” Biofilms form most commonly at water/solid interfaces, although it can appear at an interface between two immiscible liquids like oil or water, at air-water interfaces and at gas-solid surfaces (Wimpenny et al., 2000). Similarly, a definition of a biofilm as an “aggregate of microorganisms,” in which cells are frequently embedded within a self-produced matrix of EPS adhere to a surface and/or to each other, binding firmly in the form of flocs and aggregates, property known as autoflocculation (Gonzalez-Fernandez et al., 2012) i.e., granular biofilms (González-Fernández and Ballesteros, 2012), was proposed by other authors with the aim to provide terminology useful and non-confusable between different research fields (Vert et al., 2012).
Algal biofilms can be established on any surface that receives sufficient light and moisture. These films are complex communities of both autotrophic and heterotrophic organisms (Callow, 2000). Thus, these films can contain different microorganisms, from diatoms and cyanobacteria to bacteria and/or protozoa. As an alternative, the term “photosynthetic biofilm” is often used to differentiate these films from those where the main source of energy is a dissolved or solid substrate (heterotrophic). However, the terms “algal biofilm,” “photosynthetic biofilm,” and “microalgal biofilm” have been used interchangeably to describe films in which microalgae constitute a significant portion of the microbial community (Irving and Allen, 2011).
Microalgal biofilms, composed of cyanobacteria and/or green microalgae, are ubiquitously distributed in almost all the photic aquatic environments. An important attribute of biofilms is that they both create and are functionally controlled by gradients of energy sources and chemical products (Bernstein et al., 2014). A determinant point involved in attached cultivation as defined before is the need of distinguishing two basic sub-types of “algal biofilm” (Wang et al., 2017). The first type is the biofilm consisting of pure and single species of microalga (axenic culture). For this biofilm, the attached cultivation is initiated from the concentrated algal biomass inoculum that is pasted onto the carrier material (Cheng et al., 2013; Liu et al., 2013; Shi et al., 2014; Zhang et al., 2015; Cheng et al., 2017). In this case, the biofilm diversity keeps stable during cultivation and no obvious contamination by exotic organisms occurs. The second type of biofilm is related with biofouling (Katarzyna et al., 2015). It may also start from axenic microalgal biomass, however, during the growth process, allochthonous bacteria, fungi and cyanobacterial also settle inside the biofilm and it becomes a diverse microbial community (Wang et al., 2017). Therefore, Mantzorou and Ververidis (2019) defined that a biofilm could be characterized as a consortium of microorganisms, embedded in EPS, forming a complex structure which is developed on solid surfaces.
As was stated on the introduction of this review, among all biological systems, microalgae are on top of interest due to their biotechnological applications. Microalgae can be used in aqueous treatment processes (secondary and tertiary) for a range of purposes (Mohsenpour et al., 2021), some of which are: the removal of coliform bacteria, reduction in both chemical and biochemical oxygen demand, removal of phosphorous and/or nitrogen, as well as the removal of heavy metals (Agarwal et al., 2019) since they can further converting them to more amenable forms. Additionally, microalgae biofilms have promising applications on toxicity measurements (biosensors) (Brayner et al., 2011; Lam and Lee, 2012; Nandimandalam and Gude, 2019), CO2 capture (Hamano et al., 2017), as well as polycyclic aromatic hydrocarbons accumulation and degradation (Zhang et al., 2019).
Industrial applications including wastewater treatment have mainly employed biofilms where no support material is used and cells form biomass granules and flocs that also grow in size with time (Moreno-Garrido, 2008). This granular biofilm has been used in the so-called granular biofilm reactors. Granule formation may take from several weeks to several months. Thus, phototrophic biofilms of microalgae bring beneficial features within the whole process scale: i) the biological system tolerates higher inhibitor concentrations in the medium, what simplified the upstream processing; ii) a separate cell concentration step might be neglected, which could reduce drastically downstream costs and iii) the biological reaction step can be accomplished in a continuous mode of operation (Wimpenny et al., 2000; Buhmann et al., 2012; Han et al., 2017).
Algal biodiesel production is limited by the downstream cost of lipid extraction and the availability of water, CO2 and nutrient (Ravindran et al., 2016). However, algae biomass can also be used to produce biogas. This idea was first proposed by Golueke et al. (1957), and it continues to be a research field to which a great effort is devoted to increase its efficiency (Zabed et al., 2020). The coupled process of algae cultivation and succeeding biogas production is a better option compared to algal biodiesel production. A recommended solution for overcoming the high cost is to integrate algae attached-cultivation with an existing biogas plant, where algae can be cultivated using the digestate as nutrient input and discharges of CO2, and then the attained biomass can be converted directly to biogas or biomethane by the existing infrastructures (Wang et al., 2013; Zabed et al., 2020).
Single species biofilms are used to produce industrially important chemicals (Abdel-Hameed and Hammouda, 2007). For chemical production is preferred to control and maximize the production of desired target products (Qureshi et al., 2005), scenario that can be obtain with the inoculation of a single species into a sterile environment and allowed to form a biofilm before being used to produce a particular product (Zhang et al., 2015; Cheng et al., 2017). Production of high added value molecules by microalgae allows that forms of valorization, i.e., health food and quality feed, carbohydrates, polyunsaturated fatty acids, pigments (Dixon and Wilken, 2018), are currently penetrating their markets and reach economic competitiveness (Levasseur et al., 2020). Beyond its rich macronutrient composition, microalgae are able accumulate and to express secondary metabolites under stressful conditions (Mukherjee et al., 2015). This paves the way to targeted bioactive compounds production throw biofilm cultivation systems, and eventually penetrate cosmetics and pharmaceutical markets (Levasseur et al., 2020).
Despite the importance of microalgal biofilms in nature, their potential as biological indicators in wastewater systems, and the detrimental role they can play in fouling of surfaces, further research is still needed to fully understand the factors influencing microalgae biofilm development (Burns and Ryder, 2001; Ljaljevic-Grbic et al., 2010; Nováková and Neustupa, 2015; Novoa et al., 2021). This particularly contrasts to the extensive study that has been devoted to the formation of bacterial biofilms, e.g., Buckingham-Meyer et al. (2007) and Zeng et al. (2017), in particular using architectural non-destructive techniques such as continuous culture flow cells and confocal laser scanning microscopy (Barranguet et al., 2004; Zippel and Neu, 2005; Garny et al., 2008; Hille et al., 2009; Neu et al., 2010).
An innovative research area is the use of microalgal nanoparticles for enhanced removal efficiency, because physical and chemical methods used to obtain nanoparticles have some consequences due to their negative environmental impact, laborious production technique, and unaffordable cost (Dahoumane et al., 2016; Sharma et al., 2016). Nanoparticle synthesis through a biosynthetic route (via plant extracts or microorganisms) is a simpler technique and has attracted considerable interest globally (Agarwal et al., 2019; Benettoni et al., 2019).
Biomedical applications of algal nanoparticles comprise wound healing as well as antifungal, anticancer, and antibacterial activities. It has been reported that metallic nanoparticles (gold, silver, and copper oxide) synthesized using Bifurcaria bifurcata, Galaxaura elongata, Sargassum plagiophyllum, Caulerpa racemosa, Microcoleus sp. and Chlorococcum humicola have antibacterial activity against Gram-positive and Gram-negative bacteria (Agarwal et al., 2019). Benettoni et al. (2019) demonstrated the suitability of C. vulgaris biofilms for nanoparticles production and the way to study nanoparticles fate and localization in complex environments simultaneously using high resolution 3D visualization techniques. The current and future research trend is leading to the promising application of algal nanoparticles as antibiofilm agents against multidrug resistant bacteria due to their capability to penetrate EPS and cell membranes (Sharma et al., 2016). Moreover, they can be further explored in nanocomposites and biosensing applications (Agarwal et al., 2019).
Microalgal colonization on hard surfaces is a common phenomenon in natural aquatic and aero-terrestrial (Hallmann et al., 2013) environments which has both ecological and industrial significance (Sekar et al., 2004a; Roeselers et al., 2008; Ozkan and Berberoglu, 2013a; Sirmerova et al., 2013). In general, four stages of the development of a microbial mature biofilm can be observed: initial attachment (adsorption), irreversible attachment by the production of EPS (consolidation), early development (colonization), and maturation of biofilm architecture (Stoodley et al., 2002; Lewandowski and Boltz, 2011).
Colonization of a surface is characterized by the alternation from freely moving cells in a, aquous medium, to a sessile, immobile community adhering to a surface. This transition is determinant on the microbial composition from the beginning of biofilm establishment to its maturity (Berner et al., 2015). The concentration of glycoproteins, proteins, cations and organic compounds at the surface can provide a nutritive zone for microorganisms compared to the bulk aqueous environment. Furthermore, fluid flow at the interface allows microorganisms to approach the hard surface. Once near the surface, microorganisms either get in contact with the surface by chemotaxis or by Brownian motion towards the surface in response to the chemical concentration gradient, and then the cell will form a temporary association with the microbes already present on the surface (Qureshi et al., 2005; Roeselers et al., 2007).
The initial adhesion of microbial cells to solid materials is mainly determined by the cell–solid and cell–cell interactions based on the physicochemical properties of the interacting surfaces, such as the thermodynamic balance of interaction energies (Sirmerova et al., 2013). Initially, cells can displace towards the conditioned surface in many ways: they can be transported by gravity, be transferred by advection or actively move via motile mechanisms (Ozkan and Berberoglu, 2013a). Attachment of most marine algae is thought to be facilitated by its specialized reproductive propagules which allow initial contact with a solid surface. Motile spores have the ability to respond to external stimuli such as surface chemistry and texture. On the other hand, non-motile spores are led by gravity and by the adhesive properties of the extracellular residual mucilage. After this initial contact all spores secrete adhesive materials which allow a permanent attachment to be made (Fletcher and Callow, 1992).
In freshwater, the primary colonizers are considered to be mainly diatoms, which are unicellular or colonial algae (Romaní et al., 2008). The spontaneous, often reversible, adsorption of organic and inorganic aqueous molecules to the solid surface is followed by a non-reversible secondary attachment of cells through the production of EPS. This provides protection from any toxic compounds that could harm the cells and from phagocytes and bacteriocides by granting a diffusive barrier to biofilm cells (Barranguet et al., 2005; Qureshi et al., 2005). The binding strength of EPS found in Algogenic Organic Matter (AOM) occurs by hydrogen bonding, electrostatic interactions and van der Waals forces between functional groups (Ozkan and Berberoglu, 2013a). Once the coating and the first biofilm layer is established, the colonization for other organisms is eased by the modification of the initial surface properties of the material (Lobelle and Cunliffe, 2011).
The second attachment, based on cell−surface interactions, has led to numerous adaptation strategies; e.g., surface charge (Hori and Matsumoto, 2010) and hydrophobicity of the cell walls and membranes can be adjusted by forming surface structures (Krasowska and Sigler, 2014), such as pili, curli, fimbriae (Bullitt and Makowski, 1995; Lasaro et al., 2009), and flagella (Guttenplan and Kearns, 2013) or by regulating EPS production (Romaní et al., 2008), all of which may improve adhesion to an habitable solid surface, establishing a stronger binding of the algal cells forming colonies (Rummel et al., 2017).
The micro-colonies produce more EPS, which serves to maintain the growing biofilm on the surface and increase its thickness during the development phase (Figure 3) as EPS supports cell–cell communication (Toyofuku et al., 2016). The biofilm can be colonized by secondary organisms and serve as a sink for other particulate matter in the environment (Wimpenny et al., 2000). The architecture of the biofilm develops during this phase in response to shear forces. Biofilms can form thick mushroom-like masses in low shear environments and may be flatter or form long strands in high shear environments (Costerton et al., 1995). This structure is shaped mainly by heterogeneous matrices with clusters made of cells and polymers, randomly distributed along a three-dimensional (3D) architecture and surrounded by water channels that sometimes extend from the bottom to the top of the biofilm (Lewandowski and Beyenal, 2003; Melo, 2005; Petroff et al., 2011; Toyofuku et al., 2016).
FIGURE 3. Stages of biofilm development. (A) initial attachment, (B) irreversible attachment, (C) development/growth and (D) maturation/detachment. EPS, Extracellular Polymeric Substances.
In this development phase, the cells living in the biofilm take up nutrients, mainly towards production of EPS rather than to biomass growth. When nutrients become scarce, cells must emigrate from the EPS matrix. Biofilm associated cells are able to release enzymes capable of breaking down the EPS matrix in times of nutrient starvation (Qureshi et al., 2005). Biofilm development is intrinsically linked to the accumulation of cellular and polymeric biomass. The loss of small or larger areas of biofilm biomass, called biofilm detachment, has been recognized as a part of the biofilm life cycle decades ago (Chang et al., 1991). In addition to abiotic growing factors that have an impact on the biofilm architecture, i.e., chemical factors such as cell detachment due to starvation or nutrient deficiency (Hunt et al., 2004; Wijeyekoon et al., 2004), and physical factors, i.e., abrasion, shear stress, sloughing and grazing (Stoodley et al., 2002; Telgmann et al., 2004; Celmer et al., 2008), there are also biotic factors as the microbial metabolic activity (Allison et al., 1998) and microbial gene expression (Kaplan et al., 2004) that influence biofilm detachment. A balance between attachment, growth and detachment of biofilm is important in the production and maintenance of a functional biofilm community (Garny et al., 2008; Woodcock and Sloan, 2017).
Several parameters affect how quickly biofilms form and mature. Microalgal adhesion to a hard surface is one of the main steps of biofilm development and strongly depends on different factors that can be grouped according to the origin/place where the effect occurs as: surface (interface), environmental and cellular factors (Qureshi et al., 2005; Irving and Allen, 2011; Liao et al., 2015). On other hand, other authors have divided these factors regarding their nature into: physicochemical properties, biological factors and environmental conditions (Barros et al., 2018). Both types of aggrupation can be exchangeable, as biological factors are mainly cellular and physiological properties that mostly occur at the interface. Important interface factors are the surface charge, surface energy, chemical nature, hydrophobicity (wettability), lubricity and surface topography. Environmental variables affecting photosynthetic biofilm formation include light availability, pH, hydrodynamic conditions, nutrient quality and quantity, temperature, carbon source and the target surface for attachment (Tan et al., 2020). Cellular influences are the microalgae genetics, growth stage and metabolic activity (Irving and Allen, 2011). Although there are many studies focusing on factors that impact the biofilm formation, especially surface physico-chemical properties of microorganisms and surface materials, studies comparing metabolism and experiments under in vivo conditions are uncommon or even absent for microalgae and cyanobacteria (Sekar et al., 2004a; Irving and Allen, 2011; Ozkan and Berberoglu, 2013a; Sirmerova et al., 2013).
The effect of substrate material on the algal attachment and attached growth is a complicated process (Schnurr and Allen, 2015). The physico-chemical properties of material surface such as hydrophobicity (Finlay et al., 2002.; Ozkan and Berberoglu, 2013b), surface energy (Genin et al., 2014), and dispersive surface energy (Cui and Yuan, 2013) are relevant parameters for the initial algal colonization, but this highly depends on the materials and model strain used.
Hydrophobicity is a factor that affects the surface of the algal cell and substrate, for this reason considered an interface and a cellular factor. In diverse multi-species microbial biofilm, the presence of fimbriae, proteinaceous bacterial appendages rich in hydrophobic amino acids, can increase cell surface hydrophobicity (Barros et al., 2018). Flagellated cells show increased ability to attach to surfaces. Flagellar motility may serve to overcome initial electrostatic surface repulsion (Bullitt and Makowski, 1995; Qureshi et al., 2005; Krasowska and Sigler, 2014).
Some studies have shown that cell adhesion tends to occur preferably on hydrophobic surfaces (Sekar et al., 2004b; Ozkan and Berberoglu, 2013a). Ozkan and Berberoglu (2013a) reported differences in cell attachment between green algae and diatoms relating to the hydrophobicity of the surface. The principle is that hydrophobic molecules, cells and particles, prefer a hydrophobic environment and adhere to each other to minimize their contact with water (Palmer et al., 2007). A different micro-pattern of the material surface, i.e., texture or roughness of the material surface, affects cell attachment and retention (Ozkan and Berberoglu, 2013b; Gross et al., 2016; Huang et al., 2018). Cao et al. (2009) proposed that by increasing the surface texture (roughness), zones where the flow velocity is slower are created which allows algal cells to settle on the surface. The appropriate texture also minimizes the shear forces and reduces cell sloughing. It was demonstrated by Huang et al. (2018) and Kardel et al. (2018) that the shear stress on the surfaces with grooves was weaker than that on the surface without grooves, and differences for cells attachment were found between the different groove shapes tested. The initial attachment time was shortened under the hydraulic shear stress on the grooved surfaces compared to that of the surface without microgrooves.
The materials with an appropriate surface texture provide a “shelter” for the attached cells; as a result, the sloughing of the attached cells can be significantly reduced (Irving and Allen, 2011). Initial algal colonization on smooth surfaces was tested by Gross et al. (2016). The tetradecane contact angle of the materials had a good correlation with cell attachment. Therefore, porous materials work well for biofilm formation. Inside pores, the shear forces are very low even under conditions where bulk fluid velocity is high. Pores provide a protected environment for cells to attach and grow. Porous materials such as brick and bone char have been used to immobilize microbial cells used in biofilm reactors (Ozkan and Berberoglu, 2013a; Boelee et al., 2014), as well as textile materials (Christenson and Sims, 2012; Gross et al., 2013; Lahin et al., 2016; Carbone et al., 2017; Moreno Osorio et al., 2020). However, Hook et al. (2012) concluded that the hydrophobicity (wettability) and polymer topography did not affect the attachment of bacteria to synthetic polymeric substrates. Selection of an appropriate attachment material is important in the development of a microalgal biofilm system. However, only a limited number of materials has been studied so far (Gross et al., 2016).
Altering the substrate surface can drastically increase algal attachment (Kohler et al., 1999; Sekar et al., 2004a; Gross et al., 2016). The initial conditioning film may have the capacity to lead the colonizing community by altering the material-specific surface properties (Jones et al., 2007; Limoli et al., 2015) At the nano-scale, the behavior of particles in fluids is driven by the phenomenon of sorbed molecules and it is a key point for the rapid establishment of a coating layer consisting of proteins and other biomolecules around nanoparticles in biological fluids, e.g. cytoplasm and serum, that affects the physicochemical interaction of the materials with tissues and individual cells (Galloway et al., 2017). Lorite et al. (2011) concluded that the chemical nature of the aforementioned conditioning film appears to be more relevant for the settlement of organisms than the hydrophobicity or surface roughness of the initial substratum, which highlights the importance of this very first sorption process (Rummel et al., 2017).
Microbial cells have the ability to rapidly manipulate their production of EPS in response to changing environmental conditions (Latour, 2004). Thus, while hydrophobicity is one of the important factors in algal adhesion, it is not a simple matter to predict how the growth material affects algal EPS production (Genzer and Efimenko, 2006). Becker (1996) monitored the production of EPS by diatoms colonizing six different surfaces ranging from hydrophobic to hydrophilic ones. The organisms were able to colonize all surfaces tested with little difference between the amounts of EPS produced on a per cell basis. However, the nature of the polymer produced was not measured; it is possible that it changed from surface to surface. On the contrary, Arce et al. (2004) studied the attachment of a single diatom on intersleek and mica surfaces, determined from force versus distance curves. The attachment strongly depended on the individual Navicula cell and not on their growth stage, because the adhesion of Navicula sp. to surfaces with different physicochemical properties was governed by macromolecular specificity of diatom EPS; as Navicula diatoms secrete EPS with hydrophobic and hydrophilic properties. Recently, other studies have also demonstrated the relation between substrate and the production of EPS (Shen et al., 2015; Barros et al., 2018). Shen et al. (2015) tested two support materials and two medium compositions to evaluate the enhancement of biofilm formation. A significant effect of the culture period, nutrient, and substrate on the EPS production was observed. They concluded that the increase of EPS production enhanced the biofilm growth.
The research conducted on the effects of material properties on microalgal biofilm growth is inconclusive. Some researchers have demonstrated a correlation between biofilm formation and growth and hydrophobic surfaces. Specifically, they conclude the suitability of hydrophobic surfaces for the growth of biofilms (Finlay et al., 2002; Ozkan et al., 2012; Ozkan and Berberoglu, 2013a). Other researchers, on the contrary, have demonstrated that hydrophilic materials with good liquid-holding capacity can be preferable for the attachment cultivation (Zhang et al., 2015). Many algal species belonging to the genera Chlorella, Chroococcus, Chlorosarcinopsis, Synechococcus, and Scenedesmus could be immobilized by hydrophilic polymers (Zeng et al., 2015). In contrast, other authors have found no or only weak correlations between hydrophobicity and microalgal biofilm formation and growth (Irving and Allen, 2011; Genin et al., 2014). These contrasting results could be due to different length of cultivation time and cell density of biofilm adopted in the experiments. Researchers reporting an effect of material properties on growth mainly study the initial biofilm adhesion (Schnurr and Allen, 2015). However, other factors, such as microbial species and culture medium, can play an imperative role on microalgal attachment to a surface (Becker, 1996; Johnson and Wen, 2010; Irving and Allen, 2011).
Biofilm structure and species distribution can vary considerably depending of environmental factors and culture conditions (Irving and Allen, 2011). Therefore, biofilm structure is not simple and uniform, but instead it dynamically changes depending on environmental conditions. Biofilm formation has been suggested to be a microbial preparation for unstable environmental conditions (Boles and Singh, 2008; Toyofuku et al., 2016). However, biofilms can also proliferate in nutrient-rich, stable-flowing streams, causing water management problems (Zippel et al., 2007). The hydrodynamics and composition of the external liquid are important factors affecting the biofilm formation and physical structure changes (Vieira et al., 1993; Beyenal and Lewandowski, 2000; Barberousse et al., 2007). Mechanical forces, in particular shear develop in many natural environments where liquids, generally water, are flowing across substrata surfaces. The strength of adhesion is determined by subjecting the cells to a shear force and determining the percentage remaining after a certain period of time (Schultz et al., 2000). Shear forces are lower near a rough surface, and there is a larger surface area to which cells can adhere (Qureshi et al., 2005). Strength of adhesion varies with microalgae strain and substrate surface, but substantial numbers of algal cells have been reported to remain attached at shear rates of 200 s−1 or more (Barberousse et al., 2007). Besemer et al. (2007) showed that turbulent flow conditions favored the growth of filamentous green algae, while single-celled green algae were better able to thrive under laminar conditions.
The amount of nutrients present in the medium can affect the rate of biofilm formation. Biofilm formation tends to be more readily in the presence of adequate nutrient concentrations, e.g., phosphorus is a particularly important nutrient. Saturation with phosphate increases the tendency of cells to flocculate and adhere due to their raised hydrophobicity, while cells depleted in phosphate are more hydrophilic and less likely to adhere (Qureshi et al., 2005). Similarly, in other studies on diatom and green algae species, Domozych (2007) and Shen et al. (2015) demonstrated that EPS production increases by incrementing nutrient concentrations, particularly nitrogen. As a significant fraction of EPS is composed of proteins (Shen et al., 2015), and while nitrogen is abundant and environmental conditions are favorable, algae can unrestrictedly produce aminoacids. Evidence suggests that algal cells increase their EPS production along their colonies age and maturity (Becker, 1996). This could be due to mature colonies investing less resources into reproduction, i.e., reaching a stationary growth phase, and more into stabilizing their biofilm community (Schnurr and Allen, 2015). A pioneer study demonstrated that the colony size directly correlated with the substrate concentration in the flowing medium; showing that the substrate concentration regulates the microbial colony size, i.e., growth and detachment of cells (Szewzyk and Schink, 1987). van Loosdrecht et al. (1995) reported that biofilm structure was related to both substrate concentration and hydrodynamic conditions. Although the authors considered substrate concentration and shear force as two dependent factors, it can be preferable to consider these two as separate factors (Wimpenny et al., 2000).
Temperature can have an effect on biofilm formation. Depending upon the species involved, high temperature increases the rate of cell growth, surface adhesion, and EPS production, all of which enhance biofilm formation (Qureshi et al., 2005). Biofilms cultivated under a thin water layer are more sensitive to ambient temperature fluctuations than conventional suspended cultures (Posadas et al., 2013). Domozych (2007) reported that temperature stress and mineral (calcium) accumulation stress adversely affect EPS production from algal cells. The calculations in the study of Fica and Sims (2016) indicated that both an increase in temperature of the wastewater (7–27°C) and an increase in the level of organic carbon (300–1,200 mg L−1) contributed significantly to the increase of the biomass growth rate in the algae-based biofilm system.
Zippel and Neu (2005) observed that biofilms developed under high irradiance were thick (<900 nm), loosely structured, and dominated by Scenedesmus-like green algae. In contrast, those grown under low irradiance using the same inoculum were thinner, more compact and included a wider variety of species, including cyanobacteria. Hultberg et al. (2014) demonstrated the direct effect of light quality using monochromatic illumination in biofilm formation; indicating that light quality can improve cellular growth and lipid content at the same time. Furthermore, the authors concluded that the optimal wavelength depends on the species and showed the potential for using light as a tool for managing algal biofilm formation and harvesting.
From the cellular point of view, the genome, which determines the biofilm developmental potential of the organism, is one of the most important determinants of the biofilm structure. Thus, filamentous organisms might be expected to form a different multicellular array than non-motile coccoid organisms. Biofilms can be mainly regarded as approximately planar systems which include micro-colonies and more or less abundant EPS, which differentiates them from clearly filamentous structures (Wimpenny et al., 2000). Kokare et al. (2009) proposed that bacteria in biofilms express different phenotypic characteristics from planktonic counterparts. This is due to different genes transcribed in the planktonic and biofilm associated phases of the bacterial life cycle. Some genes may be expressed in response to a specific surface on which bacteria settle (Becker et al., 2001), e.g., genes involved in the attachment to chitin diverge from those required for attachment to abiotic, non-nutritive, surfaces such as glass or plastic (Keyhani and Roseman, 1996). Research on the role of metabolic and gene expression in microalgae biofilm formation is still a wide research field to explore, which can bring novel strategies to improve and enhance microalgae biofilm systems.
As it has been highlighted above, the material of the surface where the microalgal cells attach has an important effect on the biofilm formation. The ideal supporting material for large scale cultivation should be cheap, non-toxic, durable, porous/rough texture and show proper water retention ability. Currently most of the substratum materials used on laboratory studies are from local markets (Wang et al., 2017). Supplementary Table S1 provides a comprehensive summary of the different carrier materials used on microalgal biofilm systems. In this review, based on its origin and its degradability of “substrate,” “support” or “carrier” materials, we categorized them as artificial/synthetic and natural materials (Supplementary Table S1), similar as other authors have defined, bio-materials and non-bio-materials (Han et al., 2017). The term “carrier” will be exclusively used in this review.
Considering the entrapped materials for biofilm formation, immobilized S. obliquus cells in polyvinyl and polyurethane were cultivated in order to remove nitrate from wastewater (Urrutia et al., 1995). Bench scale studies were performed using rotating disks of aluminum (Torpey et al., 1971) or polystyrene (Przytocka-Jusiak et al., 1984) to grow algae biofilms and reduce phosphorus and nitrogen levels in wastewater. The Algal Turf Scrubber (ATS) was developed by Adey and associates at the Smithsonian Institution (Washington D.C., United States) as a more natural means of maintaining water quality in model ecosystems (Adey et al., 1993). Plastic mesh has been frequently used to grow filamentous algae in a ATS by intermittently pouring water over the surface (Adey et al., 2011).
Some researchers have studied the differences in biofilm growth with different synthetic materials without any material property measurement, and differences in growth rates were found. Some representative studies of enclosed biofilm bioreactors screening carrier materials are for instance, the work of Sekar et al. (2004b) who used two species of green algae: Chlorella vulgaris and Nitzschia amphibian. Both green algae adhered most readily to titanium, stainless steel perspex and glass, while brass and copper showed fewer attached cells. Subsequent experiments with N. amphibian showed that cells were more likely to adhere to surfaces that had been roughened with sandpaper than the smooth control. Likewise, Johnson and Wen (2010) studied biofilm growth rates on six different carriers (Supplementary Table S1). They observed that polystyrene foam yielded significantly higher biomass productivities than the other materials tested. On the other hand, Genin et al. (2014) examined six materials for their capability of supporting algal growth in a parallel airlift bioreactor. In this case, cellulose acetate showed the highest biomass productivity. Shen et al. (2014) evaluated the microalgal biofilm formation on nine carrier materials and the higher biomass productivity was observed using glass fiber reinforced plastic.
Christenson and Sims (2012) tested eight synthetic and natural carrier materials for the ability to support algal attachment and found that cotton rope showed the best performance. Gross et al. (2013) screened 16 potential biofilm carriers and found that cotton duct was the best material for microalgal biofilm growth. Likewise, (Moreno Osorio et al., 2019b; Moreno Osorio et al., 2020) reported efficient attached microalgal growth of C. vulgaris ACUF_802 and ACUF_809 as well as to S. vacuolatus ACUF_053 on cotton fabric. Similarly, Chuah et al. (2020) found that attached growth of C. vulgaris on cotton duct served as a better supporting material than polystyrene foam as it promoted microalgae attachment at low-cost, supporting an easy microalgae harvesting process.
An extensive and detailed study was performed by Gross et al. (2016), 33 synthetic materials with smooth surface were tested. The materials were grouped as metals, plastics and rubbers to evaluate their surface physico-chemical properties. Similarly, Barros et al. (2018) studied the influence of physicochemical properties of selected microalgae strains, growth medium composition and six carrier materials, using a lab scale methodology that allowed to evaluate multivariables in parallel.
Other authors used the same type of bioreactor configuration when testing different substrate materials. Naumann et al. (2013) developed a solid-state bioreactor, denominated “twin layer system” that combines a nutrient substrate and works as a support layer of an attachment surface where the biofilm grows. They evaluated the biofilm formation performance of four microalgae strains on plain printed paper. They concluded that Phaedactylum tricornutum has the higher biomass productivity (Supplementary Table S1). Complementary, (Shi et al., 2014) tested nylon filter sheets as attachment substrate material for the biofilm formation of Hallochlorella rubescens.
Studies for testing materials with different surface textures have demonstrated that the patterns on the substrate cannot only offer larger areas for cell attachment, but also act as anchor points sheltering cells from shear stresses (Huang et al., 2018). As expected, higher biomass productivity is achieved on materials with relatively larger surface area. Cao et al. (2009) confirmed that micro-dimple surface features of corrosion resistant steel sheets could significantly enhance the attachment of algal cells to the carrier, compared to a surface without microdimple features.
Additional materials have been used at laboratory scale to screen the biofilm formation capability of microbial consortia or microalgae axenic cultures, most of them aiming for the selection of a cheap and easily produced surface material that could be applied at large-scale in-land systems. The system used by Ozkan et al. (2012) is notable for its use of concrete as a cultivation surface, which can mean a significant reduction in the maintenance cost. The same material was used by Sukacova et al. (2015) with effective results. Locally produced materials as glass (Schnurr et al., 2013), foam PVC with surfaces sanded in order to promote microbial attachment and polyvinyl chloride (Posadas et al., 2013) were selected based on availability, non-toxicity, ease of manipulation and costs.
Various types of natural materials have been used as carriers for the microalgal biofilm cultivation (Supplementary Table S1). The advantages of a natural carrier material are the low cost and the environmental sustainability of the biofilm system, especially compared to the immobilization technologies where (although these can employ natural polymers, i.e., alginate and carrageenan), the high cost of the polymeric matrix prohibits their use at large scale (Hoffmann, 1998; Christenson and Sims, 2011). Several immobilization media, such as alginates, carrageenans and polacrylamide gel have been used for entrapped the microbial biomass (Moreno-Garrido, 2008). Akhtar et al. (2003) and Akhtar et al. (2008) provided an immobilized microalgal biosorption process using a highly porous, ridged, physically strong and low cost immobilization matrix (luffa sponge) that consists of discs obtained from matured dried fruit of Luffa cylindrica for the removal of Ni(II) and Cr (III) using Chlorella sorokiniana as model organism. Surface immobilization of the microalgae on luffa sponge, providing direct contact of the biomass to the metal solution, was better suited for metal biosorption than microalgae cells enclosed or beaded immobilized in a polymeric gel structure.
Filter paper was used as carrier material for algal growth vertically, and differences in biomass productivity were observed between three microalgae biofilms (4.2–6.1 m−2 d−1) (Naumann et al., 2013). Zhang et al. (2017) explored the feasibility of using lignocellulosic materials as biofilm carriers, which had a similar chemical composition to the algal cell wall. Of the materials tested, pine sawdust as carrier achieved high biomass productivity (10.2 g m−2 d−1). Lignocellulosic-based materials are generally characterized by a high surface energy and achieved greater attachment than synthetic polymers which have a low surface energy (Christenson and Sims, 2012). Moreover, lignocellulosic materials are hydrophilic natural polymers and have a good liquid-holding capacity (Zhang et al., 2017). The biofilm productivity was found to be increased with the increase of material surface roughness (p < 0.05) (Cao et al., 2009). Fica and Sims (2016) characterized the biofilm formation and nutrient removal of a microbial consortium dominated by algae species. The biofilm was grown on cotton cords in a Rotating Algal Biofilm Reactors (RABR) as described by Christenson and Sims (2012). They found that different concentrations of total organic carbon and temperature influenced the biomass productivity.
The rotating algal biofilm system proposed by Gross et al. (2013) was a conveyor belt system that had cotton as carrier material. The carrier moved around several rotating shafts, passing through a reservoir of nutrient-rich liquid at the bottom. Cotton duct promoted a high biofilm productivity in other studies with different alga strains (Christenson and Sims, 2012; Gross et al., 2016; Zhuang et al., 2016; Carbone et al., 2017). A clinoptilolite rotating photobioreactor (cRPB) was used by Young (2011) for removal of nitrate and ammonium from synthetic wastewater, the zeolite surface can be bio-regenerated through continued nitrogen removal. A microbial consortia biofilm productivity of 12 m−2 d−1 was obtained. Two component biodegradable carriers for Biofilm Airlift Suspension (BAS) reactors were used to investigate the oxygen transport inside the biofilm and the development of the biofilm structure (Hille et al., 2009). The carriers were composed of PHB (polyhydroxybutyrate), which is easily degradable, and PCL (caprolactone), which is less easily degradable by heterotrophic microorganisms (Hille et al., 2009).
Talukder et al. (2014) studied for the first time the immobilization of microalgae on exogenous fungal mycelium. Mycelium is a natural carrier material that can be obtained free or at low-cost as waste from fungal fermentation processes that produce valuable products, i.e., enzymes or soy sauce. The positively charged hyphae interact with the negatively charged algal cell surface and cause flocculation (Alam et al., 2016). The occurrence of filamentous microorganisms could thus be considered as a beneficial factor for microalgal biofilm cultivation by the promotion of algal cell attachment on the substrate layer. Meanwhile, respiration of the microorganisms could enhance algal productivity by decreasing O2 inhibition of algal growth (Bai et al., 2015).
Biofilm-based production of microalgae is gaining more attention as a proven alternative to traditional suspension cultures. There are an increasing number of systems with biomass production and controlled cultivation of a single target species as their primary focus that has been proposed in the literature, and most of them still retain strong links to wastewater treatment as coupled application. Several new and innovative designs have the potential to fundamentally change the approach to microalgal cultivation and many have been extensively reviewed previously (Kesaano and Sims, 2014; Berner et al., 2015; Gross et al., 2015; Hoh et al., 2016; Han et al., 2017; Mantzorou and Ververidis, 2019; Zuccaro et al., 2020).
Microalgal biofilm reactors were first reported in the 1970’s (Torpey et al., 1971) with a rotating aluminum disk design used for removing phosphorus and nitrogen from municipal wastewater. Several microalgal biofilm reactors have been reported (Supplementary Table S1) with applications in wastewater treatment (Mulbry and Wilkie, 2001; Adey et al., 2011; Posadas et al., 2013; Kesaano and Sims, 2014; Gao et al., 2015) and biomass production (Gross et al., 2013; Liu et al., 2013; Blanken et al., 2014).
“Algal biofilm-based cultivation” systems have been classified by Berner et al. (2015) into three irrigation procedures or relative position of the cultivation medium and the microalgae on the cultivation surface: constantly submerged, intermittently submerged, and perfused systems. In the first two categories, the microalgae are directly submerged in the growth medium, either all the time or some of the time (Mantzorou and Ververidis, 2019). Perfused systems use a porous substrate that supplies nutrients and moisture to the microalgae which grow on the outside, exposed to the surrounding gas phase (Podola et al., 2017). The advantages of perfused systems are i) ease of harvesting, algal cells can be harvested from a surface by biofilm scraping; ii) direct contact to atmospheric CO2, the cells on a solid surface can thus absorb CO2 directly from the atmosphere; and iii) light exposition, to maximize the capture of solar radiation, the surface plane of the substratum can be oriented in the best direction, and inclined at the optimal angle for the highest photosynthetic quantum yield to maximize biomass productivity per horizontal footprint area (Hamano et al., 2017). Gross et al. (2015) classified the different types of algal biofilm systems based on the relative movement of the algal biofilm to the liquid medium, i.e., rotating biofilm or stationary.
Hoh et al. (2016) categorized the biofilm reactor designs for wastewater treatment according to their configuration as horizontal, vertical, flow cell, rotating, and rocker bioreactors. The rocker reactors in the horizontal category and “floating” category are included in this review as they are still widely used biofilm (immobilized) configurations in wastewater treatment. The horizontal biofilm reactor has the advantage of effective absorption of light for photosynthesis. Similarly, the flow lane biofilm reactor, which uses water flow-over the biofilm growing on the carrier materials allows to switch different carriers for biofilm attachment into the flow lanes (Zippel et al., 2007). However, a large surface area is required in order to increase biomass productivity and wastewater treatment efficiency (Sukacova et al., 2015). The most typical vertical biofilm reactor is the twin layer vertical reactor. The two-layer vertical configuration helps to deliver nutrients to the microalgae (Podola et al., 2017), similar to perfused systems defined by Berner et al. (2015). Recently the rotating biofilm reactor has been intensively studied. Different rotating designs have been developed, offering different patterns of contact between the biofilm, liquid and atmospheric phases (Gross et al., 2015).
Moving bed biofilm reactors (MBBR) are common floating systems when the wastewater treatment plant needs to have a strongly limited footprint (Zkeri et al., 2021). Two systems were compared for medium-strength dairy wastewater treatment: i) a methanogenic Moving Bed Biofilm Reactor (AnMBBR) and an aerobic MBBR (AeMBBR), ii) an AnMBBR and a sequencing batch reactor (SBR) with C. sorokiniana. The coupling of a microalgae reactor with a methanogenic MBBR results in complete elimination of COD, but lower removal of NH4+-N (65%), TKN (69%) and PO43--P (31%). This effluent can be used for agricultural irrigation due to the high concentrations of contained N and P (Zkeri et al., 2021). Another study using floating reactors, the role of bacterial EPS on microalgae biofilm formation was evaluated using coated cotton carriers in a suspended-solid phase photobioreactor (sspBR). Their results showed that pre-coating significantly improved the attachment of microalgae cells (Zhuang et al., 2016).
Various microalgal biofilm systems have been developed over the past few years. Depending on the application of the system, the attachment materials (Supplementary Table S1), design rationale and configuration geometry (Table 2) employed have varied widely. The geometries of the microalgal biofilm systems are also highly variable, from a flat plate to a rotating drum (Gross et al., 2015). Some biofilm systems use carriers with many crevasses that generate additional attachment area, but this additional area does not have light contact. This system is commonly used in wastewater treatment, with a consortium of algae and bacteria involved (Fitch and England, 2002). The biomass in this system is not harvested but rather sloughed off into the liquid (Hassard et al., 2015). In contrast, the application of flat attachment materials allows maximal light exposure to the biofilm and biomass in these systems can be easily harvested. The algal cells in the biofilm are predominant due to the favorable conditions for photosynthesis, although some level of bacterial contamination has been reported, especially when microalgal biofilms grow in wastewater containing organic carbon (Pohlon et al., 2009; Irving and Allen, 2011).
A real time assessment of the biofilm structure and distribution of microorganisms in the matrix is compulsory for ecological and environmental studies (Wimpenny et al., 2000). Recent technologies focus on offer non-destructive techniques for biofilm studies (Fanesi et al., 2019; Saccomano et al., 2021). The matrix components and spatial arrangement of microorganisms define the number and size of voids and channels, altering in turn the transport of nutrients and gases. Therefore, the biofilm architecture induces marked gradients of light, nutrients and gases, along depth, inducing the cells to displace or acclimate in order to maintain optimal growth (Stewart and Franklin, 2008). Thus, structural data are of major importance to improve the productivity of biofilm-based technologies through a better understanding of the complex behavior of biofilms, i.e., development and activity (Fanesi et al., 2019).
The simple identification of the microbial groups is not informative enough, as the microbial activity is influenced by the biofilm spatial structure (Barranguet et al., 2004). Many different methods to characterize the properties of biofilms including detection, imaging, and sensing methods have been proposed in the literature (Neu and Lawrence, 2015). Sensing and imaging differ from each other in that sensing refers to the quantifiable measurement of some analyte or property of the subject or system, while imaging is used to generate visual information (topography, area, colour) related to a subject, and some combine it with the detection of analytes or even concentration measurements (Saccomano et al., 2021).
Techniques that do not disrupt biofilm structure and its microgradients have been given priority for understanding biofilm formation and development (de Beer et al., 1993; Zippel and Neu, 2005). Non-destructive methods for examining photosynthetic biofilms (Table 3) include various forms of microscopy and chemical imaging: simple light microscopy, Helium Ion Microscopy (HIM) (Schmidt et al., 2021); Scanning Electron Microscopy with Energy Dispersive X-ray analysis (SEM-EDX) (Moreno Osorio et al., 2019a), Confocal Laser Scanning Microscopy (CLSM) (Neu and Lawrence, 2014; Moreno Osorio et al., 2020), Confocal Raman Microscopy (CRM1) (Moudříková et al., 2017; Keleştemur et al., 2018), Pulse Amplitude Modulation (PAM) (Wimpenny et al., 2000; Moreno Osorio et al., 2020), Vibrational Spectroscopy (ATR-FTIR spectroscopy) (Fanesi et al., 2019), Time-of-Flight Secondary Ion Mass Spectrometry (ToF-SIMS) (Benettoni et al., 2019) and imaging analysis (De Muynck et al., 2009).
An increasing trend in nanoscale research is the combination of more than two techniques (high resolution microscopy + chemical imaging) to analyze different parameters in parallel to greatly improving the sensitivity and spatial resolution to visualize and quantify nutrients, metabolites and toxic elements in single cells at the subcellular and cell to cell level. Many of these are considered emerging imaging technologies that can be combined to correlate elemental, isotopic and molecular information from a region of the cell or from the entire cell in two or three dimensions (Decelle et al., 2020).
Optical methods have arisen as promising techniques in biofilm studies. Although biofilms can be observed with an unaided eye, imaging their structure, distribution of EPS and microbial community structure requires the use of several types of microscopy instruments combined with various probes, such as fluorescent proteins (FPs) used as reporter genes and labels or fluorescent in situ hybridization (FISH) probes (Azeredo et al., 2017).
HIM resembles a field-emission scanning electron microscope (FE-SEM) in many aspects, but several advantages are provided by the use of helium ions rather than electrons, including higher surface sensitivity, larger depth of field and a straightforward charge-compensating electron flood gun, which enables imaging of non-conductive samples, rendering HIM a promising high-resolution imaging technique for biological samples. The key remarkable capabilities of HIM in bio-imaging, in terms of spatial resolution, very large depth of field, and the ability to image non-conductive samples as biofilms without the application of conductive coatings support the growth in the number of studies which has been published in related fields and the opportunity for further developments (Schmidt et al., 2021). However, every problem in microscopy cannot be solved by HIM alone. In subcellular chemical imaging, for instance, correlative workflows combining nanoanalytics (e.g., X-ray spectroscopy and SIMS) and high-resolution electron microscopy are currently being established (Decelle et al., 2020).
In ToF-SIMS, a release of atoms from the outer few nanometers of the surface is caused by the bombarding with ions of the sample surface, and their identification is based on their mass. In SIMS imaging, high resolution in the mass spectrum comes at the price of lower image spatial resolution. However, development of the Nanoscale Secondary Ion Mass Spectrometry (NanoSIMS) allowed imaging at sub-micron resolution while maintaining high mass resolution (Azeredo et al., 2017). LeTourneau et al. (2018) used SEM, HIM, and NanoSIMS as correlate imaging tools to show that phenazine-1-carboxylic acid, produced by rhizobacteria in unirrigated wheat fields, and soil moisture promotes biofilm formation at root surfaces. The stable isotope labelling in combination with NanoSIMS, electron microscopy and HIM was used to study the carbon and nitrogen cycles. SIMS has demonstrated to be a powerful tool to study the unicellular and cell to cell interaction of microalgae (de Bashan et al., 2016) that make it a promising technique for the characterization of nanoparticles in biological media. In fact, high spatial resolution, its sensitivity and mass resolution offer label-free chemical 3D imaging capabilities down to the nanometer scale. Benettoni et al. (2019) used ToF-SIMS in an experiment on C. vulgaris biofilms to confirm the presence of TiO2 nanoparticles and to clarify their 3D distribution within this complex system. Similarly, Moreno Osorio et al. (2019) performed ToF-SIMS analyses on biofilm samples to analyze the distribution of phosphorus-containing molecular species in Chlorella pyrenoidosa and C. vulgaris biofilms. Furthermore, the high resolution and the wide range of molecular species that can be 3D-detected with this imaging technique allowed to understand the role of the cotton fabric as algal biofilm support material and confirmed that the cotton carrier does not accumulate phosphates and thus phosphate removal was the result of biofilm metabolic activity.
The favorite types of microscopies among biofilm researchers are those that allow the examination of living and fully hydrated biofilms. In addition, sophisticated image acquisition devices that can selectively image and stimulate various probes are often required when more than one type of multicolored probe is used simultaneously (Lewandowski and Boltz, 2011). Among them, CLSM enables the in vivo reconstitution of the 3D structure of microbial biofilms in their naturally hydrated form (Zippel and Neu, 2005). CLSM provides simultaneous identification of the different components and the information about the 3D structure of the biofilms, either by using specific fluorescent dyes for bacterial DNA or EPS glycoconjugates or by algal autofluorescence (Staudt et al., 2004; Neu et al., 2010; Neu and Lawrence, 2015).
Most of the understanding about biofilm architecture and composition (Chen et al., 2007; Neu and Lawrence, 2014) as well as their relation to mass transfer (Yawata et al., 2010) and metabolism is gained from rather simple CLSM point scanners. Several studies have been carried out using CLSM as informative technique for microalgae biofilm formation performance (Norton et al., 1998; Barranguet et al., 2004; Mueller et al., 2006; Blanken et al., 2014; Cole et al., 2014; Sommerfeld Ross et al., 2014; Zhang et al., 2017). A set of computational tools for extraction of the biofilm morphology from the 3D structural data obtained from CLSM imaging, and the accompanying web-based interface was described by Xavier et al. (2003).
A variation of the reflection microscopy technique employing a CLSM is defined as confocal reflection microscopy (CRM2). This technique allows the visualization of 3D samples without fluorescent labeling and fixation. Yawata et al. (2010) achieved a successful non-destructive monitoring of the entire biofilm development of Streptococcus mutans, on microfluidic-device experiments by a modified CRM2 technique. This modified version of CRM2 with continuous gain adjustment was termed Continuous Optimizing CRM (COCRM) and the mean biovolume measured showed good correlation with those calculated based on SYTO9 staining.
Biofilms are of outstanding interest for biological wastewater treatment, however in situ and real time techniques for analyses of biofilm systems (aerobic and anaerobic) are still limited. In contrast to invasive techniques for imaging analysis, such as FISH, Patzold et al. (2006) were able to identify anaerobically ammonium-oxidising (anammox) bacteria without pretreatment in samples just by its Raman vibrational signature. The presented results provided new insights into the complex interactions of different organisms in microbial communities without interfering with them. On the other hand, analytical techniques used to investigate the presence of lipids in the selection of microalgae for the optimal production of biofuels include Gas Chromatography–mass spectrometry - GCMS (Bigelow et al., 2011), Liquid chromatography–mass spectrometry - LCMS (MacDougall et al., 2011), Nuclear magnetic resonance – NMR (Nuzzo et al., 2013), Fourier-Transform Infrared Spectroscopy - FTIR (Dean et al., 2010), and Raman spectroscopy (Wei et al., 2014). Confocal Raman spectroscopy on the other hand, allows access to full spectral information with high spatial resolution (Sharma et al., 2015). Combination of Raman spectroscopy with confocal optical microscopy resulting in 3D spatial characterization of the samples has resulted in CRM1 becoming the method of choice for label-free and real-time monitoring of various biological samples, including microalgae and bacterial biofilms (Murdock et al., 2008; Efeoglu and Culha, 2013; Wei et al., 2014; Moudříková et al., 2017; Keleştemur et al., 2018).
The pioneer study of Valladares Linares et al. (2016) assessed three novel in-situ biofouling characterization techniques on membrane systems: i) nuclear magnetic resonance (NMR), ii) oxygen imaging with planar optodes, and iii) optical coherence tomography (OCT). These non-destructive tools can elucidate the interaction of hydrodynamics and mass transport on biofilm accumulation in membrane systems. OCT is a key emerging imaging technique of the last decade in biofilm research. Remarkable features of this technique are the in-situ application, the real-time-acquisition of multi-dimensional datasets as well as the fact that no sample preparation is necessary allowing for a non-invasive and complete characterization of an unaltered biofilm structure (Wagner and Horn, 2017). A significant advantage of OCT against other microscopy imaging techniques is the availability of investigating biofilms directly inside the cultivation device (e.g., biofilm reactor) under operational conditions. 2D and 3D datasets contain a representative description of the overall biofilm structure (Wagner and Horn, 2017). In-situ characterization using NMR techniques were successfully applied for non-invasive online monitoring of biofilm development, sloughing, forced detachment, and chemical cleaning. It allows quantification and visualization of development of biofilm and the cell interactions with the surrounding fluid at the mesoscale (McLean et al., 2008; Wagner et al., 2010).
Planar optodes use luminescent O2 indicators immobilized in an O2 permeable polymeric matrix, which can be coated on glass or foils surfaces. The principle is based on measuring the dynamic collisional quenching of the indicator luminescence by O2 (DeGraff and Demas, 2005). Optical O2 sensing was first developed in the medical field for blood gas analysis and was introduced in aquatic microbiology when fiber-optic O2 microsensors were developed and applied to biofilm studies (Kühl et al., 2007; Neu and Lawrence, 2015; Khosravi et al., 2020; Saccomano et al., 2021).
In microalgal biofilms, PAM fluorimetry allows the measurement of algal biomass on undisturbed biofilm samples, maximum quantum yield and algal photosynthetic activity (Genty et al., 1989; Barranguet and Kromkamp, 2000). Variable fluorescence emerged as a promising and non-intrusive tool to estimate photosynthesis in phytoplankton (Kolber et al., 1998). The principle of variable fluorescence is also used by PAM fluorimeters (Schreiber et al., 1995) and this technique has been used to measure algal photosynthesis, although mainly on laboratory cultures. Increasing applications of PAM fluorimetry for biofilm studies have been reported in the literature of the past decade (Eggert et al., 2006; Häubner et al., 2006; Malapascua et al., 2014; Manso et al., 2014; Ng et al., 2014; Schuurmans et al., 2015; Wang et al., 2016b).
De Muynck et al. (2009) evaluated the algal fouling on concrete by means of colorimetric and image analysis. The fouling intensity parameter and the image analysis is based upon the quantification of the area covered by algae by means of threshold analysis on the a* (green to red axis) and b* (blue to yellow axis) coordinates of the CIELab colour space of ImageJ software (Rasband, 1997–2008). This analysis allows a clear distinction between fouled and unfouled areas of concrete. Imaging analysis has been an effective tool to follow the biofilm growth in external facades and monuments (Sanmartin et al., 2010; Fernandez-Silva et al., 2011; 2011; 2012). Colorimetric measurements and image analysis were used to evaluate the intensity of the alga Klebsormidium flaccidum biofilm to evaluate the intrinsic properties of porosity, roughness, and surface pH on the susceptibility of mortars to biodegradation (Tran et al., 2012; Tran et al., 2014). Eyssautier-Chuine et al. (2016) and Carbone et al. (2017) combined the analysis of color changes in the carrier surface (stone and cotton duct, respectively) and the algal photosynthetic activity using PAM fluorometry. Both studies concluded that both techniques are complementary in the analysis of microalgae biofilm formation.
Another study that combined and correlated different non-destructive imaging techniques analyzed the early colonization stages of Chlorella biofilms by coupling imaging analysis techniques and mathematical methods (Moreno Osorio et al., 2020). Different parameters were measured correlating imaging techniques: the biomass growth and health condition were measured using PAM fluorometry; carrier colonization was studied by imaging analysis (Weka Segmentation) and through CIELAB colorimetry the green tones variations in the biofilm were measured. Biofilm vertical distribution and structural features were estimated by multichannel CLSM, and some parameters were aligned to the Gompertz model (Moreno Osorio et al., 2020). However, studies of this type are not common (Wagner and Horn, 2017; Fanesi et al., 2019), the combination of different techniques in bacterial biofilms is an extensive research field (Yang et al., 2013; Decelle et al., 2020) as has been shown along this section.
Many microalgae, bacteria, archaea, fungi, and protozoa cells have a natural tendency to attach to surfaces and grow on them. This absorption property has been used extensively in the development of biofilm bioreactors and the factors that stimulate and enhance it are still an open research topic.
During the last decade, a lot has been learned about the spatial distribution of different species and the species diversity in biofilms. The challenge for the future is to underline physiological properties, understanding the patterns in gene expression and the interaction between cells in biofilms. The fast development of molecular tools (i.e., metagenomics) has opened a new field to study in detail the physiological activity and status of individual cells in a spatial order. Among all promising beneficial applications of microalga biofilms, the production of algal nanoparticles is one of the most innovate and more research on production of recombinant proteins from algae is needed not only to identify the compounds responsible but also to better understand the mechanism of nanoparticle formation by microalgae and interactions in biofilms, what will lead to further exploration in nanocomposites and biosensing applications.
A variety of materials has been used as the supports for microalgal biofilms. Stainless steel, nylon, and natural fibers (porous fabrics) were found to be highly effective support materials for microalgal biofilms. The integration of algal bioreactors and wastewater treatment has been limited mainly to municipal wastewater, while a few agricultural and industrial wastewaters have been used for algal bioreactors due to inhibition of algal growth with high nutrient or toxic compounds concentrations or lack of essential nutrients. Overall, microalgal biofilm reactors integrated with wastewater treatment would have significant potential for high productivity of algal biomass and efficient wastewater treatment if various conditions and factors are optimized.
Microalgal suspended cultures are currently limited by their high operating costs associated with substrate/nutrients acquisition, high energy-consuming harvest of algal cells and low productivity of algal biomass. Various types of microalgal biofilm reactors integrated with wastewater treatment were developed to overcome current limitations of algal biofuel production. To date, different configurations, designs and geometries of biofilm reactors have been studied to produce algal biomass especially for biofuel production, and secondary valuable products, in the same time removing nutrients from wastewater. Intermittently submerged and perfused biofilm reactors are promising options among various types of biofilm to provide efficient utilization of nutrients in wastewater and high biomass productivity. However, the development of algal biofilm systems is still immature compared to suspension-based systems. Identifying appropriate strains and carrier materials for optimal biofilm formation and growth are still focus of interest in future research on algal biofilm systems.
There is a substantial need for novel measurement techniques that enable non-destructive, spatially resolved and real-time observation of biofilm formation and structures in membrane systems. CRM1, CRM2 and OCT can be beneficial to improve the understanding of structure/function relationships as these tools enable detailed visualization at the mesoscale and cell level. However, for a comprehensive and fundamental understanding of biofilm development and fate of molecules in the biofilm, a combination of different methods that conserve the biofilm structure is required.
JM: conceptualization, investigation, writing—original draft preparation. AP, LF, PL, and GE: writing—review and editing. All authors have read and agreed to the published version of the manuscript.
This research was supported through the Erasmus Mundus Joint Doctorate entitled “Environmental Technologies for Contaminated Solids, Soils, and Sediments” (ETeCoS3) (FPA n. 2010-0009).
The authors declare that the research was conducted in the absence of any commercial or financial relationships that could be construed as a potential conflict of interest.
All claims expressed in this article are solely those of the authors and do not necessarily represent those of their affiliated organizations, or those of the publisher, the editors and the reviewers. Any product that may be evaluated in this article, or claim that may be made by its manufacturer, is not guaranteed or endorsed by the publisher.
The Supplementary Material for this article can be found online at: https://www.frontiersin.org/articles/10.3389/fceng.2021.737710/full#supplementary-material
Abdel-Hameed, M. S., and Hammouda, O. (2007). Biotechnological Potential Uses of Immobilized Algae. Int. J. Agri. Biol. 9 (1), 183–192.
Abdel-Raouf, N., Al-Homaidan, A. A., and Ibraheem, I. B. M. (2012). Microalgae and Wastewater Treatment. Saudi J. Biol. Sci. 19 (3), 257–275. doi:10.1016/j.sjbs.2012.04.005
Acién, F. G., Fernández, J. M., Magán, J. J., and Molina, E. (2012). Production Cost of a Real Microalgae Production Plant and Strategies to Reduce it. Biotechnol. Adv. 30 (6), 1344–1353. doi:10.1016/j.biotechadv.2012.02.005
Adey, W. H., Kangas, P. C., and Mulbry, W. (2011). Algal Turf Scrubbing: Cleaning Surface Waters with Solar Energy while Producing a Biofuel. BioScience 61 (6), 434–441. doi:10.1525/bio.2011.61.6.5
Adey, W., Luckett, C., and Jensen, K. (1993). Phosphorus Removal from Natural Waters Using Controlled Algal Production. Restor Ecol. 1, 29–39. doi:10.1111/j.1526-100x.1993.tb00006.x
Agarwal, P., Gupta, R., and Agarwal, N. (2019). Advances in Synthesis and Applications of Microalgal Nanoparticles for Wastewater Treatment. J. Nanotechnology 2019, 1–9. doi:10.1155/2019/7392713
Akhtar, N., Iqbal, J., and Iqbal, M. (2003). Microalgal-luffa Sponge Immobilized Disc: a New Efficient Biosorbent for the Removal of Ni(II) from Aqueous Solution. Lett. Appl. Microbiol. 37, 149–153. doi:10.1046/j.1472-765x.2003.01366.x
Akhtar, N., Iqbal, M., Zafar, S. I., and Iqbal, J. (2008). Biosorption Characteristics of Unicellular green Alga Chlorella Sorokiniana Immobilized in Loofa Sponge for Removal of Cr(III). J. Environ. Sci. 20, 231–239. doi:10.1016/s1001-0742(08)60036-4
Alam, M. A., Vandamme, D., Chun, W., Zhao, X., Foubert, I., Wang, Z., et al. (2016). Bioflocculation as an Innovative Harvesting Strategy for Microalgae. Rev. Environ. Sci. Biotechnol. 15, 1–11. doi:10.1007/s11157-016-9408-8
Allison, D. G., Ruiz, B. a., SanJose, C., Jaspe, A., and Gilbert, P. (1998). Extracellular Products as Mediators of the Formation and Detachment ofPseudomonas Fluorescensbiofilms. FEMS Microb. Lett 167, 179–184. doi:10.1111/j.1574-6968.1998.tb13225.x
Arce, F. T., Avci, R., Beech, I. B., Cooksey, K. E., and Wigglesworth-Cooksey, B. (2004). A Live Bioprobe for Studying Diatom-Surface Interactions. Biophysical J. 87 (6), 4284–4297. doi:10.1529/biophysj.104.043307
Azeredo, J., Azevedo, N. F., Briandet, R., Cerca, N., Coenye, T., Costa, A. R., et al. (2017). Critical Review on Biofilm Methods. Crit. Rev. Microbiol. 43 (3), 313–351. doi:10.1080/1040841X.2016.1208146
Bai, X., Lant, P., and Pratt, S. (2015). The Contribution of Bacteria to Algal Growth by Carbon Cycling. Biotechnol. Bioeng. 112, 688–695. doi:10.1002/bit.25475
Barberousse, H., Brayner, R., Do Rego, A. M. B., Castaing, J.-C., Beurdeley-Saudou, P., and Colombet, J.-F. (2007). Adhesion of Façade Coating Colonisers, as Mediated by Physico-Chemical Properties. Biofouling 23, 15–24. doi:10.1080/08927010601093026
Barranguet, C., and Kromkamp, J. (2000). Estimating Primary Production Rates from Photosynthetic Electron Transport in Estuarine Microphytobenthos. Mar. Ecol. Prog. Ser. 204 (204), 39–52. doi:10.3354/meps204039
Barranguet, C., van Beusekom, S., Veuger, B., Neu, T., Manders, E., Sinke, J., et al. (2004). Studying Undisturbed Autotrophic Biofilms: Still a Technical challenge. Aquat. Microb. Ecol. 34, 1–9. doi:10.3354/ame034001
Barranguet, C., Veuger, B., Van Beusekom, S. A. M., Marvan, P., Sinke, J. J., and Admiraal, W. (2005). Divergent Composition of Algal-Bacterial Biofilms Developing under Various External Factors. Eur. J. Phycology 40 (1), 1–8. doi:10.1080/09670260400009882
Barros, A. C., Gonçalves, A. L., and Simões, M. (2018). Microalgal/cyanobacterial Biofilm Formation on Selected Surfaces: the Effects of Surface Physicochemical Properties and Culture media Composition. J. Appl. Phycol 31, 375–387. doi:10.1007/s10811-018-1582-3
Barros, A. I., Gonçalves, A. L., Simões, M., and Pires, J. C. M. (2015). Harvesting Techniques Applied to Microalgae: A Review. Renew. Sust. Energ. Rev. 41, 1489–1500. doi:10.1016/j.rser.2014.09.037
Becker, K. (1996). Exopolysaccharide Production and Attachment Strength of Bacteria and Diatoms on Substrates with Different Surface Tensions. Microb. Ecol. 32, 23–33. doi:10.1007/BF00170104
Becker, P., Hufnagle, W., Peters, G., and Herrmann, M. (2001). Detection of Differential Gene Expression in Biofilm-Forming versus Planktonic Populations of Staphylococcus aureus Using Micro-representational-difference Analysis. Appl. Environ. Microbiol. 67 (7), 2958–2965. doi:10.1128/AEM.67.7.2958-2965.2001
Benettoni, P., Stryhanyuk, H., Wagner, S., Kollmer, F., Moreno Osorio, J. H., Schmidt, M., et al. (2019). Identification of Nanoparticles and Their Localization in Algal Biofilm by 3D-Imaging Secondary Ion Mass Spectrometry. J. Anal. Spectrom. 34 (6), 1098–1108. doi:10.1039/C8JA00439K
Berner, F., Heimann, K., and Sheehan, M. (2015). Microalgal Biofilms for Biomass Production. J. Appl. Phycol 27 (5), 1793–1804. doi:10.1007/s10811-014-0489-x
Bernstein, H. C., Kesaano, M., Moll, K., Smith, T., Gerlach, R., Carlson, R. P., et al. (2014). Direct Measurement and Characterization of Active Photosynthesis Zones inside Wastewater Remediating and Biofuel Producing Microalgal Biofilms. Bioresour. Tech. 156, 206–215. doi:10.1016/j.biortech.2014.01.001
Besemer, K., Singer, G., Limberger, R., Chlup, A.-K., Hochedlinger, G., Hodl, I., et al. (2007). Biophysical Controls on Community Succession in Stream Biofilms. Appl. Environ. Microbiol. 73, 4966–4974. doi:10.1128/aem.00588-07
Beyenal, H., and Lewandowski, Z. (2000). Combined Effect of Substrate Concentration and Flow Velocity on Effective Diffusivity in Biofilms. Wat. Res. 34 (2), 528–538. doi:10.1016/s0043-1354(99)00147-5
Bigelow, N. W., Hardin, W. R., Barker, J. P., Ryken, S. A., Macrae, A. C., and Cattolico, R. A. (2011). A Comprehensive GC-MS Sub‐Microscale Assay for Fatty Acids and its Applications. J. Am. Oil Chem. Soc. 88 (9), 1329–1338. doi:10.1007/s11746-011-1799-7
Blanken, W., Janssen, M., Cuaresma, M., Libor, Z., Bhaiji, T., and Wijffels, R. H. (2014). Biofilm Growth ofChlorella Sorokinianain a Rotating Biological Contactor Based Photobioreactor. Biotechnol. Bioeng. 111 (12), 2436–2445. doi:10.1002/bit.25301
Boelee, N. C., Janssen, M., Temmink, H., Shrestha, R., Buisman, C. J. N., and Wijffels, R. H. (2014). Nutrient Removal and Biomass Production in an Outdoor Pilot-Scale Phototrophic Biofilm Reactor for Effluent Polishing. Appl. Biochem. Biotechnol. 172, 405–422. doi:10.1007/s12010-013-0478-6
Boelee, N. C., Temmink, H., Janssen, M., Buisman, C. J. N., and Wijffels, R. H. (2011). Nitrogen and Phosphorus Removal from Municipal Wastewater Effluent Using Microalgal Biofilms. Water Res. 45 (18), 5925–5933. doi:10.1016/j.watres.2011.08.044
Boles, B. R., and Singh, P. K. (2008). Endogenous Oxidative Stress Produces Diversity and Adaptability in Biofilm Communities. Proc. Natl. Acad. Sci. 105, 12503–12508. doi:10.1073/pnas.0801499105
Borowitzka, M. A. (1999). Commercial Production of Microalgae: Ponds, Tanks, Tubes and Fermenters. J. Biotechnol. 70, 313–321. doi:10.1016/s0168-1656(99)00083-8
Brayner, R., Couté, A., Livage, J., Perrette, C., and Sicard, C. (2011). Micro-algal Biosensors. Anal. Bioanal. Chem. 401 (2), 581–597. doi:10.1007/s00216-011-5107-z
Buckingham-Meyer, K., Goeres, D. M., and Hamilton, M. A. (2007). Comparative Evaluation of Biofilm Disinfectant Efficacy Tests. J. Microbiol. Methods 70 (2), 236–244. doi:10.1016/j.mimet.2007.04.010
Buhmann, M., Kroth, P. G., and Schleheck, D. (2012). Photoautotrophic-heterotrophic Biofilm Communities: a Laboratory Incubator Designed for Growing Axenic Diatoms and Bacteria in Defined Mixed-Species Biofilms. Environ. Microbiol. Rep. 4 (1), 133–140. doi:10.1111/j.1758-2229.2011.00315.x
Bullitt, E., and Makowski, L. (1995). Structural Polymorphism of Bacterial Adhesion Pili. Nature 373 (6510), 164–167. doi:10.1038/373164a0
Bumbak, F., Cook, S., Zachleder, V., Hauser, S., and Kovar, K. (2011). Best Practices in Heterotrophic High-Cell-Density Microalgal Processes: Achievements, Potential and Possible Limitations. Appl. Microbiol. Biotechnol. 91 (1), 31–46. doi:10.1007/s00253-011-3311-6
Burns, A., and Ryder, D. S. (2001). Potential for Biofilms as Biological Indicators in Australian Riverine Systems. Ecol. Manage. Restor. 2 (1). doi:10.1046/j.1442-8903.2001.00069.x
Callow, M. E. (2000). “Algal Biofilms,” in Biofilms: Recent Advances in Their Study and Control. Editor L. V. Evans (Amsterdam: Harwood Academic Publishers), 189–204. doi:10.1201/9781482284157-18
Cao, J., Yuan, W., Pei, Z. J., Davis, T., Cui, Y., and Beltran, M. (2009). A Preliminary Study of the Effect of Surface Texture on Algae Cell Attachment for a Mechanical-Biological Energy Manufacturing System. J. Manufacturing Sci. Eng. 131 (6). doi:10.1115/1.4000562
Carbone, D. A., Gargano, I., Pinto, G., De Natale, A., and Pollio, A. (2017). Evaluating Microalgal Attachment to Surfaces: a First Approach towards a Laboratory Integrated Assessment. Chem. Eng. Trans. 57, 73–78. doi:10.3303/CET1757013
Castro-Ceseña, A. B., del Pilar Sánchez-Saavedra, M., and Ruíz-Güereca, D. A. (2015). del Pilar Sánchez-Saavedra, M., and Ruíz-Güereca, D.A. (Optimization of entrapment efficiency and evaluation of nutrient removal (N and P) of Synechococcus elongatus in novel core-shell capsules. J. Appl. Phycol 28 (4), 2343–2351. doi:10.1007/s10811-015-0771-6
Celmer, D., Oleszkiewicz, J. A., and Cicek, N. (2008). Impact of Shear Force on the Biofilm Structure and Performance of a Membrane Biofilm Reactor for Tertiary Hydrogen-Driven Denitrification of Municipal Wastewater. Water Res. 42 (12), 3057–3065. doi:10.1016/j.watres.2008.02.031
Chang, H. T., Rittmann, B. E., Amar, D., Heim, R., Ehlinger, O., and Lesty, Y. (1991). Biofilm Detachment Mechanisms in a Liquid-Fluidized Bed. Biotechnol. Bioeng. 38, 499–506. doi:10.1002/bit.260380508
Chen, C.-Y., Lu, I.-C., Nagarajan, D., Chang, C.-H., Ng, I.-S., Lee, D.-J., et al. (2018). A Highly Efficient Two-Stage Cultivation Strategy for Lutein Production Using Heterotrophic Culture of Chlorella Sorokiniana MB-1-M12. Bioresour. Tech. 253, 141–147. doi:10.1016/j.biortech.2018.01.027
Chen, M. Y., Lee, D. J., and Tay, J. H. (2007). Distribution of Extracellular Polymeric Substances in Aerobic Granules. Appl. Microbiol. Biotechnol. 73, 1463–1469. doi:10.1007/s00253-006-0617-x
Cheng, P., Ji, B., Gao, L., Zhang, W., Wang, J., and Liu, T. (2013). The Growth, Lipid and Hydrocarbon Production of Botryococcus Braunii with Attached Cultivation. Bioresour. Tech. 138, 95–100. doi:10.1016/j.biortech.2013.03.150
Cheng, P., Wang, Y., Liu, T., and Liu, D. (2017). Biofilm Attached Cultivation of Chlorella Pyrenoidosa Is a Developed System for Swine Wastewater Treatment and Lipid Production. Front. Plant Sci. 8, 1594. doi:10.3389/fpls.2017.01594
Christenson, L. B., and Sims, R. C. (2012). Rotating Algal Biofilm Reactor and Spool Harvester for Wastewater Treatment with Biofuels By-Products. Biotechnol. Bioeng. 109, 1674–1684. doi:10.1002/bit.24451
Christenson, L., and Sims, R. (2011). Production and Harvesting of Microalgae for Wastewater Treatment, Biofuels, and Bioproducts. Biotechnol. Adv. 29 (6), 686–702. doi:10.1016/j.biotechadv.2011.05.015
Chuah, S. Y., Dasan, Y. K., Cheng, Y. W., Lim, J. W., Ho, Y. C., Tan, I. S., et al. (2020). The Potential of Attached Growth of Microalgae on Solid Surface for Biomass and Lipid Production. IOP Conf. Ser. Mater. Sci. Eng. 965, 012001. doi:10.1088/1757-899x/965/1/012001
Cole, J. K., Hutchison, J. R., Renslow, R. S., Kim, Y.-M., Chrisler, W. B., Engelmann, H. E., et al. (2014). Phototrophic Biofilm Assembly in Microbial-Mat-Derived Unicyanobacterial Consortia: Model Systems for the Study of Autotroph-Heterotroph Interactions. Front. Microbiol. 5, 109. doi:10.3389/fmicb.2014.00109
Costerton, J. W., Lewandowski, Z., Caldwell, D. E., Korber, D. R., and Lappin-Scott, H. M. (1995). Microbial Biofilms. Annu. Rev. Microbiol. 49, 711–745. doi:10.1146/annurev.mi.49.100195.003431
Cui, Y., and Yuan, W. (2013). Thermodynamic Modeling of Algal Cell-Solid Substrate Interactions. Appl. Energ. 112, 485–492. doi:10.1016/j.apenergy.2013.03.036
Dahoumane, S. A., Mechouet, M., Mechouet, M., Alvarez, F. J., Agathos, S. N., and Jeffryes, C. (2016). Microalgae: An Outstanding Tool in Nanotechnology. Rb 1 (4). doi:10.21931/rb/2016.01.04.7
de Beer, D., van den Heuvel, J. C., and Ottengraf, S. P. P. (1993). Microelectrode Measurements of the Activity Distribution in Nitrifying Bacterial Aggregates. Appl. Environ. Microbiol. 59, 573–579. doi:10.1128/aem.59.2.573-579.1993
De Muynck, W., Ramirez, A. M., De Belie, N., and Verstraete, W. (2009). Evaluation of Strategies to Prevent Algal Fouling on white Architectural and Cellular concrete. Int. Biodeterioration Biodegradation 63 (6), 679–689. doi:10.1016/j.ibiod.2009.04.007
de-Bashan, L. E., and Bashan, Y. (2010). Immobilized Microalgae for Removing Pollutants: Review of Practical Aspects. Bioresour. Tech. 101 (6), 1611–1627. doi:10.1016/j.biortech.2009.09.043
de-Bashan, L. E., Mayali, X., Bebout, B. M., Weber, P. K., Detweiler, A. M., Hernandez, J. P., et al. (2016). Establishment of Stable Synthetic Mutualism without Co-evolution between Microalgae and Bacteria Demonstrated by Mutual Transfer of Metabolites (NanoSIMS Isotopic Imaging) and Persistent Physical Association (Fluorescent In Situ Hybridization). Algal Res. 15, 179–186. doi:10.1016/j.algal.2016.02.019
Dean, A. P., Sigee, D. C., Estrada, B., and Pittman, J. K. (2010). Using FTIR Spectroscopy for Rapid Determination of Lipid Accumulation in Response to Nitrogen Limitation in Freshwater Microalgae. Bioresour. Tech. 101 (12), 4499–4507. doi:10.1016/j.biortech.2010.01.065
Decelle, J., Veronesi, G., Gallet, B., Stryhanyuk, H., Benettoni, P., Schmidt, M., et al. (2020). Subcellular Chemical Imaging: New Avenues in Cell Biology. Trends Cel Biol. 30 (3), 173–188. doi:10.1016/j.tcb.2019.12.007
DeGraff, B., and Demas, J. (2005). Reviews in Fluorescence. NY Springer, 125–151.Luminescence-based Oxygen Sensors
Dixon, C., and Wilken, L. R. (2018). Green Microalgae Biomolecule Separations and Recovery. Bioresour. Bioproc. 5 (1). doi:10.1186/s40643-018-0199-3
Domozych, D. S. (2007). Exopolymer Production by the Green AlgaPenium Margaritaceum: Implications for Biofilm Residency. Int. J. Plant Sci. 168 (6), 763–774. doi:10.1086/513606
Efeoglu, E., and Culha, M. (2013). In Situ-monitoring of Biofilm Formation by Using Surface-Enhanced Raman Scattering. Appl. Spectrosc. 67 (5), 498–505. doi:10.1366/120689610.1366/12-06896
Eggert, A., Häubner, N., Klausch, S., Karsten, U., and Schumann, R. (2006). Quantification of Algal Biofilms Colonising Building Materials: Chlorophyllameasured by PAM-Fluorometry as a Biomass Parameter. Biofouling 22 (2), 79–90. doi:10.1080/08927010600579090
Eyssautier-Chuine, S., Vaillant-Gaveau, N., Gommeaux, M., Thomachot-Schneider, C., Pleck, J., and Fronteau, G. (2016). Chlorophyll Fluorescence and Colorimetric Analysis for Monitoring the Algal Development on Biocide-Treated Stone. Open Conf. Proceed. J. 7 (Suppl. 1M5), 55–64. doi:10.2174/2210289201607020055
Fanesi, A., Paule, A., Bernard, O., Briandet, R., and Lopes, F. (2019). The Architecture of Monospecific Microalgae Biofilms. Microorganisms 7 (9), 352. doi:10.3390/microorganisms7090352
Fernández-Silva, I., Sanmartín, P., Silva, B., Moldes, A., and Prieto, B. (2011). Quantification of Phototrophic Biomass on Rocks: Optimization of Chlorophyll-A Extraction by Response Surface Methodology. J. Ind. Microbiol. Biotechnol. 38 (1), 179–188. doi:10.1007/s10295-010-0843-1
Fica, Z. T., and Sims, R. C. (2016). Algae-based Biofilm Productivity Utilizing Dairy Wastewater: Effects of Temperature and Organic Carbon Concentration. J. Biol. Eng. 10, 18. doi:10.1186/s13036-016-0039-y
Finlay, J. A., Callow, M., Ista, L., Lopez, G., and Callow, J. (2002). The Influence of Surface Wettability on the Adhesion Strength of Settled Spores of the green Alga Enteromorpha and the Diatom Amphora. Integr. Comp. Biol. 42, 1116–1122. doi:10.1093/icb/42.6.1116
Fitch, M., and England, E. (2002). Biological Fixed Film Systems. Water Environ. Res. 74, 1–87. doi:10.2175/106143002x140440
Fletcher, R. L., and Callow, M. E. (1992). The Settlement, Attachment and Establishment of marine Algal Spores. Br. Phycological J. 27 (3), 303–329. doi:10.1080/00071619200650281
Gagliano, M. C., Ismail, S. B., Stams, A. J. M., Plugge, C. M., Temmink, H., and Van Lier, J. B. (2017). Biofilm Formation and Granule Properties in Anaerobic Digestion at High Salinity. Water Res. 121, 61–71. doi:10.1016/j.watres.2017.05.016
Galloway, T. S., Cole, M., and Lewis, C. (2017). Interactions of Microplastic Debris throughout the marine Ecosystem. Nat. Ecol. Evol. 1, 116. doi:10.1038/s41559-017-0116
Gao, F., Yang, Z.-H., Li, C., Zeng, G.-M., Ma, D.-H., and Zhou, L. (2015). A Novel Algal Biofilm Membrane Photobioreactor for Attached Microalgae Growth and Nutrients Removal from Secondary Effluent. Bioresour. Tech. 179, 8–12. doi:10.1016/j.biortech.2014.11.108
Garny, K., Horn, H., and Neu, T. R. (2008). Interaction between Biofilm Development, Structure and Detachment in Rotating Annular Reactors. Bioproc. Biosyst Eng 31 (6), 619–629. doi:10.1007/s00449-008-0212-x
Genin, S. N., Stewart Aitchison, J., and Grant Allen, D. (2014). Design of Algal Film Photobioreactors: Material Surface Energy Effects on Algal Film Productivity, Colonization and Lipid Content. Bioresour. Tech. 155, 136–143. doi:10.1016/j.biortech.2013.12.060
Genty, B., Briantais, J.-M., and Baker, N. R. (1989). The Relationship between the Quantum Yield of Photosynthetic Electron Transport and Quenching of Chlorophyll Fluorescence. Biochim. Biophys. Acta (Bba) - Gen. Subjects 990, 87–92. doi:10.1016/S0304-4165(89)80016-9
Genzer, J., and Efimenko, K. (2006). Recent Developments in Superhydrophobic Surfaces and Their Relevance to marine Fouling: a Review. Biofouling 22 (5-6), 339–360. doi:10.1080/08927010600980223
Golueke, C. G., Oswald, W. J., and Gotaas, H. B. (1957). Anaerobic Digestion of Algae. Appl. Microbiol. 5 (1), 47–55. doi:10.1128/am.5.1.47-55.195710.1128/aem.5.1.47-55.1957
González-Fernández, C., and Ballesteros, M. (2012). Microalgae Autoflocculation: an Alternative to High-Energy Consuming Harvesting Methods. J. Appl. Phycol 25 (4), 991–999. doi:10.1007/s10811-012-9957-3
González-Fernández, C., Sialve, B., Bernet, N., and Steyer, J. P. (2012). Comparison of Ultrasound and thermal Pretreatment of Scenedesmus Biomass on Methane Production. Bioresour. Tech. 110, 610–616. doi:10.1016/j.biortech.2012.01.043
Goodwin, T. W., and Jamikorn, M. (1954). Studies in Carotenogenesis. 11. Carotenoid Synthesis in the Alga Haematococcus pluvialis. Biochem. J. 57 (3), 376–381. doi:10.1042/bj0570376
Gross, M., Henry, W., Michael, C., and Wen, Z. (2013). Development of a Rotating Algal Biofilm Growth System for Attached Microalgae Growth with In Situ Biomass Harvest. Bioresour. Tech. 150, 195–201. doi:10.1016/j.biortech.2013.10.016
Gross, M., Jarboe, D., and Wen, Z. (2015). Biofilm-based Algal Cultivation Systems. Appl. Microbiol. Biotechnol. 99 (14), 5781–5789. doi:10.1007/s00253-015-6736-5
Gross, M., Zhao, X., Mascarenhas, V., and Wen, Z. (2016). Effects of the Surface Physico-Chemical Properties and the Surface Textures on the Initial Colonization and the Attached Growth in Algal Biofilm. Biotechnol. Biofuels 9, 38. doi:10.1186/s13068-016-0451-z
Gupta, P. L., Choi, H. J., and Lee, S.-M. (2016). Enhanced Nutrient Removal from Municipal Wastewater Assisted by Mixotrophic Microalgal Cultivation Using Glycerol. Environ. Sci. Pollut. Res. 23 (10), 10114–10123. doi:10.1007/s11356-016-6224-1
Guttenplan, S. B., and Kearns, D. B. (2013). Regulation of Flagellar Motility during Biofilm Formation. FEMS Microbiol. Rev. 37 (6), 849–871. doi:10.1111/1574-6976.12018
Guzzon, A., Bohn, A., Diociaiuti, M., and Albertano, P. (2008). Cultured Phototrophic Biofilms for Phosphorus Removal in Wastewater Treatment. Water Res. 42 (16), 4357–4367. doi:10.1016/j.watres.2008.07.029
Hallmann, C., Stannek, L., Fritzlar, D., Hause-Reitner, D., Friedl, T., and Hoppert, M. (2013). Molecular Diversity of Phototrophic Biofilms on Building Stone. FEMS Microbiol. Ecol. 84 (2), 355–372. doi:10.1111/1574-6941.12065
Hamano, H., Nakamura, S., Hayakawa, J., Miyashita, H., and Harayama, S. (2017). Biofilm-based Photobioreactor Absorbing Water and Nutrients by Capillary Action. Bioresour. Tech. 223, 307–311. doi:10.1016/j.biortech.2016.10.088
Han, T., Lu, H. F., Ma, S. S., Zhang, Y. H., and Duan, N. (2017). Progress in Microalgae Cultivation Photobioreactors and Applications in Wastewater Treatment: A Review. Int. J. Agric. Biol. Eng. 10 (1), 1–29. doi:10.3965/j.ijabe.20171001.2705
Han, W., Clarke, W., and Pratt, S. (2016). Cycling of Iodine by Microalgae: Iodine Uptake and Release by a Microalgae Biofilm in a Groundwater Holding Pond. Ecol. Eng. 94, 286–294. doi:10.1016/j.ecoleng.2016.05.001
Hassard, F., Biddle, J., Cartmell, E., Jefferson, B., Tyrrel, S., and Stephenson, T. (2015). Rotating Biological Contactors for Wastewater Treatment - A Review. Process Saf. Environ. Prot. 94, 285–306. doi:10.1016/j.psep.2014.07.003
Häubner, N., Schumann, R., and Karsten, U. (2006). Aeroterrestrial Microalgae Growing in Biofilms on Facades-Response to Temperature and Water Stress. Microb. Ecol. 51 (3), 285–293. doi:10.1007/s00248-006-9016-1
He, H., Zhou, M., Yang, J., Hu, Y., and Zhao, Y. (2014). Simultaneous Wastewater Treatment, Electricity Generation and Biomass Production by an Immobilized Photosynthetic Algal Microbial Fuel Cell. Bioproc. Biosyst Eng 37 (5), 873–880. doi:10.1007/s00449-013-1058-4
He, S., and Xue, G. (2010). Algal-based Immobilization Process to Treat the Effluent from a Secondary Wastewater Treatment Plant (WWTP). J. Hazard. Mater. 178 (1-3), 895–899. doi:10.1016/j.jhazmat.2010.02.022
Hille, A., He, M., Ochmann, C., Neu, T. R., and Horn, H. (2009). Application of Two Component Biodegradable Carriers in a Particle-Fixed Biofilm Airlift Suspension Reactor: Development and Structure of Biofilms. Bioproc. Biosyst Eng 32 (1), 31–39. doi:10.1007/s00449-008-0217-5
Hoffmann, J. P. (1998). Wastewater Treatment with Suspended and Nonsuspended Algae. J. Phycol. 34, 757–763. doi:10.1046/j.1529-8817.1998.340757.x
Hoh, D., Watson, S., and Kan, E. (2016). Algal Biofilm Reactors for Integrated Wastewater Treatment and Biofuel Production: A Review. Chem. Eng. J. 287, 466–473. doi:10.1016/j.cej.2015.11.062
Hook, A. L., Chang, C.-Y., Yang, J., Luckett, J., Cockayne, A., Atkinson, S., et al. (2012). Combinatorial Discovery of Polymers Resistant to Bacterial Attachment. Nat. Biotechnol. 30, 868–875. doi:10.1038/nbt.2316
Hori, K., and Matsumoto, S. (2010). Bacterial Adhesion: From Mechanism to Control. Biochem. Eng. J. 48, 424–434. doi:10.1016/j.bej.2009.11.014
Hu, Q., Sommerfeld, M., Jarvis, E., Ghirardi, M., Posewitz, M., Seibert, M., et al. (2008). Microalgal Triacylglycerols as Feedstocks for Biofuel Production: Perspectives and Advances. Plant J. 54 (4), 621–639. doi:10.1111/j.1365-313X.2008.03492.x
Huang, Y., Xiong, W., Liao, Q., Fu, Q., Xia, A., Zhu, X., et al. (2016). Comparison of Chlorella Vulgaris Biomass Productivity Cultivated in Biofilm and Suspension from the Aspect of Light Transmission and Microalgae Affinity to Carbon Dioxide. Bioresour. Tech. 222, 367–373. doi:10.1016/j.biortech.2016.09.099
Huang, Y., Zheng, Y., Li, J., Liao, Q., Fu, Q., Xia, A., et al. (2018). Enhancing Microalgae Biofilm Formation and Growth by Fabricating Microgrooves onto the Substrate Surface. Bioresour. Tech. 261, 36–43. doi:10.1016/j.biortech.2018.03.139
Hultberg, M., Asp, H., Marttila, S., Bergstrand, K.-J., and Gustafsson, S. (2014). Biofilm Formation by Chlorella Vulgaris Is Affected by Light Quality. Curr. Microbiol. 69 (5), 699–702. doi:10.1007/s00284-014-0645-1
Hunt, S. M., Werner, E. M., Huang, B., Hamilton, M. A., and Stewart, P. S. (2004). Hypothesis for the Role of Nutrient Starvation in Biofilm Detachment. Appl. Environ. Microbiol. 70 (12), 7418–7425. doi:10.1128/aem.70.12.7418-7425.2004
Irving, T. E., and Allen, D. G. (2011). Species and Material Considerations in the Formation and Development of Microalgal Biofilms. Appl. Microbiol. Biotechnol. 92 (2), 283–294. doi:10.1007/s00253-011-3341-0
Johnson, M. B., and Wen, Z. (2010). Development of an Attached Microalgal Growth System for Biofuel Production. Appl. Microbiol. Biotechnol. 85 (3), 525–534. doi:10.1007/s00253-009-2133-2
Jones, P. R., Cottrell, M. T., Kirchman, D. L., and Dexter, S. C. (2007). Bacterial Community Structure of Biofilms on Artificial Surfaces in an Estuary. Microb. Ecol. 53, 153–162. doi:10.1007/s00248-006-9154-5
Kaplan, J. B., Ragunath, C., Fine, D. H., and Ramasubbu, N. (2004). Enzymatic Detachment of Staphylococcus Epidermidis Biofilms. Antimicrob. Agents Chemother. 48 (7), 2633–2636. doi:10.1128/aac.48.7.2633-2636.2004
Kardel, K., Blersch, D. M., and Carrano, A. L. (2018). Custom Design of Substratum Topography Increases Biomass Yield in Algal Turf Scrubbers. Environ. Eng. Sci. 35, 856–863. doi:10.1089/ees.2017.0354
Katarzyna, L., Sai, G., and Singh, O. A. (2015). Non-enclosure Methods for Non-suspended Microalgae Cultivation: Literature Review and Research Needs. Renew. Sust. Energ. Rev. 42, 1418–1427. doi:10.1016/j.rser.2014.11.029
Keleştemur, S., Avci, E., and Çulha, M. (2018). Raman and Surface-Enhanced Raman Scattering for Biofilm Characterization. Chemosensors 6 (1), 5. doi:10.3390/chemosensors6010005
Kesaano, M., and Sims, R. C. (2014). Algal Biofilm Based Technology for Wastewater Treatment. Algal Res. 5, 231–240. doi:10.1016/j.algal.2014.02.003
Keyhani, N. O., and Roseman, S. (1996). The Chitin Catabolic cascade in the marine Bacterium Vibrio Furnissii. J. Biol. Chem. 271, 33414–33424. doi:10.1074/jbc.271.52.33414
Khosravi, Y., Kandukuri, R. D. P., Palmer, S. R., Gloag, E. S., Borisov, S. M., Starke, E. M., et al. (2020). Use of an Oxygen Planar Optode to Assess the Effect of High Velocity Microsprays on Oxygen Penetration in a Human Dental Biofilms In-Vitro. BMC Oral Health 20 (1), 230. doi:10.1186/s12903-020-01217-0
Köhler, J., Hansen, P., and Wahl, M. (1999). Colonization Patterns at the Substratum‐water Interface: How Does Surface Microtopography Influence Recruitment Patterns of Sessile Organisms?. Biofouling 14, 237–248. doi:10.1080/08927019909378415
Kokare, C., Chakraborty, S., Khopade, A., and Mahadik, K. (2009). Biofilm: Importance and Applications. Indian J. Biotechnol. 8, 159–168.
Kolber, Z. S., Prášil, O., and Falkowski, P. G. (1998). Measurements of Variable Chlorophyll Fluorescence Using Fast Repetition Rate Techniques: Defining Methodology and Experimental Protocols. Biochim. Biophys. Acta (Bba) - Bioenerg. 1367, 88–106. doi:10.1016/s0005-2728(98)00135-2
Krasowska, A., and Sigler, K. (2014). How Microorganisms Use Hydrophobicity and what Does This Mean for Human Needs?. Front. Cel. Infect. Microbiol. 4, 112. doi:10.3389/fcimb.2014.00112
Kuhl, M., Rickelt, L. F., and Thar, R. (2007). Combined Imaging of Bacteria and Oxygen in Biofilms. Appl. Environ. Microbiol. 73 (19), 6289–6295. doi:10.1128/AEM.01574-07
Lahin, F. A., Sarbatly, R., and Suali, E. (2016). Polishing of POME by Chlorella Sp. In Suspended and Immobilized System. IOP Conf. Ser. Earth Environ. Sci. 36, 012030. doi:10.1088/1755-1315/36/1/012030
Lam, M. K., and Lee, K. T. (2012). Immobilization as a Feasible Method to Simplify the Separation of Microalgae from Water for Biodiesel Production. Chem. Eng. J. 191, 263–268. doi:10.1016/j.cej.2012.03.013
Lasaro, M. A., Salinger, N., Zhang, J., Wang, Y., Zhong, Z., Goulian, M., et al. (2009). F1C Fimbriae Play an Important Role in Biofilm Formation and Intestinal Colonization by the Escherichia coli Commensal Strain Nissle 1917. Appl. Environ. Microbiol. 75 (1), 246–251. doi:10.1128/AEM.01144-08
Latour, R. A. (2004). "Biomaterials: Protein-Surface interactions.‖ In: ," in Encyclopedia of Biomaterials and Biomedical Engineering eds. G. L. Bowlin (New York Marcel Dekker)..
Lee, S.-H., Oh, H.-M., Jo, B.-H., Lee, S.-A., Shin, S.-Y., Kim, H.-S., et al. (2014). Higher Biomass Productivity of Microalgae in an Attached Growth System, Using Wastewater. J. Microbiol. Biotechnol. 24 (11), 1566–1573. doi:10.4014/jmb.1406.06057
LeTourneau, M. K., Marshall, M. J., Cliff, J. B., Bonsall, R. F., Dohnalkova, A. C., Mavrodi, D. V., et al. (2018). Phenazine‐1‐carboxylic Acid and Soil Moisture Influence Biofilm Development and Turnover of Rhizobacterial Biomass on Wheat Root Surfaces. Environ. Microbiol. 20(6, 2178, 2194). doi:10.1111/1462-2920.14244
Levasseur, W., Perré, P., and Pozzobon, V. (2020). A Review of High Value-Added Molecules Production by Microalgae in Light of the Classification. Biotechnol. Adv. 41, 107545. doi:10.1016/j.biotechadv.2020.107545
Lewandowski, Z., and Beyenal, H. (2003). “Mass Transport in Heterogeneous Biofilms,” in Biofilms in Wastewater Treatment. An Interdisciplinary Approach. Editors (London: IWA Publising), 147–177.
Lewandowski, Z., and Boltz, J. P. (2011). Biofilms in Water and Wastewater Treatment. Treatise Water Sci., 529–570. doi:10.1016/b978-0-444-53199-5.00095-6
Liao, C., Liang, X., Soupir, M. L., and Jarboe, L. R. (2015). Cellular, Particle and Environmental Parameters Influencing Attachment in Surface Waters: a Review. J. Appl. Microbiol. 119 (2), 315–330. doi:10.1111/jam.12860
Limoli, D. H., Jones, C. J., and Wozniak, D. J. (2015). Bacterial Extracellular Polysaccharides in Biofilm Formation and Function. Microbiol. Spectr. 3 (3). doi:10.1128/microbiolspec.MB-0011-2014
Liu, T., Wang, J., Hu, Q., Cheng, P., Ji, B., Liu, J., et al. (2013). Attached Cultivation Technology of Microalgae for Efficient Biomass Feedstock Production. Bioresour. Tech. 127, 216–222. doi:10.1016/j.biortech.2012.09.100
Ljaljevic-Grbic, M., Vukojevic, J., Subakov-Simic, G., Krizmanic, J., and Stupar, M. (2010). Biofilm Forming Cyanobacteria, Algae and Fungi on Two Historic Monuments in Belgrade, Serbia. Arch. Biol. Sci. Belgra 62 (3), 625–631. doi:10.2298/abs1003625l
Lobelle, D., and Cunliffe, M. (2011). Early Microbial Biofilm Formation on marine Plastic Debris. Mar. Pollut. Bull. 62, 197–200. doi:10.1016/j.marpolbul.2010.10.013
Lorite, G. S., Rodrigues, C. M., de Souza, A. A., Kranz, C., Mizaikoff, B., and Cotta, M. A. (2011). The Role of Conditioning Film Formation and Surface Chemical Changes on Xylella Fastidiosa Adhesion and Biofilm Evolution. J. Colloid Interf. Sci. 359, 289–295. doi:10.1016/j.jcis.2011.03.066
MacDougall, K. M., McNichol, J., McGinn, P. J., O’Leary, S. J. B., and Melanson, J. E. (2011). Triacylglycerol Profiling of Microalgae Strains for Biofuel Feedstock by Liquid Chromatography-High-Resolution Mass Spectrometry. Anal. Bioanal. Chem. 401 (8), 2609–2616. doi:10.1007/s00216-011-5376-6
Malapascua, J., Jerez, C., Sergejevová, M., Figueroa, F., and Masojídek, J. (2014). Photosynthesis Monitoring to Optimize Growth of Microalgal Mass Cultures: Application of Chlorophyll Fluorescence Techniques. Aquat. Biol. 22, 123–140. doi:10.3354/ab00597
Mallick, N. (2002). Biotechnological Potential of Immobilized Algae for Wastewater N, P and Metal Removal: A Review. BioMetals 15, 377–390. doi:10.1023/a:1020238520948
Manso, S., De Muynck, W., Segura, I., Aguado, A., Steppe, K., Boon, N., et al. (2014). Bioreceptivity Evaluation of Cementitious Materials Designed to Stimulate Biological Growth. Sci. Total Environ. 481, 232–241. doi:10.1016/j.scitotenv.2014.02.059
Mantzorou, A., and Ververidis, F. (2019). Microalgal Biofilms: A Further Step over Current Microalgal Cultivation Techniques. Sci. Total Environ. 651, 3187–3201. doi:10.1016/j.scitotenv.2018.09.355
Mata, T. M., Martins, A. A., and Caetano, N. S. (2010). Microalgae for Biodiesel Production and Other Applications: A Review. Renew. Sust. Energ. Rev. 14 (1), 217–232. doi:10.1016/j.rser.2009.07.020
McLean, J. S., Ona, O. N., and Majors, P. D. (2008). Correlated Biofilm Imaging, Transport and Metabolism Measurements via Combined Nuclear Magnetic Resonance and Confocal Microscopy. Isme J. 2 (2), 121–131. doi:10.1038/ismej.2007.107
Melo, L. F. (2005). Biofilm Physical Structure, Internal Diffusivity and Tortuosity. Water Sci. Tech. 52, 77–84. doi:10.2166/wst.2005.0184
Mohammad Mirzaie, M. A., Kalbasi, M., Mousavi, S. M., and Ghobadian, B. (2016). Investigation of Mixotrophic, Heterotrophic, and Autotrophic Growth of Chlorella Vulgaris under Agricultural Waste Medium. Prep. Biochem. Biotechnol. 46 (2), 150–156. doi:10.1080/10826068.2014.995812
Mohsenpour, S. F., Hennige, S., Willoughby, N., Adeloye, A., and Gutierrez, T. (2021). Integrating Micro-algae into Wastewater Treatment: A Review. Sci. Total Environ. 752, 142168. doi:10.1016/j.scitotenv.2020.142168
Moreno Osorio, J. H., De Natale, A., Del Mondo, A., Frunzo, L., Lens, P. N. L., Esposito, G., et al. (2020). Early Colonization Stages of Fabric Carriers by Two Chlorella Strains. J. Appl. Phycol 32 (6), 3631–3644. doi:10.1007/s10811-020-02244-8
Moreno Osorio, J. H., Luongo, V., Del Mondo, A., Pinto, G., Frunzo, L., Pollio, A., et al. (2018). Nutrient Removal from High Strength Nitrate Containing Industrial Wastewater Using Chlorella Sp. Strain ACUF_802. Ann. Microbiol. 68 (12), 899–913. doi:10.1007/s13213-018-1400-9
Moreno Osorio, J. H., Pinto, G., Pollio, A., Frunzo, L., Lens, P. N. L., and Esposito, G. (2019b). Start-up of a Nutrient Removal System Using Scenedesmus Vacuolatus and Chlorella Vulgaris Biofilms. Bioresour. Bioproc. 6. doi:10.1186/s40643-019-0259-3
Moreno-Garrido, I. (2008). Microalgae Immobilization: Current Techniques and Uses. Bioresour. Tech. 99 (10), 3949–3964. doi:10.1016/j.biortech.2007.05.040
Moudříková, Š., Sadowsky, A., Metzger, S., Nedbal, L., Mettler-Altmann, T., and Mojzeš, P. (2017). Quantification of Polyphosphate in Microalgae by Raman Microscopy and by a Reference Enzymatic Assay. Anal. Chem. 89 (22), 12006–12013. doi:10.1021/acs.analchem.7b02393
Mueller, L., de Brouwer, J., Almeida, J., Stal, L., and Xavier, J. (2006). Analysis of a marine Phototrophic Biofilm by Confocal Laser Scanning Microscopy Using the New Image Quantification Software PHLIP. BMC Ecol. 6, 1. doi:10.1186/1472-6785-6-1
Mukherjee, C., Chowdhury, R., and Ray, K. (2015). Phosphorus Recycling from an Unexplored Source by Polyphosphate Accumulating Microalgae and Cyanobacteria-A Step to Phosphorus Security in Agriculture. Front. Microbiol. 6, 1421. doi:10.3389/fmicb.2015.01421
Mulbry, W. W., and Wilkie, A. C. (2001). Growth of Benthic Freshwater Algae on Dairy Manures. J. Appl. Phycol. 13, 301–306. doi:10.1023/a:1017545116317
Muñoz, R., Köllner, C., and Guieysse, B. (2009). Biofilm Photobioreactors for the Treatment of Industrial Wastewaters. J. Hazard. Mater. 161 (1), 29–34. doi:10.1016/j.jhazmat.2008.03.018
Murdock, J. N., Dodds, W. K., and Wetzel, D. L. (2008). Subcellular Localized Chemical Imaging of Benthic Algal Nutritional Content via HgCdTe Array FT-IR. Vibrational Spectrosc. 48 (2), 179–188. doi:10.1016/j.vibspec.2007.12.011
Nandimandalam, H., and Gude, V. G. (2019). Indigenous Biosensors for In Situ Hydrocarbon Detection in Aquatic Environments. Mar. Pollut. Bull. 149, 110643. doi:10.1016/j.marpolbul.2019.110643
Naumann, T., Çebi, Z., Podola, B., and Melkonian, M. (2013). Growing Microalgae as Aquaculture Feeds on Twin-Layers: a Novel Solid-State Photobioreactor. J. Appl. Phycol 25 (5), 1413–1420. doi:10.1007/s10811-012-9962-6
Neu, T. R., and Lawrence, J. R. (2015). Innovative Techniques, Sensors, and Approaches for Imaging Biofilms at Different Scales. Trends Microbiology 23 (4), 233–242. doi:10.1016/j.tim.2014.12.010
Neu, T. R., and Lawrence, J. R. (2014). Investigation of Microbial Biofilm Structure by Laser Scanning Microscopy. Adv. Biochem. Eng. Biotechnol. 146, 1–51. doi:10.1007/10_2014_272
Neu, T. R., Manz, B., Volke, F., Dynes, J. J., Hitchcock, A. P., and Lawrence, J. R. (2010). Advanced Imaging Techniques for Assessment of Structure, Composition and Function in Biofilm Systems. FEMS Microbiol. Ecolology 72 (1), 1–21. doi:10.1111/j.1574-6941.2010.00837.x
Ng, F.-L., Phang, S.-M., Periasamy, V., Yunus, K., and Fisher, A. C. (2014). Evaluation of Algal Biofilms on Indium Tin Oxide (ITO) for Use in Biophotovoltaic Platforms Based on Photosynthetic Performance. PLoS One 9 (5), e97643. doi:10.1371/journal.pone.0097643
Norton, T., Thompson, R., Pope, J., Veltkamp, C., Banks, B., Howard, C., et al. (1998). Using Confocal Laser Scanning Microscopy, Scanning Electron Microscopy and Phase Contrast Light Microscopy to Examine marine Biofilms. Aquat. Microb. Ecol. 16, 199–204. doi:10.3354/ame016199
Nováková, R., and Neustupa, J. (2015). Microalgal Biofilms on Common Yew needles in Relation to Anthropogenic Air Pollution in Urban Prague, Czech Republic. Sci. Total Environ. 508, 7–12. doi:10.1016/j.scitotenv.2014.11.031
Novoa, A. F., Vrouwenvelder, J. S., and Fortunato, L. (2021). Membrane Fouling in Algal Separation Processes: A Review of Influencing Factors and Mechanisms. Front. Chem. Eng. 3 (21). doi:10.3389/fceng.2021.687422
Nuzzo, G., Gallo, C., d'Ippolito, G., Cutignano, A., Sardo, A., and Fontana, A. (2013). Composition and Quantitation of Microalgal Lipids by ERETIC 1H NMR Method. Mar. Drugs 11 (10), 3742–3753. doi:10.3390/md11103742
Olguín, E. J. (2012). Dual Purpose Microalgae-Bacteria-Based Systems that Treat Wastewater and Produce Biodiesel and Chemical Products within a Biorefinery. Biotechnol. Adv. 30 (5), 1031–1046. doi:10.1016/j.biotechadv.2012.05.001
Osorio, J. H. M., Benettoni, P., Schmidt, M., Stryhanyuk, H., Schmitt-Jansen, M., Pinto, G., et al. (2019a). Investigation of Architecture Development and Phosphate Distribution in Chlorella Biofilm by Complementary Microscopy Techniques. FEMS Microbiol. Ecol. 95 (4). doi:10.1093/femsec/fiz029
Ozkan, A., and Berberoglu, H. (2013a). Cell to Substratum and Cell to Cell Interactions of Microalgae. Colloids Surf. B: Biointerfaces 112, 302–309. doi:10.1016/j.colsurfb.2013.08.007
Ozkan, A., and Berberoglu, H. (2013b). Physico-chemical Surface Properties of Microalgae. Colloids Surf. B: Biointerfaces 112, 287–293. doi:10.1016/j.colsurfb.2013.08.001
Ozkan, A., Kinney, K., Katz, L., and Berberoglu, H. (2012). Reduction of Water and Energy Requirement of Algae Cultivation Using an Algae Biofilm Photobioreactor. Bioresour. Tech. 114, 542–548. doi:10.1016/j.biortech.2012.03.055
Palmer, J., Flint, S., and Brooks, J. (2007). Bacterial Cell Attachment, the Beginning of a Biofilm. J. Ind. Microbiol. Biotechnol. 34, 577–588. doi:10.1007/s10295-007-0234-4
Pätzold, R., Keuntje, M., and Anders-von Ahlften, A. (2006). A New Approach to Non-destructive Analysis of Biofilms by Confocal Raman Microscopy. Anal. Bioanal. Chem. 386 (2), 286–292. doi:10.1007/s00216-006-0663-3
Petroff, A. P., Wu, T.-D., Liang, B., Mui, J., Guerquin-Kern, J.-L., Vali, H., et al. (2011). Reaction-diffusion Model of Nutrient Uptake in a Biofilm: Theory and experiment. J. Theor. Biol. 289, 90–95. doi:10.1016/j.jtbi.2011.08.004
Podola, B., Li, T., and Melkonian, M. (2017). Porous Substrate Bioreactors: A Paradigm Shift in Microalgal Biotechnology?. Trends Biotechnol. 35 (2), 121–132. doi:10.1016/j.tibtech.2016.06.004
Pohlon, E., Marxsen, J., and Küsel, K. (2009). Pioneering Bacterial and Algal Communities and Potential Extracellular Enzyme Activities of Stream Biofilms. FEMS Microbiol. Ecol. 71, 364–373. doi:10.1111/j.1574-6941.2009.00817.x
Posadas, E., García-Encina, P.-A., Soltau, A., Domínguez, A., Díaz, I., and Muñoz, R. (2013). Carbon and Nutrient Removal from Centrates and Domestic Wastewater Using Algal-Bacterial Biofilm Bioreactors. Bioresour. Tech. 139, 50–58. doi:10.1016/j.biortech.2013.04.008
Posadas, E., García-Encina, P. A., Domínguez, A., Díaz, I., Becares, E., Blanco, S., et al. (2014). Enclosed Tubular and Open Algal-Bacterial Biofilm Photobioreactors for Carbon and Nutrient Removal from Domestic Wastewater. Ecol. Eng. 67, 156–164. doi:10.1016/j.ecoleng.2014.03.007
Przytocka-Jusiak, M., Baszczyk, M., Kosinska, E., and Bisz-Konarzewska, A. (1984). Removal of Nitrogen from Industrial Wastewaters with the Use of Algal Rotating Disks and Denitrification Packed Bed Reactor. Water Res. 18, 1077–1082. doi:10.1016/0043-1354(84)90221-5
Qureshi, N., Annous, B. A., Ezeji, T. C., Karcher, P., and Maddox, I. S. (2005). Biofilm Reactors for Industrial Bioconversion Processes: Employing Potential of Enhanced Reaction Rates. Microb. Cel Fact 4, 24. doi:10.1186/1475-2859-4-24
Rasband, W. S. (1997–2008). ImageJ online at http://rsb.info.nih.gov/ij/. [Accessed 2018]. .
Ravindran, B., Gupta, S., Cho, W.-M., Kim, J., Lee, S., Jeong, K.-H., et al. (2016). Microalgae Potential and Multiple Roles-Current Progress and Future Prospects-An Overview. Sustainability 8 (12), 1215. doi:10.3390/su8121215
Richmond, A. (2004). Handbook of Microalgal Culture: Biotechnology and Applied Phycology. Oxford, OX: Blackwell Publishing Ltd.
Roeselers, G., Loosdrecht, M. C. M. v., and Muyzer, G. (2008). Phototrophic Biofilms and Their Potential Applications. J. Appl. Phycol 20 (3), 227–235. doi:10.1007/s10811-007-9223-2
Roeselers, G., van Loosdrecht, M. C. M., and Muyzer, G. (2007). Heterotrophic Pioneers Facilitate Phototrophic Biofilm Development. Microb. Ecol. 54 (3), 578–585. doi:10.1007/s00248-007-9238-x
Romaní, A. M., Fund, K., Artigas, J., Schwartz, T., Sabater, S., and Obst, U. (2008). Relevance of Polymeric Matrix Enzymes during Biofilm Formation. Microb. Ecol. 56, 427–436. doi:10.1007/s00248-007-9361-8
Rummel, C. D., Jahnke, A., Gorokhova, E., Kühnel, D., and Schmitt-Jansen, M. (2017). Impacts of Biofilm Formation on the Fate and Potential Effects of Microplastic in the Aquatic Environment. Environ. Sci. Technol. Lett. 4 (7), 258–267. doi:10.1021/acs.estlett.7b00164
Saccomano, S. C., Jewell, M. P., and Cash, K. J. (2021). A Review of Chemosensors and Biosensors for Monitoring Biofilm Dynamics. Sensors Actuators Rep. 3, 100043. doi:10.1016/j.snr.2021.100043
Sanmartín, P., Aira, N., Devesa-Rey, R., Silva, B., and Prieto, B. (2010). Relationship between Color and Pigment Production in Two Stone Biofilm-Forming Cyanobacteria (Nostocsp. PCC 9104 andNostocsp. PCC 9025). Biofouling 26 (5), 499–509. doi:10.1080/08927011003774221
Sanmartín, P., Vázquez-Nion, D., Silva, B., and Prieto, B. (2012). Spectrophotometric Color Measurement for Early Detection and Monitoring of Greening on Granite Buildings. Biofouling 28 (3), 329–338. doi:10.1080/08927014.2012.673220
Sanmartín, P., Villa, F., Silva, B., Cappitelli, F., and Prieto, B. (2011). Color Measurements as a Reliable Method for Estimating Chlorophyll Degradation to Phaeopigments. Biodegradation 22 (4), 763–771. doi:10.1007/s10532-010-9402-8
Schmidt, M., Byrne, J. M., and Maasilta, I. J. (2021). Bio-imaging with the Helium-Ion Microscope: A Review. Beilstein J. Nanotechnol. 12, 1–23. doi:10.3762/bjnano.12.1
Schnurr, P. J., and Allen, D. G. (2015). Factors Affecting Algae Biofilm Growth and Lipid Production: A Review. Renew. Sust. Energ. Rev. 52, 418–429. doi:10.1016/j.rser.2015.07.090
Schnurr, P. J., Espie, G. S., and Allen, D. G. (2013). Algae Biofilm Growth and the Potential to Stimulate Lipid Accumulation through Nutrient Starvation. Bioresour. Tech. 136, 337–344. doi:10.1016/j.biortech.2013.03.036
Schreiber, U., Hormann, H., Neubauer, C., and Klughammer, C. (1995). Assessment of Photosystem II Photochemical Quantum Yield by Chlorophyll Fluorescence Quenching Analysis. Funct. Plant Biol. 22, 209–220. doi:10.1071/pp9950209
Schultz, M. P., Finlay, J. A., Callow, M. E., and Callow, J. A. (2000). A Turbulent Channel Flow Apparatus for the Determination of the Adhesion Strength of Microfouling Organisms. Biofouling 15, 243–251. doi:10.1080/08927010009386315
Schuurmans, R. M., van Alphen, P., Schuurmans, J. M., Matthijs, H. C. P., and Hellingwerf, K. J. (2015). Comparison of the Photosynthetic Yield of Cyanobacteria and Green Algae: Different Methods Give Different Answers. PLoS One 10 (9), e0139061. doi:10.1371/journal.pone.0139061
Sekar, R., Venugopalan, V. P., Nandakumar, K., Nair, K. V. K., and Rao, V. N. R. (2004a). Early Stages of Biofilm Succession in a Lentic Freshwater Environment. Hydrobiologia 512, 97–108. doi:10.1023/b:hydr.0000020314.69538.2c
Sekar, R., Venugopalan, V. P., Satpathy, K. K., Nair, K. V. K., and Rao, V. N. R. (2004b). Laboratory Studies on Adhesion of Microalgae to Hard Substrates. Hydrobiologia 512, 109–116. doi:10.1023/b:hydr.0000020315.40349.38
Sharma, A., Sharma, S., Sharma, K., Chetri, S. P. K., Vashishtha, A., Singh, P., et al. (2016). Algae as Crucial Organisms in Advancing Nanotechnology: a Systematic Review. J. Appl. Phycol 28 (3), 1759–1774. doi:10.1007/s10811-015-0715-1
Sharma, S. K., Nelson, D. R., Abdrabu, R., Khraiwesh, B., Jijakli, K., Arnoux, M., et al. (2015). An Integrative Raman Microscopy-Based Workflow for Rapid In Situ Analysis of Microalgal Lipid Bodies. Biotechnol. Biofuels 8, 164. doi:10.1186/s13068-015-0349-1
Shen, Y., Xu, X., Zhao, Y., and Lin, X. (2014). Influence of Algae Species, Substrata and Culture Conditions on Attached Microalgal Culture. Bioproc. Biosyst Eng 37 (3), 441–450. doi:10.1007/s00449-013-1011-6
Shen, Y., Zhang, H., Xu, X., and Lin, X. (2015). Biofilm Formation and Lipid Accumulation of Attached Culture of Botryococcus Braunii. Bioproc. Biosyst Eng 38 (3), 481–488. doi:10.1007/s00449-014-1287-1
Sheng, G.-P., Yu, H.-Q., and Li, X.-Y. (2010). Extracellular Polymeric Substances (EPS) of Microbial Aggregates in Biological Wastewater Treatment Systems: a Review. Biotechnol. Adv. 28, 882–894. doi:10.1016/j.biotechadv.2010.08.001
Shi, J., Podola, B., and Melkonian, M. (2014). Application of a Prototype-Scale Twin-Layer Photobioreactor for Effective N and P Removal from Different Process Stages of Municipal Wastewater by Immobilized Microalgae. Bioresour. Tech. 154, 260–266. doi:10.1016/j.biortech.2013.11.100
Silva-Aciares, F. R., and Riquelme, C. E. (2008). Comparisons of the Growth of Six Diatom Species between Two Configurations of Photobioreactors. Aquacultural Eng. 38 (1), 26–35. doi:10.1016/j.aquaeng.2007.10.005
Sirmerova, M., Prochazkova, G., Siristova, L., Kolska, Z., and Branyik, T. (2013). Adhesion of Chlorella Vulgaris to Solid Surfaces, as Mediated by Physicochemical Interactions. J. Appl. Phycol 25 (6), 1687–1695. doi:10.1007/s10811-013-0015-6
Sommerfeld Ross, S., Tu, M. H., Falsetta, M. L., Ketterer, M. R., Kiedrowski, M. R., Horswill, A. R., et al. (2014). Quantification of Confocal Images of Biofilms Grown on Irregular Surfaces. J. Microbiol. Methods 100, 111–120. doi:10.1016/j.mimet.2014.02.020
Spolaore, P., Joannis-Cassan, C., Duran, E., and Isambert, A. (2006). Commercial Applications of Microalgae. J. Biosci. Bioeng. 101 (2), 87–96. doi:10.1263/jbb.101.87
Staudt, C., Horn, H., Hempel, D. C., and Neu, T. R. (2004). Volumetric Measurements of Bacterial Cells and Extracellular Polymeric Substance Glycoconjugates in Biofilms. Biotechnol. Bioeng. 88 (5), 585–592. doi:10.1002/bit.20241
Stewart, P. S., and Franklin, M. J. (2008). Physiological Heterogeneity in Biofilms. Nat. Rev. Microbiol. 6 (3), 199–210. doi:10.1038/nrmicro1838
Stoodley, P., Sauer, K., Davies, D. G., and Costerton, J. W. (2002). Biofilms as Complex Differentiated Communities. Annu. Rev. Microbiol. 56, 187–209. doi:10.1146/annurev.micro.56.012302.160705
Strieth, D., Ulber, R., and Muffler, K. (2018). Application of Phototrophic Biofilms: from Fundamentals to Processes. Bioproc. Biosyst Eng 41 (3), 295–312. doi:10.1007/s00449-017-1870-3
Sukačová, K., Trtílek, M., and Rataj, T. (2015). Phosphorus Removal Using a Microalgal Biofilm in a New Biofilm Photobioreactor for Tertiary Wastewater Treatment. Water Res. 71, 55–63. doi:10.1016/j.watres.2014.12.049
Szewzyk, U., and Schink, B. (1987). Surface Colonization by and Life Cycle of Pelobacter Acidigallici Studied in a Continuous-Flow Microchamber. J. Gen. Microbiol. 134, 183–190.
Talukder, M. M. R., Das, P., and Wu, J. C. (2014). Immobilization of Microalgae on Exogenous Fungal Mycelium: A Promising Separation Method to Harvest Both marine and Freshwater Microalgae. Biochem. Eng. J. 91, 53–57. doi:10.1016/j.bej.2014.07.001
Tampion, J., and Tampion, M. D. (1987). Immobilized Cells: Principles and Applications. Cambridge, UK: Cambridge University Press.
Tan, J. S., Lee, S. Y., Chew, K. W., Lam, M. K., Lim, J. W., Ho, S.-H., et al. (2020). A Review on Microalgae Cultivation and Harvesting, and Their Biomass Extraction Processing Using Ionic Liquids. Bioengineered 11 (1), 116–129. doi:10.1080/21655979.2020.1711626
Telgmann, U., Horn, H., and Morgenroth, E. (2004). Influence of Growth History on Sloughing and Erosion from Biofilms. Water Res. 38, 3671–3684. doi:10.1016/j.watres.2004.05.020
Torpey, W. N., Heukelekian, H., Kaplowsky, A. J., and Epstein, R. (1971). Rotating Disks with Biological Growths Prepare Wastewater for Disposal or Reuse. J. Water Pollut. Control. Fed. 43, 2181–2188.
Toyofuku, M., Inaba, T., Kiyokawa, T., Obana, N., Yawata, Y., and Nomura, N. (2016). Environmental Factors that Shape Biofilm Formation. Biosci. Biotechnol. Biochem. 80 (1), 7–12. doi:10.1080/09168451.2015.1058701
Tran, T. H., Govin, A., Guyonnet, R., Grosseau, P., Lors, C., Damidot, D., et al. (2014). Influence of the Intrinsic Characteristics of Mortars on Their Biofouling by Pigmented Organisms: Comparison between Laboratory and Field-Scale Experiments. Int. Biodeterioration Biodegradation 86, 334–342. doi:10.1016/j.ibiod.2013.10.005
Tran, T. H., Govin, A., Guyonnet, R., Grosseau, P., Lors, C., Garcia-Diaz, E., et al. (2012). Influence of the Intrinsic Characteristics of Mortars on Biofouling by Klebsormidium Flaccidum. Int. Biodeterioration Biodegradation 70, 31–39. doi:10.1016/j.ibiod.2011.10.017
Urrutia, I., Serra, J. L., and Llama, M. J. (1995). Nitrate Removal from Water by Scenedesmus Obliquus Immobilized in Polymeric Foams. Enzyme Microb. Tech. 17, 200–205. doi:10.1016/0141-0229(94)00008-f
Valladares Linares, R., Fortunato, L., Farhat, N. M., Bucs, S. S., Staal, M., Fridjonsson, E. O., et al. (2016). Mini-review: Novel Non-destructivein Situbiofilm Characterization Techniques in Membrane Systems. Desalination Water Treat. 57 (48-49), 22894–22901. doi:10.1080/19443994.2016.1180483
van Loosdrecht, M. C. M., Eikelboom, D., Gjaltema, A., Mulder, A., Tijhuis, L., and Heijnen, J. J. (1995). Biofilm Structures. Sci. Tech. 32, 35–43. doi:10.2166/wst.1995.0258
Vert, M., Doi, Y., Hellwich, K.-H., Hess, M., Hodge, P., Kubisa, P., et al. (2012). Terminology for Biorelated Polymers and Applications (IUPAC Recommendations 2012). Pure Appl. Chem. 84 (2), 377–410. doi:10.1351/pac-rec-10-12-04
Vieira, M. J., Melo, L. F., and Pinheiro, M. M. (1993). Biofilm Formation: Hydrodynamic Effects on Internal Diffusion and Structure. Biofouling 7, 67–80. doi:10.1080/08927019309386244
Wagner, M., and Horn, H. (2017). Optical Coherence Tomography in Biofilm Research: A Comprehensive Review. Biotechnol. Bioeng. 114 (7), 1386–1402. doi:10.1002/bit.26283
Wagner, M., Manz, B., Volke, F., Neu, T. R., and Horn, H. (2010). Online Assessment of Biofilm Development, Sloughing and Forced Detachment in Tube Reactor by Means of Magnetic Resonance Microscopy. Biotechnol. Bioeng. 107 (1), 172–181. doi:10.1002/bit.22784
Wang, J., Liu, W., and Liu, T. (2017). Biofilm Based Attached Cultivation Technology for Microalgal Biorefineries-A Review. Bioresour. Tech. 244 (Pt 2), 1245–1253. doi:10.1016/j.biortech.2017.05.136
Wang, X., Nordlander, E., Thorin, E., and Yan, J. (2013). Microalgal Biomethane Production Integrated with an Existing Biogas Plant: A Case Study in Sweden. Appl. Energ. 112, 478–484. doi:10.1016/j.apenergy.2013.04.087
Wang, Y., Ho, S.-H., Cheng, C.-L., Guo, W.-Q., Nagarajan, D., Ren, N.-Q., et al. (2016a). Perspectives on the Feasibility of Using Microalgae for Industrial Wastewater Treatment. Bioresour. Tech. 222, 485–497. doi:10.1016/j.biortech.2016.09.106
Wang, Y., Qu, T., Zhao, X., Tang, X., Xiao, H., and Tang, X. (2016b). A Comparative Study of the Photosynthetic Capacity in Two green Tide Macroalgae Using Chlorophyll Fluorescence. Springerplus 5 (1), 775. doi:10.1186/s40064-016-2488-7
Wei, X., Jie, D., Cuello, J. J., Johnson, D. J., Qiu, Z., and He, Y. (2014). Microalgal Detection by Raman Microspectroscopy. Trac Trends Anal. Chem. 53, 33–40. doi:10.1016/j.trac.2013.09.012
Wijeyekoon, S., Mino, T., Satoh, H., and Matsuo, T. (2004). Effects of Substrate Loading Rate on Biofilm Structure. Water Res. 38, 2479–2488. doi:10.1016/j.watres.2004.03.005
Wijffels, R. H., Kruse, O., and Hellingwerf, K. J. (2013). Potential of Industrial Biotechnology with Cyanobacteria and Eukaryotic Microalgae. Curr. Opin. Biotechnol. 24 (3), 405–413. doi:10.1016/j.copbio.2013.04.004
Wiley, P. E., Campbell, J. E., and McKuin, B. (2011). Production of Biodiesel and Biogas from Algae: A Review of Process Train Options. Water Environ. Res. 83 (4), 326–338. doi:10.2175/106143010X10.2175/106143010x12780288628615
Wimpenny, J., Manz, W., and Szewzyk, U. (2000). Heterogeneity in Biofilms: Table 1. FEMS Microbiol. Rev. 24, 661–671. doi:10.1111/j.1574-6976.2000.tb00565.x
Wongthanate, J., and Polprasert, C. (2015). Immobilized Biofilm in Thermophilic Biohydrogen Production Using Synthetic versus Biological Materials. Braz. Arch. Biol. Technol. 58 (1), 124–130. doi:10.1590/s1516-8913201502895
Woodcock, S., and Sloan, W. T. (2017). Biofilm Community Succession: a Neutral Perspective. Microbiology 163, 664–668. doi:10.1099/mic.0.000472
Xavier, J. B., White, D. C., and Almeida, J. S. (2003). Automated Biofilm Morphology Quantification from Confocal Laser Scanning Microscopy Imaging. Wat. Sci. Technol. 47 (5), 31–37. doi:10.2166/wst.2003.0273
Yang, Y., Heine, R., Xu, F., Suhonen, H., Helfen, L., Rosenhahn, A., et al. (2013). Correlative Imaging of Structural and Elemental Composition of Bacterial Biofilms, 463, 012053. doi:10.1088/1742-6596/463/1/012053Correlative Imaging of Structural and Elemental Composition of Bacterial BiofilmsJ. Phys. Conf. Ser.
Yawata, Y., Toda, K., Setoyama, E., Fukuda, J., Suzuki, H., Uchiyama, H., et al. (2010). Monitoring Biofilm Development in a Microfluidic Device Using Modified Confocal Reflection Microscopy. J. Biosci. Bioeng. 110, 377–380. doi:10.1016/j.jbiosc.2010.04.002
Young, A. M. (2011). Zeolite‐Based Agae Biofilm Rotating Photobioreactor for Algae and Biomass Production Mster of Science. Utah State University. http://digitalcommons.usu.edu/etd/986
Zabed, H. M., Akter, S., Yun, J., Zhang, G., Zhang, Y., and Qi, X. (2020). Biogas from Microalgae: Technologies, Challenges and Opportunities. Renew. Sust. Energ. Rev. 117, 109503. doi:10.1016/j.rser.2019.109503
Zeng, X., Guo, X., Su, G., Danquah, M. K., Zhang, S., Lu, Y., et al. (2015). Bioprocess Considerations for Microalgal-Based Wastewater Treatment and Biomass Production. Renew. Sust. Energ. Rev. 42, 1385–1392. doi:10.1016/j.rser.2014.11.033
Zeng, Z., Cai, X., Wang, P., Guo, Y., Liu, X., Li, B., et al. (2017). Biofilm Formation and Heat Stress Induce Pyomelanin Production in Deep-Sea Pseudoalteromonas Sp. SM9913. Front. Microbiol. 8, 1822. doi:10.3389/fmicb.2017.01822
Zhang, C., Lu, J., and Wu, J. (2019). Adsorptive Removal of Polycyclic Aromatic Hydrocarbons by Detritus of green Tide Algae Deposited in Coastal Sediment. Sci. Total Environ. 670, 320–327. doi:10.1016/j.scitotenv.2019.03.296
Zhang, D., Fung, K. Y., and Ng, K. M. (2014). Novel Filtration Photobioreactor for Efficient Biomass Production. Ind. Eng. Chem. Res. 53, 12927–12934. doi:10.1021/ie501913k
Zhang, L., Chen, L., Wang, J., Chen, Y., Gao, X., Zhang, Z., et al. (2015). Attached Cultivation for Improving the Biomass Productivity of Spirulina Platensis. Bioresour. Tech. 181, 136–142. doi:10.1016/j.biortech.2015.01.025
Zhang, Q., Liu, C., Li, Y., Yu, Z., Chen, Z., Ye, T., et al. (2017). Cultivation of Algal Biofilm Using Different Lignocellulosic Materials as Carriers. Biotechnol. Biofuels 10, 115. doi:10.1186/s13068-017-0799-8
Zheng, Y., Huang, Y., Liao, Q., Fu, Q., Xia, A., and Zhu, X. (2017). Impact of the Accumulation and Adhesion of Released Oxygen during Scenedesmus Obliquus Photosynthesis on Biofilm Formation and Growth. Bioresour. Tech. 244 (Pt 1), 198–205. doi:10.1016/j.biortech.2017.07.145
Zhuang, L.-L., Azimi, Y., Yu, D., Wang, W.-L., Wu, Y.-H., Dao, G.-H., et al. (2016). Enhanced Attached Growth of Microalgae Scenedesmus. LX1 through Ambient Bacterial Pre-coating of Cotton Fiber Carriers. Bioresour. Tech. 218, 643–649. doi:10.1016/j.biortech.2016.07.013
Zippel, B., and Neu, T. R. (2005). Growth and Structure of Phototrophic Biofilms under Controlled Light Conditions. Water Sci. Tech. 52 (7), 203–209. doi:10.2166/wst.2005.0202
Zippel, B., Rijstenbil, J., and Neu, T. R. (2007). A Flow-Lane Incubator for Studying Freshwater and marine Phototrophic Biofilms. J. Microbiol. Methods 70 (2), 336–345. doi:10.1016/j.mimet.2007.05.013
Zkeri, E., Iliopoulou, A., Katsara, A., Korda, A., Aloupi, M., Gatidou, G., et al. (2021). Comparing the Use of a Two-Stage MBBR System with a Methanogenic MBBR Coupled with a Microalgae Reactor for Medium-Strength Dairy Wastewater Treatment. Bioresour. Tech. 323, 124629. doi:10.1016/j.biortech.2020.124629
Keywords: biofilm bioreactors, immobilization, wastewater treatment, carrier material, non-destructive techniques, life cycle, biofilm formation, biotechnological application
Citation: Moreno Osorio JH, Pollio A, Frunzo L, Lens PNL and Esposito G (2021) A Review of Microalgal Biofilm Technologies: Definition, Applications, Settings and Analysis. Front. Chem. Eng. 3:737710. doi: 10.3389/fceng.2021.737710
Received: 07 July 2021; Accepted: 23 August 2021;
Published: 12 October 2021.
Edited by:
Albert Guisasola, Universitat Autònoma de Barcelona, SpainReviewed by:
Sabeela Beevi Ummalyma, Institute of Bioresources and Sustainable Development (IBSD), IndiaCopyright © 2021 Moreno Osorio, Pollio, Frunzo, Lens and Esposito. This is an open-access article distributed under the terms of the Creative Commons Attribution License (CC BY). The use, distribution or reproduction in other forums is permitted, provided the original author(s) and the copyright owner(s) are credited and that the original publication in this journal is cited, in accordance with accepted academic practice. No use, distribution or reproduction is permitted which does not comply with these terms.
*Correspondence: Jairo Hernán Moreno Osorio, amhlcm5hbm1AZ21haWwuY29t
Disclaimer: All claims expressed in this article are solely those of the authors and do not necessarily represent those of their affiliated organizations, or those of the publisher, the editors and the reviewers. Any product that may be evaluated in this article or claim that may be made by its manufacturer is not guaranteed or endorsed by the publisher.
Research integrity at Frontiers
Learn more about the work of our research integrity team to safeguard the quality of each article we publish.