- 1Department of Chemical Engineering, De La Salle University, Manila, Philippines
- 2College of Physical Sciences and Engineering, Cardiff University, Cardiff, United Kingdom
Ammonia has been proposed as a replacement for fossil fuels. Like hydrogen, emissions from the combustion of ammonia are carbon-free. Unlike hydrogen, ammonia is more energy dense, less explosive, and there exists extensive experience in its distribution. However, ammonia has a low flame speed and combustion emits nitrogen oxides. Ammonia is produced via the Haber-Bosch process which consumes large amounts of fossil fuels and requires high temperatures and pressures. A life cycle assessment to determine potential environmental advantages and disadvantages of using ammonia is necessary. In this work, emissions data from experiments with generating heat from tangential swirl burners using ammonia cofired with methane employing currently available technologies were utilized to estimate the environmental impacts that may be expected. Seven ammonia sources were combined with two methane sources to create 14 scenarios. The impacts from these 14 scenarios were compared to those expected from using pure methane. The results show that using ammonia from present-day commercial production methods will result in worse global warming potentials than using methane to generate the same amount of heat. Only two scenarios, methane from biogas combined with ammonia from hydrogen from electricity and nuclear power via electrolysis and subsequent ammonia synthesis using nitrogen from the air, showed reductions in global warming potential. Subsequent analysis of other environmental impacts for these two scenarios showed potentially lower impacts for respiratory organics, terrestrial acidification-nutrification and aquatic acidification depending on how the burner is operated. The other eight environmental impacts were worse than the methane scenario because of activities intrinsic to the generation of electricity via wind power and nuclear fission. The results show that generating heat from a tangential swirl burner using ammonia currently available technologies will not necessarily result in improved environmental benefits in all categories. Improvements in renewable energy technologies could change these results positively. Other means of producing ammonia and improved means of converting ammonia to energy must continue to be explored.
Introduction
The search for alternative fuels has led to numerous proposals including biodiesel (Knothe and Razon, 2017), bioethanol (Ferreira et al., 2018), methanol (Verhelst et al., 2019), and hydrogen (Acar and Dincer, 2019). Hydrogen has received a considerable amount of attention because of the prospect for zero tailpipe-carbon emissions. However, hydrogen is difficult to distribute because it is explosive, and it has a low energy density (Zamfirescu and Dincer, 2008). Instead, ammonia has been proposed as an alternative. Ammonia also has zero tailpipe carbon emissions but is more energy dense, may be stored at lower temperatures and is not explosive (Zamfirescu and Dincer, 2008). Ammonia has also been used extensively as a fertilizer and a refrigerant so there is extensive experience with its use. It has also been proposed for many other applications related to climate change mitigation and alternative fuel production (Razon, 2018). The use of ammonia as a fuel is not exactly new, with a patent having been filed as early as 1937 (Valera-Medina et al., 2018). Fuel ammonia has been tested in reciprocating internal combustion engines as a gasoline or diesel substitute (Reiter and Kong, 2011); direct ammonia fuel cells (Afif et al., 2016) and as a coal substitute (Zhao et al., 2017). Its use for power applications has been comprehensively reviewed recently (Valera-Medina et al., 2018).
The use of ammonia presents many challenges which require further research and understanding. Some of these challenges fall within the use of materials, health and safety aspects, and public perception, leaving enough room for continuous efforts on the investigation of these parameters for progression in the technology (Kobayashi et al., 2019).
As for the use of materials, it is still not well-understood how ammonia will influence the integrity of high temperature resistant materials. Currently, it is well-known that ammonia is detrimental to copper-based materials. This is also the case with certain plastics, Viton and natural rubber (Valera-Medina et al., 2018). While ammonia does not degrade steels and aluminum under most handling conditions, stress corrosion cracking (SCC) can happen in carbon steels when liquid ammonia and oxygen are present (EFMA, 2007).
In terms of health and safety, handling of ammonia is well-understood, and practices are well-established worldwide. Ammonia is the second most commercialized chemical in the world and thus the industry possesses a mature infrastructure for production, handling and distribution. However, this is not the case for the use of ammonia as an energy carrier. The use of the chemical in applications such as marine engines, gas turbines and engines still requires more research and understanding, especially when it comes to release, training, and emissions mitigation. While the marine industry seems to be very interested in adopting ammonia as a fueling vector, it is clear that the International Maritime Organization (IMO) still requires more inputs and data before an international legislation is in place for the manufacturing, storage and injection of ammonia in engines that are currently being designed for that purpose (Jacobsen, 2020). Similarly, there are still unknown parameters regarding fueling stations, personnel requirements, and overall, public acceptance.
Public acceptance is another point of interest that has been barely documented. In a recent study around the perception of ammonia as an energy vector in a developed (UK) and developing (Mexico) economies. The results showed that perspectives change depending on the level of rigor of regulations and standards, with British people concerned about the smell of the chemical, whilst Mexicans were more concerned about being poisoned by a sudden release (Guati-Rojo et al., 2021).
Part of the equation in public acceptance is the overall perception of less effects on the environment when compared to fossil fuels. The widely adopted present-day commercial production of ammonia proceeds via two primary pathways which differ in how hydrogen is provided for the synthesis reaction with nitrogen gas from the air. These are via steam reforming of natural gas and partial oxidation of heavy oils and coal. Both processes consume large amounts of fossil fuels and intrinsically release considerable amounts of greenhouse gases. Thus, several other processes have been proposed. The replacement of fossil fuels with biomass feedstock has been studied (Tunå et al., 2014). In addition, the cultivation of nitrogen-fixing microorganisms for producing reactive nitrogen (Razon, 2012) and subsequent conversion to ammonia (Razon, 2014) has also been proposed. Other proposals call for the direct electrolysis of water via the use of renewable electricity for the generation of hydrogen (Bicer et al., 2017). While there are some large installations of these systems that may come online soon (Brown, 2020), they have not yet been widely adopted and thus we consider these future technologies in this paper.
In theory, combustion of ammonia should result in the emission of only nitrogen and water. Imperfect combustion, however, results in the emission of nitrogen oxides (NOx) (Okafor et al., 2020). The possibility of influencing NOx emissions by controlling the equivalence ratio (Sun et al., 2021) and by the addition of water (Ariemma et al., 2020) has been explored. Ammonia has a low flame speed and, therefore, for sustained combustion, ammonia has had to be fed with other fuels like coal (Yamamoto et al., 2018) or natural gas (Khateeb et al., 2021) have had to be fed with the ammonia. These factors offset some of the advantages of using ammonia as a fuel.
The possible trade-offs from the use of ammonia as a fuel and the availability of many alternatives for the supply of ammonia indicate that a comparative life cycle assessment of environmental impacts is imperative. Prior to commercial adoption, these analyses would naturally have to be coupled with many other considerations like profitability and social acceptability. To address this need, a few life cycle assessment and life cycle optimization studies have been done to address possible trade-offs in the use of ammonia as a fuel. Angeles et al. (2017) computed and compared nitrogen and carbon footprints of either fuel cell or internal combustion engine vehicles fed with ammonia or ammonia-diesel/gasoline combinations. It was found that a fuel cell vehicle fed with ammonia from the cyanobacteria-based process proposed in Razon (2014) gave both the lowest nitrogen footprint and the lowest carbon footprint. In a separate study, it was found that internal combustion engine vehicles fed with ammonia from biomass-based sources had lower carbon footprints than equivalent petroleum diesel or gasoline engines (Angeles et al., 2018). However, all of the ammonia vehicles had worse nitrogen footprints than the diesel or gasoline engines. Bicer and Dincer (2018a) performed life cycle assessments of city transport and power generation systems fueled by ammonia produced using an electrolytic process with electricity from nuclear power plants. They found that such systems would have considerably lower global warming potentials than equivalent petroleum-based engines. However, their results also show that there may be trade-offs resulting from higher acidification potentials primarily from the NOx emissions. Similarly, they also found that vehicles using ammonia for a nuclear-powered electrolysis process had lower global warming potentials than a wide variety of vehicles (Bicer and Dincer, 2018b). These vehicles also showed higher environmental impacts in other categories, however. The results from these studies of Bicer and Dincer are consistent with the results in Angeles et al. (2018)—lower global warming potential from lower greenhouse gas emissions if the ammonia is taken from low-carbon processes but with a trade-off from environmental impacts resulting from other emissions. Bicer and Khalid (2018) also obtained similar results upon comparing ammonia-, hydrogen-, natural gas-, and methanol-powered fuel cells for power generation.
In this paper, we aim to compare and analyze the environmental impacts of 14 scenarios for producing and combusting methane and ammonia to produce heat. The system boundaries are set to include the materials, energy and infrastructure to produce and burn the methane and ammonia. To create the 14 scenarios, we combine published life-cycle inventories of seven different sources of ammonia and two different sources of methane. For the combustion step, we use data obtained from experiments on the combustion of methane, ammonia and air mixtures in a tangential swirl burner to obtain a comparison of life cycle assessments for 11 environmental impacts if the ammonia and methane are taken from a variety of sources. To our knowledge, this is the first time a life cycle assessment has been done using data from gas-turbine based experiments for ammonia combustion. While economic and social evaluations will eventually be necessary for the complete assessment of the desirability of using ammonia as a fuel, these evaluations are beyond the scope of the present study.
Methods
Methods Overview
This study makes use of life cycle assessment (LCA), which is a technique for accounting for and evaluating the overall impact of every part of a process from “cradle-to-grave;” i.e., from production from the most basic raw materials to disposal of the finished product or from “cradle-to-gate;” i.e., from raw materials to delivery of the product.
A life cycle assessment is commonly prescribed to have four stages: (1) Goal and scope definition; (2) Inventory analysis; (3) Impact assessment and (4) Interpretation (Lee and Inaba, 2004). The goal and scope was defined in section Introduction.
We discuss the inventory analysis in section Methane and Ammonia Source Inventory. During the inventory analysis, all of the material, energy and environmental flows associated with each step of the process is identified and accounted for. It is therefore important that the system boundary be properly identified such that quantifications of environmental impacts and any comparisons made between systems are fair. The decision on whether to include the effects of new infrastructure is also an important one at this stage.
We discuss the life-cycle impact assessment (LCIA) in section Combustion of Ammonia-Methane Mixture. In a life-cycle impact assessment, the material flows are classified according to the impacts they may have on the environment. For example, the flows of CO2, methane and N2O all have impacts on global warming but at different intensities and durations. In an LCIA method, these factors are all accounted for in the impacts desired to be analyzed.
We consider the last stage (Interpretation) in the Results and Discussion and the Conclusions.
Methane and Ammonia Source Inventory
For this study, we considered 7 possible sources of ammonia combined with two different sources of methane. These are illustrated in Figure 1. The first source (Source A1) is ammonia that would be commonly obtained from a regional warehouse in Europe at present. The data for this ammonia source is taken directly from the ecoinvent process, “ammonia, liquid, at regional storehouse/RER U,” which is the representative composition of ammonia produced in Europe (Althaus et al., 2007). In this process, the ammonia is assumed to consist of 85% of ammonia produced by the steam-reforming method and 15% produced by partial oxidation. Source A2 is similar to the first except that a retrofit monoethanol amine (MEA)-based CO2 capture process is assumed to capture 99.5% of the CO2 emissions from both. The process inputs from the ecoinvent process “carbon dioxide liquid, at plant/RER U” (Althaus et al., 2007) are used to simulate the addition of a CO2 capture process to the ammonia production process.
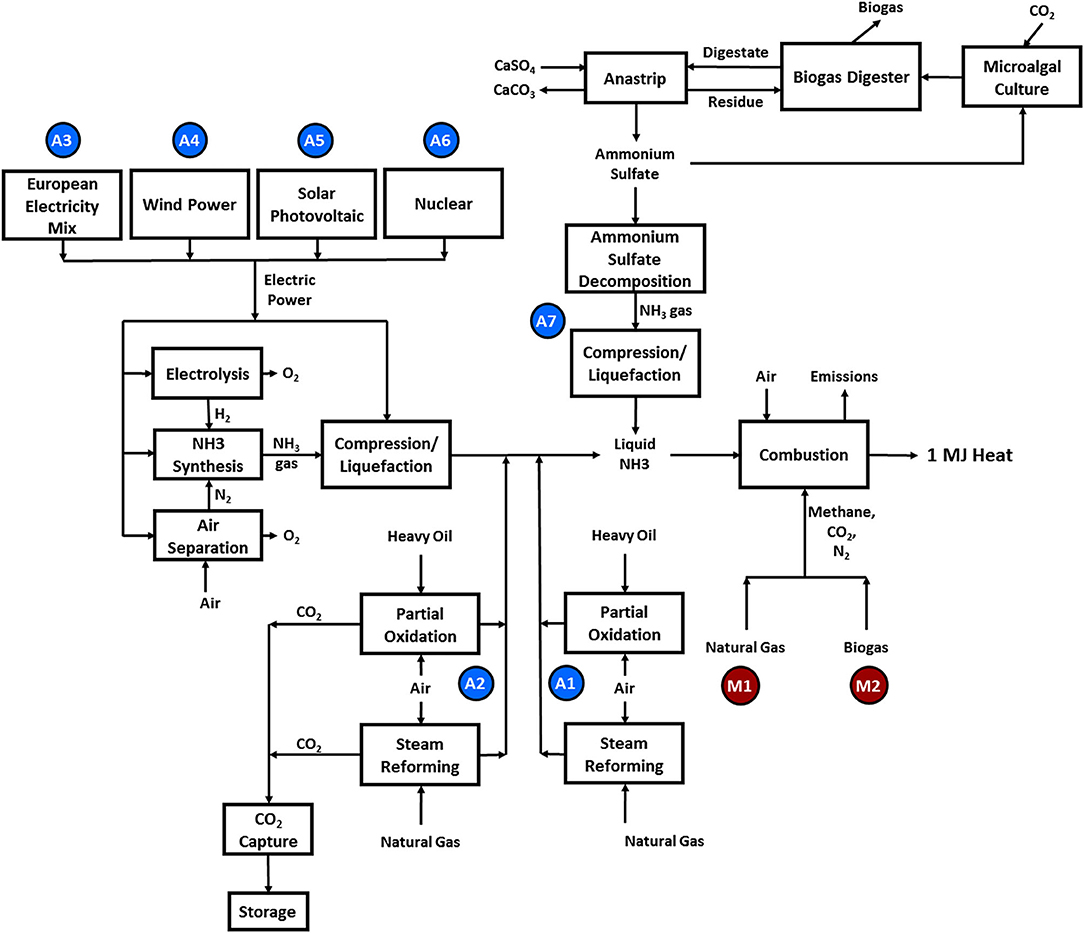
Figure 1. Simplified diagrams of all systems considered. A1–A7 denote ammonia source options. M1 and M2 are the methane source options.
Sources A3–A6 are four variants of a process consisting of water electrolysis, cryogenic air separation followed by the Haber synthesis reaction differing only in the electric power sources. Inputs for the process are taken from on an electrolysis-based synthesis process (Bicer et al., 2017), which produced gaseous ammonia as its product. To ensure comparability between systems, a liquefaction process similar to that used in Razon (2014) was added to the process in Bicer et al. (2017). The four variants differ only in the source of electricity: the European electricity mix (“UCTE mix,” Source A3); 100% wind (Source A4); 100% solar photovoltaic (Source A5) and 100% nuclear (Source A6). The data for these electricity sources are taken directly from the ecoinvent database (Dones et al., 2007).
The 7th source of ammonia (A7) is a stand-alone process proposed in Razon (2014) wherein a cyanobacteria, Anabaena ATCC33047, is cultured, harvested, and fed into a biogas reactor. From the biogas digestate, ammonium sulfate is recovered and decomposed into ammonia.
Two possible sources of methane are considered: natural gas (Source M1) and biogas as would be commonly sourced in Europe (Source M2). For Source M1, the natural gas is assumed to come from the ecoinvent data set “natural gas, production GB, at long-distance pipeline/RER U” (Faist Emmenegger et al., 2007). At distribution, it contains 85% mass% methane. 5.2% mass% C2H6, 4.4 mass% C5 hydrocarbons, 0.75 mass% CO2, and the balance is nitrogen gas. For this study, the non-methane hydrocarbons in natural gas were assumed to be methane. Under Source M2, the ecoinvent biogas dataset used is the one designated as “methane, 96 vol%, from biogas, at service station/CH U” (Jungbluth et al., 2007). It consists of 96 mol% methane, 2 mol% CO2, and 2 mol% N2.
The systems modeled are kept to the simplest possible system that may provide the prescribed power. Thus, there are no transport steps for any of the materials and there is likewise no transport assumed for the CO2 capture process. Because the system had been stripped down to the essentials, any other processes that may be added would serve to increase emissions.
Combustion of Ammonia-Methane Mixture
The data from the experiments at Cardiff University and quantities derived from these are summarized in Tables 1, 2. The combustion of each ammonia-methane mixture is presumed to take place under conditions similar to those from experiments at the Cardiff University Gas Turbine Research Centre, where ammonia, methane and air were fed premixed into a tangential swirl burner, shown in Figure 2, at chosen fuel-air equivalence ratios into a high pressure optical chamber (HPOC). The equivalence ratio, ϕ, of a particular blend is defined as the ratio of the current fuel-to-oxidizer ratio to the stoichiometric (i.e., complete combustion) fuel-to-oxidizer ratio. The “base power” in Tables 1, 2 represents the thermal power, which is the amount of energy that could be freed fully using the amount of fuel fed into the burner. A tangential swirl burner (Figure 2) was experimentally evaluated to determine emissions from the burning process of ammonia and methane. The burner was designed to handle up to five different gases simultaneously at pressures up to 9 bar. The hot burner gases were discharged to the atmosphere. In an ideal situation, those gases could be used for a combined cycle or for heating distribution. However, since this is a comparative LCA, the situation would be the same for all scenarios analyzed and, therefore, would not affect the results. Experiments were conducted using a swirler with a geometrical swirl number of 1.05. Coriolis mass flow meters were used to achieve precise measurement of flowrates with an accuracy of ±0.5% RD plus ±0.1% FS. Ammonia-methane ratios were kept at 61% NH3-39% CH4 mole fraction because lower amounts of methane were either impossible to ignite or exhibited unsteady behavior. Exhaust emissions were measured in terms of CO2, CO, and NOx composition. The equivalence ratio was varied to determine the effects on the emissions profile. For example, for the case of interest in this paper, a greater fuel-to-oxygen ratio than the required for stoichiometric values leads to equivalence ratios >1.0, regimes known as fuel rich conditions. Having an equivalence ratio of 1.2 means that there is an excess of fuel in the blend, which has been previously recognized as the ideal condition to enable mitigation of NOx emissions and stable flames using ammonia. Further experimental details may be found in Valera-Medina et al. (2017). Via simple mass balances, the exhaust emission data were converted to mass flow rates which serve as the output flow rates for the combustion process in the life cycle assessment.
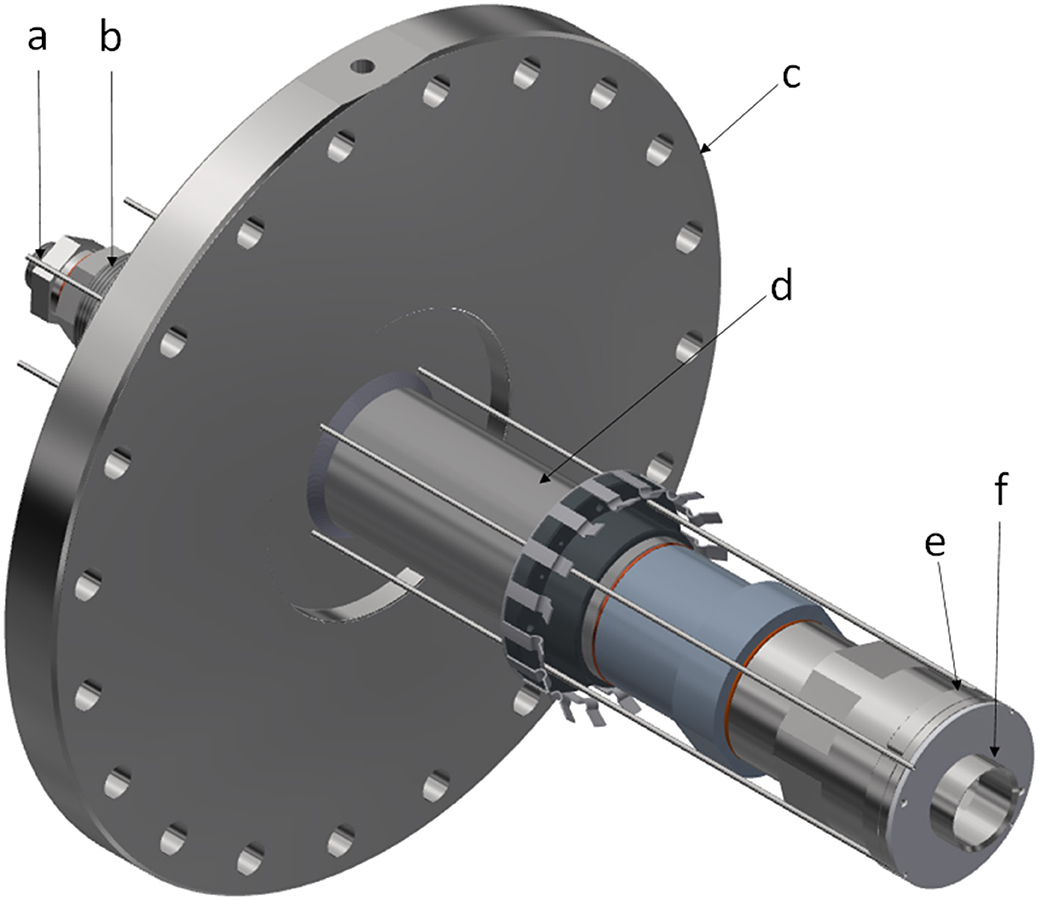
Figure 2. Experimental rig for combustion tests (a) instrumentation and pilot injection lance, (b) inlet plenum, (c) HPOC casing plate, (d) premixed chamber, (e) radial-tangential swirler, (f) burner exit nozzle. A quartz window and quartz burner confinement tube were employed for the experimental trials (not shown).
Life Cycle Impact Assessment
Impact 2002+ as implemented in Simapro® Faculty version 8.5.2 was used for the life-cycle impact assessment. Simapro® is a software tool published by Pre Sustainability®for performing Life Cycle Analyses (Simapro). Impact 2002+ is one of the most commonly used impact assessment methods. It works by characterizing the inventory of environmental flows and classifying them into mid-point categories or indicators (Jolliet et al., 2003). Using Impact 2002+, the following environmental impacts were computed: (1) global warming potential, (2) aquatic ecotoxicity, (3) aquatic eutrophication, (4) human toxicity, (5) ionizing radiation, (6) land occupation, (7) mineral extraction, (8) non-renewable energy, (9) respiratory organics, (10) ozone layer depletion, (11) terrestrial acidification/nutrification, (12) terrestrial ecotoxicity, and (13) aquatic acidification.
The functional unit for the LCA was 1 MJ of heat energy. Allocations were done according to mass flow rates.
The LCA was done as a cradle-gate assessment, the end point being the energy produced. The system boundary was defined such that the following were included in the LCA: (1) all material and energy inputs necessary for construction of infrastructure, (2) fuel and materials for energy generation and emissions to the air and water, and (3) materials and energy for the manufacture of ammonia.
The LCA of the ammonia-methane combinations were then compared to the LCA of the combustion of methane using the same experimental apparatus in Cardiff University which then serves as the control. Because fuel ammonia is primarily intended to address the problem of greenhouse gas emissions, the global warming potential was used as the primary screening criterion. If an ammonia-methane combination was found to have a higher global warming potential than the pure methane control case, then it was eliminated from further consideration. The environmental impacts for those ammonia-methane combinations with lower global warming potentials were then evaluated for possible trade-offs.
Results and Discussion
The results of the LCA for global warming potential are shown in Tables 3, 4. Virtually no differences in global warming potential arise from varying the fuel equivalence ratio and the nominal base power and thus the numbers shown in Tables 3, 4 are the averages from all of the fuel equivalence ratios. It can also be seen in Tables 3, 4 that there is very little difference in the global warming potential as the nominal base power is varied. The “nominal base power” in Tables 3, 4 corresponds to the “base power” numbers in Tables 1, 2. These results sense as more power means more fuel is consumed.
Table 3 shows that using natural gas as a co-fuel would result in global warming potentials significantly higher than the pure methane baseline control. This result is largely because of the intrinsic generation of greenhouse gases from the production of natural gas. These offset any savings from obtaining electricity from renewable sources for electrolysis. The differences vs. the methane control range from about 65 times more GWP for the A4 scenario (Ammonia by electrolysis and Haber-Bosch reaction, UCTE mix) to about 1.5 times more GWP for the A6 scenario (Ammonia by Electrolysis and Haber-Bosch reaction, Nuclear). These individual differences will be discussed further in the discussion of the GWP from using biogas as a methane source.
It is interesting to note that none of the present commercial processes, steam reforming (A1), and partial oxidation (A2), for producing ammonia would result in higher global warming potentials than using methane, regardless of the methane source. The computed GWP are more than that for the pure methane control because, for sources A1 and A2, the synthesis gas process still uses heavy oil and natural gas for its hydrogen source.
It is worthwhile to discuss the higher GWP obtained from two ammonia sources that use electricity for electrolysis. Source A3 uses electricity taken from the European grid (UCTE) for electrolysis. The energy necessary for electrolysis is high and some of the UCTE grid is still taken from fossil sources, and thus the additional global warming potential. The result for source A5, which uses solar photovoltaic electricity, seems to be counterintuitive as photovoltaic electricity is widely regarded to be environment friendly. The primary source of the high GWP for solar photovoltaic electricity is the high amount of electricity required for manufacturing the silicon wafers. In the ecoinvent database (Dones et al., 2007), this electricity is assumed to come from the existing power grid, which still contains fossil fuel.
Source A7, a proposed process for producing ammonium sulfate and ammonia from algal biomass (Razon, 2012, 2014), is computed to have a higher GWP than the methane control, although by a relatively smaller amount. While there may be a reduction in the GWP from this source, the larger amount of the ammonia-methane mixture necessary to generate an equal amount of power offsets these savings.
Indeed, of the 14 possible combinations of ammonia and methane sources, only two combinations result in global warming potentials that are lower than the 100% methane scenario. Both scenarios result from the use of biogas (Source M2). The first is the one where the ammonia is obtained via an electrolysis-based process using electricity from wind power (Source A4) and the second one is the same process but using electricity from a nuclear power plant (Source A6). The expected reductions in the global warming potential from these two scenarios are in the range of about 27–32%, with minor variations expected from the change in output power. Because the use of ammonia as a fuel is primarily intended as a means for the reduction of greenhouse gas emissions, further assessment of other environmental impacts was thus limited to these two scenarios.
Sources A4 and A6 combined with biogas were assessed for the other environmental impacts available from Impact 2002+: (1) respiratory organics; (2) ionizing radiation; (3) ozone layer depletion; (4) aquatic ecotoxicity; (5) non-renewable energy; (6) land occupation; (7) aquatic eutrophication; (8) mineral extraction; (9) terrestrial ecotoxicity; (10) human toxicity; (11) aquatic acidification; and (12) terrestrial acidification and nutrification. The results from first 10 of these 12 impact assessments are summarized in Table 3. The last two are dealt with separately.
The results from these 10 impact assessments are summarized in Table 5 and shown in Figure 3. They are grouped according to their impacts relative to the pure methane scenario. The first group consists of those with lower environmental impacts than the pure methane scenario. Both the wind and nuclear scenarios result in lower respiratory organics impact. A comparison of the impacts from using either wind power or nuclear power for the ammonia production shows almost no difference between the two. The lower impact of respiratory organics for both cases reflects the lower emissions of unburned hydrocarbons because of the replacement of methane with ammonia.
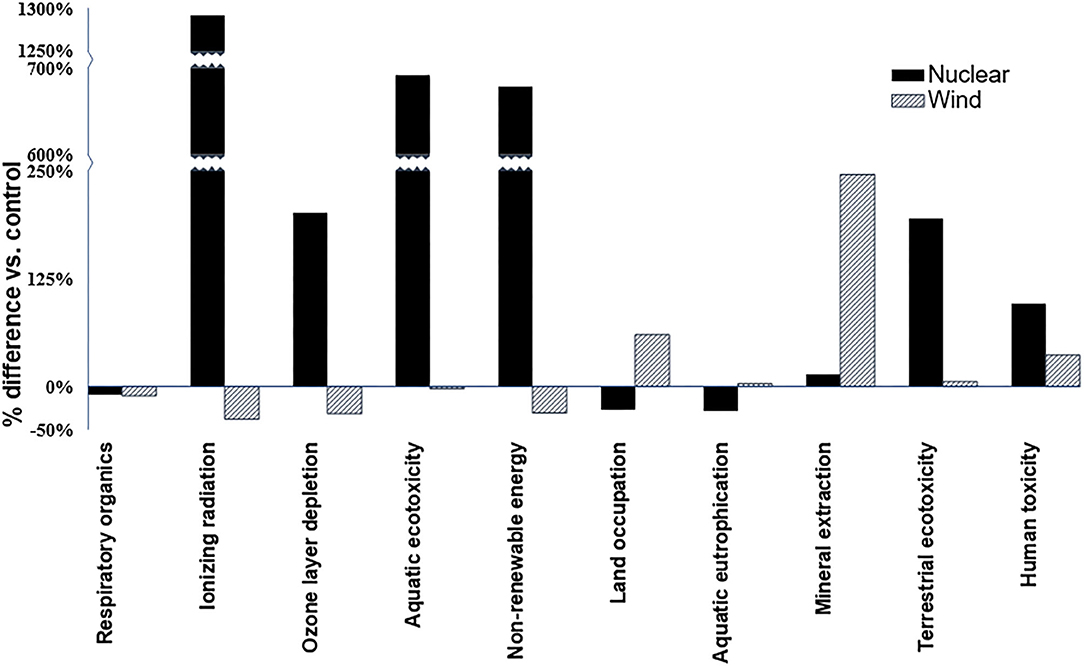
Figure 3. Environmental impacts relative to methane control. The functional unit for each of these impacts is 1 MJ of heat.
The second group of environmental impacts is that for which the wind-biogas combination has a lower environmental impact than the control scenario whereas the nuclear power-biogas combination has a higher environmental impact. These impacts are ionizing radiation, ozone layer depletion, aquatic ecotoxicity, and non-renewable energy. The larger amounts of ionizing radiation and non-renewable energy usage are expected because these are inherent to the generation of nuclear power. As seen in Table 5 and Figure 3, the differences in the impacts between nuclear-generated ammonia and the control are extremely large, on the order of 14 times more in the case of ionizing radiation. By using the network diagrams provided in Simapro, the increased amount of ozone layer depletion and aquatic ecotoxicity may be traced back to the mining and purification operations for the uranium fuel elements.
The third group of environmental impacts is that for which the nuclear power-biogas option has a lower environmental impact than the control scenario while the wind-biogas combination had a higher environmental impact. These impacts are land occupation and aquatic eutrophication. The higher amount of land occupation (60% more) may be expected as windmills occupy a higher amount of land on a per amount of energy generated. The aquatic eutrophication from the use of wind power is traced back to the copper mining operations for the windmill dynamos. The difference between the wind power case and the control is small, only 3%.
The fourth group of environmental impacts is the one wherein both the nuclear and wind options have worse environmental impacts than the methane combustion control. These are the impacts on mineral extraction, terrestrial ecotoxicity and human toxicity. All these environmental impacts can be traced back to the mining and purifications operations necessary for the generation of both types of electricity. In the case of nuclear power, these are for the uranium fuel rods while in the case of the wind power, these operations are for the building of windmills and associated equipment.
Finally, the two environmental impacts linked to the emission of nitrogen oxides, aquatic acidification and terrestrial acidification/nutrification decrease as the fuel equivalence ratio increases (Figure 4). As the fuel equivalence ratio increases, the amount of emitted nitrogen oxides decreases (see Table 1) and thus aquatic acidification decreases. At a fuel equivalence ratio of about 1.2, the aquatic acidification becomes equal and at greater values of equivalence ratio, the ammonia fuel options have a lower environmental impact. A similar observation is made for terrestrial acidification/nutrification except the crossover point is at an equivalence ratio of about 1.25 (Figure 5). These results indicate that merely selecting the correct operating conditions of the burner can have a significant impact on the nitrogen footprint of the operation.
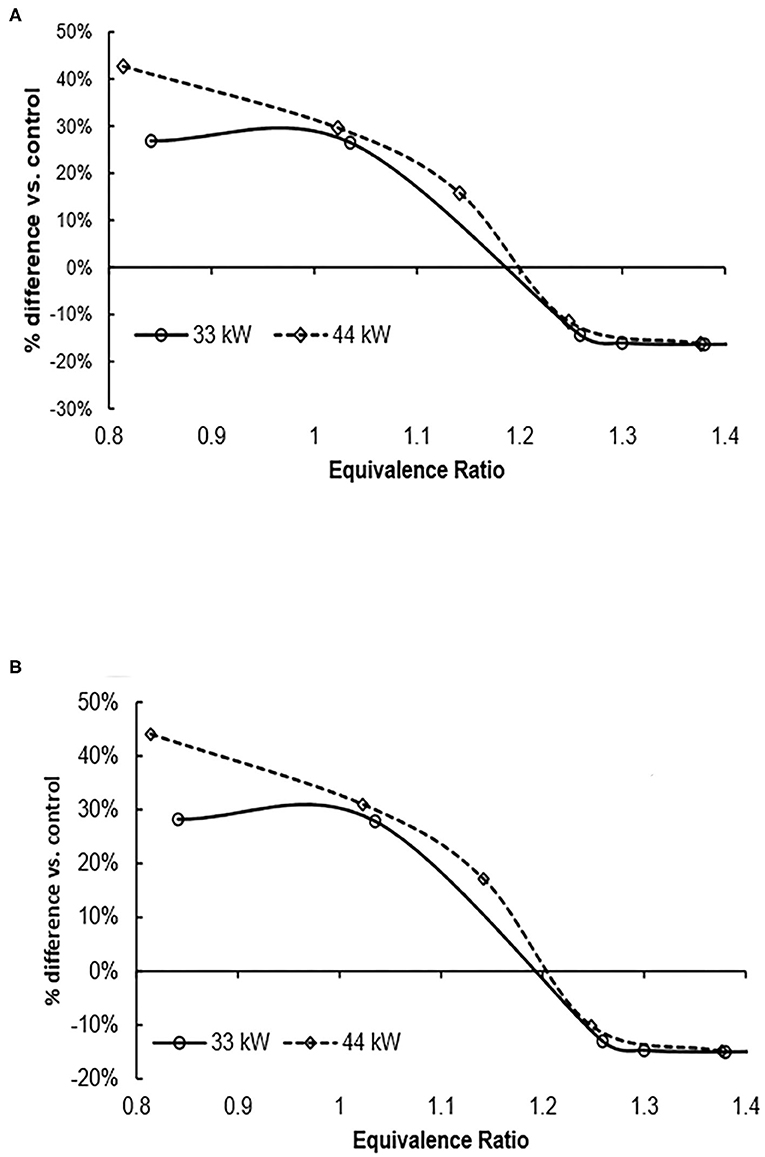
Figure 4. Aquatic acidification relative to control as fuel equivalence ratio increases. Use of ammonia results in lower aquatic acidification (negative percent difference) at equivalence ratio of about 1.2. (A) Wind power (B) nuclear power. The functional unit for this impact is 1 MJ of heat.
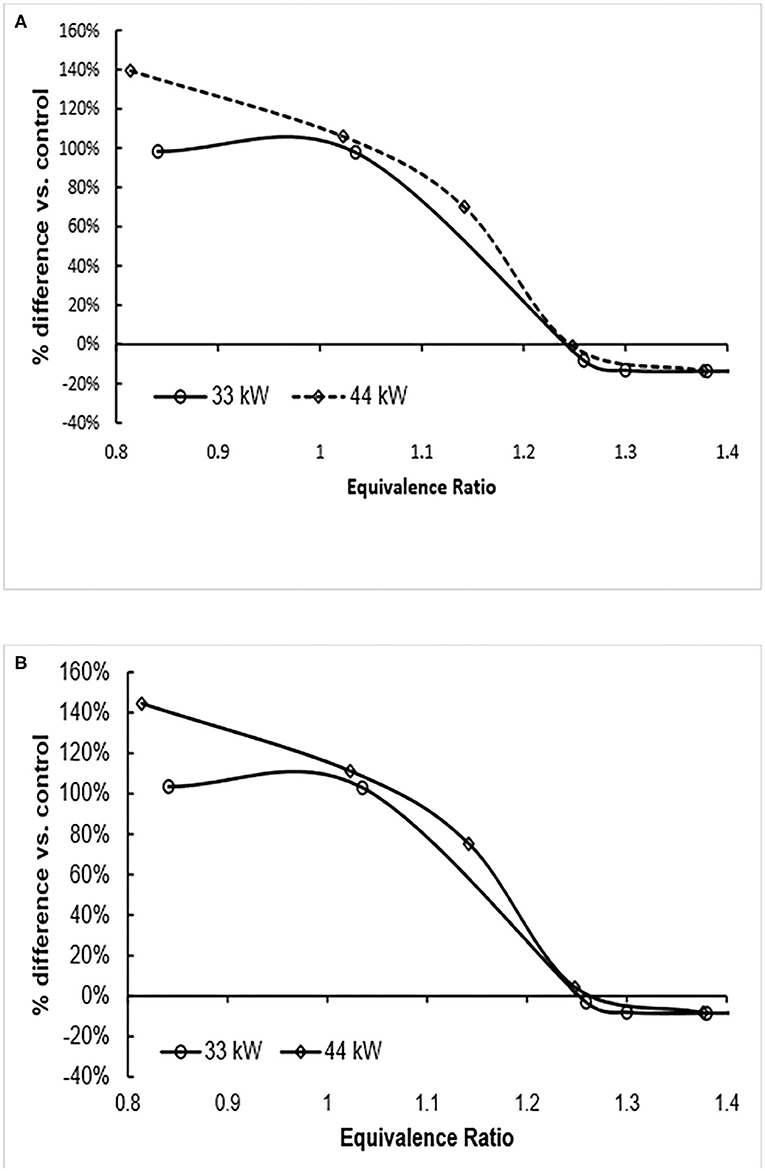
Figure 5. Terrestrial acidification/nutrification relative to control as fuel equivalence ratio increases. Use of ammonia results in lower aquatic acidification (negative percent difference) at equivalence ratio of about 1.25. (A) Wind power (B) nuclear power. The functional unit for this impact is 1 MJ of heat.
Conclusions
In sum, of the 14 scenarios resulting from combining seven ammonia and two methane sources, only two resulted in a lower global warming potential than the pure methane control. Both used biogas the methane source. One used wind power to generate electricity for a water-electrolysis ammonia production process while the other one used nuclear power. Both also had lower respiratory organics impact than the methane control. The higher GWP calculated from the scenarios that utilized natural gas and most of the biogas systems show the importance of the co-fuel that have had to be fed with ammonia in direct combustion systems. New burner systems may have to be designed to minimize the use of these co-fuels.
It is an encouraging result that operation at appropriate fuel equivalence ratios would result in lower environmental impact than the methane control. This is especially true if coupled with the finding of the influence of higher pressure (Khateeb et al., 2021) and the presence of water in the feed (Ariemma et al., 2020) on NOx emissions. One of the significant findings in previous studies (Angeles et al., 2018; Bicer and Dincer, 2018a) is that the nitrogen footprint is increased if ammonia is used. Thus, changing the combustion operating conditions could reverse this observation.
It is important to note that the burner that was analyzed did not have any NOx emission control systems. It would be interesting to analyze larger-scale burner systems that have associated NOx and carbon capture systems in future studies. While these may reduce the emissions impacts, there may again be trade-offs from building, operating and eventually disposing of the added NOx and carbon capture systems.
For the remaining eight environmental impacts, either wind power, nuclear power or both had worse environmental impacts than the methane control. The reason for these worse environmental impacts is inherent to the nature of wind and nuclear power. In the case of wind power, a considerable amount of resources needs to be used for building windmills. On the other hand, the ionizing radiation risk is inherent to nuclear power and considerable amounts of environmental impact are generated from the production of uranium fuel rods.
The choice between the use of nuclear and wind energy to produce ammonia may come down to the availability of large-scale wind resources and the socio-political acceptability of nuclear power. Many countries do not have access to wind power while other countries have adopted a nuclear-free policy. Ultimately, decision-makers will need to make the appropriate value judgements to distinguish which of these paths to follow. Social and economic considerations will eventually have to considered to determine the appropriate alternative. Evaluation of these alternatives is beyond the scope of this paper.
It is important to note that while the extant results are applicable to the specific burner system analyzed, it is fairly clear that current production methods for producing and using ammonia fuel will not be able to deliver a lower global warming potential than methane control. If ammonia from electrolysis is used, trade-offs inherent to the selected power generation process need to be addressed. Development of longer-lived renewable energy systems that use recyclable materials could greatly improve the environmental performance of these systems. For the use of ammonia as a fuel under the conditions tested, synthesis methods that are less energy intensive than current methods and can operate under ambient conditions need to be developed (Razon, 2018).
Data Availability Statement
The raw data supporting the conclusions of this article will be made available by the authors, without undue reservation.
Author Contributions
AV-M performed the combustion experiments and the initial data analysis. LR performed the life-cycle assessment. LR and AV-M wrote the manuscript. All authors contributed to the article and approved the submitted version.
Funding
De La Salle University thanks the Philippines Higher Education Research Network (PHERNet) of the Commission on Higher Education (CHEd) for a research grant. Pre Consultants generously provided the license of Simapro Faculty. Cardiff University gratefully acknowledges the support from the Welsh European Funding Office (WEFO) through its program Flexible Integrated Energy Systems (FLEXIS), project no. 80835.
Conflict of Interest
The authors declare that the research was conducted in the absence of any commercial or financial relationships that could be construed as a potential conflict of interest.
Acknowledgments
The authors thank Ms. Jainalyn Delantar for her excellent technical assistance. Also, they thank two reviewers for their constructive comments during the review process.
References
Acar, C., and Dincer, I. (2019). Review and evaluation of hydrogen production options for better environment. J. Clean. Prod. 218, 835–849. doi: 10.1016/j.jclepro.2019.02.046
Afif, A., Radenahmad, N., Cheok, Q., Shams, S., Kim, J. H., and Azad, A. K. (2016). Ammonia-fed fuel cells: a comprehensive review. Renew. Sustain. Energy Rev. 60, 822–835. doi: 10.1016/j.rser.2016.01.120
Althaus, H.-J., Chudacoff, M., Hischier, R., Jungbluth, N., Osses, M., and Primas, A. (2007). Life Cycle Inventories of Chemicals. ecoinvent report No. 8, v2.0. Paul Scherrer Institut Villigen, Swiss Centre for Life Cycle Inventories.
Angeles, D. A., Are, K. R. A. G., Aviso, K. B., Tan, R. R., and Razon, L. F. (2017). Optimization of the automotive ammonia fuel cycle Using P-graphs. ACS Sustain. Chem. Eng. 5, 8277–8283. doi: 10.1021/acssuschemeng.7b01940
Angeles, D. A., Tan, R. R., Aviso, K. B., Are, K. R. A. G., and Razon, L. F. (2018). Fuzzy optimization of the automotive ammonia fuel cycle. J. Clean. Prod. 186, 877–882. doi: 10.1016/j.jclepro.2018.03.143
Ariemma, G. B., Sabia, P., Sorrentino, G., Bozza, P., de Joannon, M., and Ragucci, R. (2020). Influence of water addition on MILD ammonia combustion performances and emissions. Proc. Combust. Inst. doi: 10.1016/j.proci.2020.06.143
Bicer, Y., and Dincer, I. (2018a). Life cycle assessment of ammonia utilization in city transportation and power generation. J. Clean. Prod. 170, 1594–1601. doi: 10.1016/j.jclepro.2017.09.243
Bicer, Y., and Dincer, I. (2018b). Life cycle environmental impact assessments and comparisons of alternative fuels for clean vehicles. Resour. Conserv. Recycl. 132, 141–157. doi: 10.1016/j.resconrec.2018.01.036
Bicer, Y., Dincer, I., Vezina, G., and Raso, F. (2017). Impact assessment and environmental evaluation of various ammonia production processes. Environ. Manag. 59, 842–855. doi: 10.1007/s00267-017-0831-6
Bicer, Y., and Khalid, F. (2018). Life cycle environmental impact comparison of solid oxide fuel cells fueled by natural gas, hydrogen, ammonia, and methanol for combined heat and power generation. Int. J. Hydrogen Energy 45, 3670–3685. doi: 10.1016/j.ijhydene.2018.11.122
Brown, T. (2020). Saudi Arabia to Export Renewable Energy Using Green Ammonia. Ammonia Energy Association. Available online at: https://www.ammoniaenergy.org/articles/saudi-arabia-to-export-renewable-energy-using-green-ammonia/ (accessed February 27, 2021).
Dones, R., Bauer, C., and Doka, G. (2007). Kernenergie. Sachbilanzen von Energiesystemen: Grundlagen fur den okologischen Verglecih von Energiesystemen un den Einbezug von Energiesystemen in Okobilanzen fur die Schweiz. Final report ecoinvent No. 6-V. Paul Scherrer Institut Villigen, Swiss Centre for Life Cycle Inventories.
EFMA (2007). Guidance for Transporting Ammonia by Rail. Brussels: European Fertilizer Manufacturers Association.
Faist Emmenegger, M., Heck, T., Jungbluth, N., and Tuchschmid, M. (2007). Erdgas. Sachbilanzen von Energiesystemen: Grundlagen fur den okologischen Verglecih von Energiesystemen un den Einbezug von Energiesystemen in Okobilanzen fur die Schweiz. Final report ecoinvent No. 6-V. Paul Scherrer Institut Villigen, Swiss Centre for Life Cycle Inventories.
Ferreira, J. A., Brancoli, P., Agnihotri, S., Bolton, K., and Taherzadeh, M. J. (2018). A review of integration strategies of lignocelluloses and other wastes in 1st generation bioethanol processes. Process Biochem. 75, 173–186. doi: 10.1016/j.procbio.2018.09.006
Guati-Rojo, A., Demski, C., and Valera-Medina, A. (2021). Beyond the technology: public perception of ammonia energy technologies, in Techno-Economic Challenges of Green Ammonia as an Energy Vector (Amsterdam: Elsevier), 277–302.
Jacobsen, D. (2020). A marine fuel standard for Ammonia–an engine designers perspective, in Ammonia Energy Association (Clayton, VIC). Available online at: https://www.ammoniaenergy.org/paper/a-marine-fuel-standard-for-ammonia-an-engine-designers-perspective/ (accessed February 26, 2021).
Jolliet, O., Margni, M., Charles, R., Humbert, S., Payet, J., Rebitzer, G., et al. (2003). IMPACT 2002+: a new life cycle impact assessment methodology. Int. J. Life Cycle Assess. 8, 324–330. doi: 10.1007/BF02978505
Jungbluth, N., Chudacoff, M., Dauriatt, A., Dinkel, F., Doka, G., Faist Emmenegger, M., et al. (2007). Life Cycle Inventories of Bioenergy. ecoinvent report No. 17. Swiss Centre for Life Cycle Inventories.
Khateeb, A. A., Guiberti, T. F., Wang, G., Boyette, W. R., Younes, M., Jamal, A., et al. (2021). Stability limits and NO emissions of premixed swirl ammonia-air flames enriched with hydrogen or methane at elevated pressures. Int. J. Hydrog. Energy 46, 11969–11981. doi: 10.1016/j.ijhydene.2021.01.036
Knothe, G., and Razon, L. F. (2017). Biodiesel fuels. Prog. Energy Combust. Sci. 58, 36–59. doi: 10.1016/j.pecs.2016.08.001
Kobayashi, H., Hayakawa, A., Somarathne, K. D. K. A., and Okafor, E. C. (2019). Science and technology of ammonia combustion. Proc. Combust. Inst. 37, 109–133. doi: 10.1016/j.proci.2018.09.029
Lee, K.-M., and Inaba, A. (2004). Life Cycle Assessment: Best Practices of International Organization for Standardization (ISO) 14040 Series. Comm. Trade Invest., 99. Available online at: http://publications.apec.org/publication-detail.php?pub_id=453 (accessed March 19, 2021).
Okafor, E. C., Somarathne, K. D. K. A., Ratthanan, R., Hayakawa, A., Kudo, T., Kurata, O., et al. (2020). Control of NOx and other emissions in micro gas turbine combustors fuelled with mixtures of methane and ammonia. Combust. Flame 211, 406–416. doi: 10.1016/j.combustflame.2019.10.012
Razon, L. F. (2012). Life cycle energy and greenhouse gas profile of a process for the production of ammonium sulfate from nitrogen-fixing photosynthetic cyanobacteria. Bioresour. Technol. 107, 339–346. doi: 10.1016/j.biortech.2011.12.075
Razon, L. F. (2014). Life cycle analysis of an alternative to the Haber-Bosch process: non-renewable energy usage and global warming potential of liquid ammonia from cyanobacteria. Environ. Prog. Sustain. Energy 33, 618–624. doi: 10.1002/ep.11817
Razon, L. F. (2018). Reactive nitrogen: a perspective on its global impact and prospects for its sustainable production. Sustain. Prod. Consum. 15, 35–48. doi: 10.1016/j.spc.2018.04.003
Reiter, A. J., and Kong, S. (2011). Combustion and emissions characteristics of compression-ignition engine using dual ammonia-diesel fuel. Fuel 90, 87–97. doi: 10.1016/j.fuel.2010.07.055
Simapro. Available online at: https://simapro.com/ (accessed February 22, 2021).
Sun, Y., Cai, T., and Zhao, D. (2021). Thermal performance and NOx emission characteristics studies on a premixed methane-ammonia-fueled micro-planar combustor. Fuel 291:120190. doi: 10.1016/j.fuel.2021.120190
Tunå, P., Hulteberg, C., and Ahlgren, S. (2014). Techno-economic assessment of nonfossil ammonia production. Environ. Prog. Sustain. Energy 33, 1290–1297. doi: 10.1002/ep.11886
Valera-Medina, A., Marsh, R., Runyon, J., Pugh, D., Beasley, P., Hughes, T., et al. (2017). Ammonia–methane combustion in tangential swirl burners for gas turbine power generation. Appl. Energy 185, 1362–1371. doi: 10.1016/j.apenergy.2016.02.073
Valera-Medina, A., Xiao, H., Owen-Jones, M., David, W. I. F., and Bowen, P. J. (2018). Ammonia for power. Prog. Energy Combust. Sci. 69, 63–102. doi: 10.1016/j.pecs.2018.07.001
Verhelst, S., Turner, J. W., Sileghem, L., and Vancoillie, J. (2019). Methanol as a fuel for internal combustion engines. Prog. Energy Combust. Sci. 70, 43–88. doi: 10.1016/j.pecs.2018.10.001
Yamamoto, A., Kimoto, M., Ozawa, Y., and Hara, S. (2018). Basic Co-Firing Characteristics of Ammonia with Pulverized Coal in a Single Burner Test Furnace. Available online at: https://aiche.confex.com/aiche/2018/meetingapp.cgi/Paper/539911 (accessed February 27, 2021).
Zamfirescu, C., and Dincer, I. (2008). Using ammonia as a sustainable fuel. J. Power Sour. 185, 459–465. doi: 10.1016/j.jpowsour.2008.02.097
Keywords: ammonia fuel, life cycle assessment, ammonia-to-power, power-to-ammonia, Haber-Bosch, renewable energy, nuclear power, wind power
Citation: Razon LF and Valera-Medina A (2021) A Comparative Environmental Life Cycle Assessment of the Combustion of Ammonia/Methane Fuels in a Tangential Swirl Burner. Front. Chem. Eng. 3:631397. doi: 10.3389/fceng.2021.631397
Received: 20 November 2020; Accepted: 09 March 2021;
Published: 07 April 2021.
Edited by:
Yusuf Bicer, Hamad Bin Khalifa University, QatarReviewed by:
Giancarlo Sorrentino, University of Naples Federico II, ItalyMehmet Akif Ezan, Dokuz Eylül University, Turkey
Copyright © 2021 Razon and Valera-Medina. This is an open-access article distributed under the terms of the Creative Commons Attribution License (CC BY). The use, distribution or reproduction in other forums is permitted, provided the original author(s) and the copyright owner(s) are credited and that the original publication in this journal is cited, in accordance with accepted academic practice. No use, distribution or reproduction is permitted which does not comply with these terms.
*Correspondence: Luis F. Razon, bHVpcy5yYXpvbiYjeDAwMDQwO2Rsc3UuZWR1LnBo; Agustin Valera-Medina, dmFsZXJhbWVkaW5hYTEmI3gwMDA0MDtjYXJkaWZmLmFjLnVr