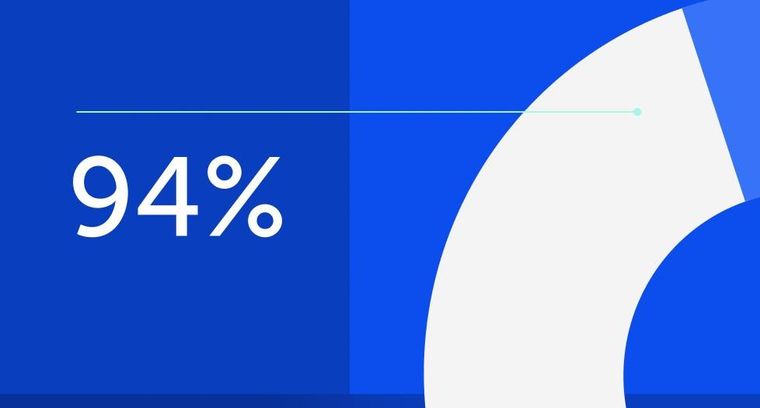
94% of researchers rate our articles as excellent or good
Learn more about the work of our research integrity team to safeguard the quality of each article we publish.
Find out more
MINI REVIEW article
Front. Chem. Eng., 22 December 2020
Sec. Catalytic Engineering
Volume 2 - 2020 | https://doi.org/10.3389/fceng.2020.604624
This article is part of the Research TopicBiomass Transformation to Chemicals, Fuels and MaterialsView all 6 articles
1,3-propanediol (1,3-PDO) is an important bulk chemical widely used in the polyester and polyurethane industry. The selective hydrogenolysis of glycerol to value-added 1,3-PDO is extremely attractive. However, the formation of 1,3-PDO is less thermodynamically stable than 1,2-PDO, and the steric hindrance effect in the reaction process makes the highly selective production of 1,3-PDO a great challenge. In this mini review, the recent research progress on the selective catalytic hydrogenolysis of glycerol to 1,3-PDO is overviewed and the catalytic mechanism of the reaction is summarized. We mainly focus on the different performances of each type of catalyst (Pt-W-based catalysts, Ir-Re based-catalysts, and other types) as well as the interactions between metals and supports. Finally, several personal perspectives on the opportunities and challenges within this promising field are discussed.
The utilization of renewable energy is the key to solving the current energy and environmental issues, and the conversion of renewable biomass to value-added chemicals is often discussed with this aim (Rao and Rathod, 2019). The catalytic conversion of glycerol, a byproduct of overcapacity from biodiesel manufacture, into value-added 1,3-PDO has shown high potential and has attracted much attention (Sun et al., 2016; Gerardy et al., 2018). 1,3-PDO is widely used in solvents, cosmetics, cleaning products, pharmaceutical industries, and organic synthesis intermediates (da Silva Ruy et al., 2020; Nakagawa et al., 2014; Lee et al., 2015; Kandasamy et al., 2019). It is a monomer of polyester and polyurethane and can be synthesized with terephthalic acid into a new polyester material, polytrimethylene terephthalate (PTT) (Sun et al., 2016; Gerardy et al., 2018; da Silva Ruy et al., 2020; Nakagawa et al., 2014). Currently, the conventional processes to produce 1,3-PDO mainly utilize the hydration of acrolein and the hydroformylation of ethylene oxide, which are both from unrenewable petroleum-based routes. At present, many strategies have been reported to produce 1,3-PDO from bioglycerol (Lee et al., 2015). Hydrogenolysis of glycerol over heterogeneous catalysts in aqueous solution shows great potential because it is both environmentally friendly and scalable for industrial processes.
Glycerol hydrogenolysis is a process in which the C-OH bond in glycerol is broken and the OH group is replaced by hydrogen to produce propylene glycol. The secondary hydroxyl group in glycerol is replaced by hydrogen to produce 1,3-PDO, and the primary hydroxyl group is replaced by hydrogen to produce 1,2-propanediol (1,2-PDO) (Kandasamy et al., 2019). Since propylene glycol also contains C-OH bonds, hydrogenolysis will continue during the reaction to produce n-propanol (n-PO), isopropanol (i-PO), propane, and other products (Figure 1) (Mizugaki and Kaneda, 2019). In this reaction, the key procedure to enhance the selectivity of 1,3-PDO is to accurately control the activation and cleavage of secondary C-O bonds while preventing further hydrogenolysis of the target product. Therefore, it is necessary to design specific active sites to target, anchor, and activate the secondary hydroxyl group of glycerol for hydrogenolysis. In this regard, there is an urgent need to develop efficient and selective catalysts, and a better understanding of the catalytic mechanism is essential for catalyst design. In addition to the catalyst, the reaction conditions are also important factors for the hydrogenolysis of glycerol.
FIGURE 1. Possible reactions and products from hydrogenolysis of glycerol over a heterogeneous catalyst.
At present, most reviews described in detail the reaction mechanism and chemical route of glycerol hydrogenolysis, but there are few reviews that focus on the refined classification of catalysts in the reaction of glycerol hydrogenolysis to 1,3-PDO (Rao and Rathod, 2019; Sun et al., 2016; Gerardy et al., 2018; da Silva Ruy et al., 2020; Nakagawa et al., 2014; Lee et al., 2015; Kandasamy et al., 2019; Mizugaki and Kaneda, 2019; Nakagawa & Tomishige, 2011; Tong et al., 2017). In this paper, the main catalytic reaction mechanisms on selective hydrogenolysis of glycerol to 1,3-PDO are summarized and discussed. Then different types of catalysts including Pt-W-based catalysts, Ir-Re-based catalysts, and other types of catalysts as well as various catalytic systems for this reaction in recent years are compared and overviewed, with focus on the different performance between each type of catalyst as well as the interactions between metals and supports. Finally, several personal perspectives on the opportunities and challenges aimed at industrial application are provided.
Several reaction mechanisms for the selective hydrogenolysis of glycerol to 1,3-PDO have been proposed based on different product distributions, active catalytic components, and reaction conditions. The two-step mechanism (dehydration- hydrogenation) has been proposed in many papers for glycerol hydrogenolysis: first, glycerol is dehydrated to form intermediates of 3-hydroxypropanal (3-PAH), followed by the rapid hydrogenation of 3-PAH to form 1,3-PDO, and the rearrangement produces propionic acid (PA) (Nakagawa & Tomishige, 2011; Tong et al., 2017). Dehydration of the primary hydroxyl group of glycerol will generate acetol intermediates, which will be further hydrogenated to obtain 1,2-PDO (Figure 2A). Edake et al. (Edake et al., 2017) put forward more detailed insights on this basis. They believed that glycerol may first undergo double dehydration to produce acrolein, which is then rehydrated to form the above intermediate 3-PAH, followed by the hydrogenation to 1,3-PDO.
FIGURE 2. (A) Dehydration-hydrogenation mechanism of glycerol hydrogenolysis; (B) different types of hydrogen species on the surface of the Pt/WOx/ZrO2 catalyst.
Studies have shown that acid properties and hydrogen species play an important role in the hydrogenolysis of glycerol to 1,3-PDO (Zhou et al., 2019). For Pt-W series catalysts, it was reported that glycerol is firstly dehydrated to form a secondary carbocation. Subsequently, the secondary carbocation will be attacked by a hydride and rapidly hydrogenated to produce 1,3-PDO, this hydride is derived from the dissociation of H2 at the noble metal (Pt) site (Feng et al., 2017; García-Fernández et al., 2017). For the effect of this hydride, Zhou (Zhou et al., 2019) studied the different types of surface hydrogen species for the hydrogenolysis of glycerol using a Pt/WOx/ZrO2 catalyst, and five different types of surface hydrogen species was described (shown in Figure 2B). During the catalytic reaction, H2 molecules were first adsorbed at the Pt site and dissociated into hydrogen atoms. PtOx was then reduced to Pt metal, and the dissociated hydrogen atoms spilled over to the adjacent WOx site, which reduced part of W6+ to W5+. The formation of W5+ significantly accelerated the hydrogenation process of intermediates, remarkably improving the selectivity of 1,3-PDO.
Catalysts play an extremely important role in the selective hydrogenolysis of glycerol to 1,3-PDO. The most commonly used catalyst is the bifunctional material composed of hydrogen-activated metal and acidic sites, typically Pt-W-based and Ir-Re-based catalysts. In addition, other catalytic systems were also tried, such as metal-supported molecular sieves, modified heteropoly acids, and oxyphilic metal oxide. The highest yield of 1,3-PDO in the current reported studies has reached 66% (Arundhathi et al., 2013), but the yield of 1,3-PDO has not yet had a breakthrough up to now.
In a Pt-W-based catalyst, metal acts as the hydrogen activation center of the reaction, accumulating and releasing hydrogen atoms continuously (Wang et al., 2019; Zhao et al., 2019; Pan et al., 2019; Zhou et al., 2016). Hydrogen dissociates into two hydrogen atoms on the metal sites, these hydrogen atoms overflow onto the support, one of the hydrogen atoms releases an electron to form H+, which interacts with another hydrogen atom on the tungsten oxide to generate H-in situ, forming an acid site on the catalyst (Figure 1A). As a deoxygenation active center for glycerol hydrogenolysis, W provides a large number of acid sites, in which there are oxygen vacancies in the defective tungsten oxide, and a large amount of Brønsted acid can be generated in the reaction. It has been verified by experiments that the combination of Pt and W exhibits outstanding activity and selectivity compared with other metals (Wang et al., 2020). Owing to the synergistic effect between the hydrogenation active center of Pt and the strong Brønsted acid sites of WOx, Pt-W-based bifunctional catalysts are relatively efficient for selective hydrogenolysis of glycerol to 1,3-PDO.
Aihara and collaborators believed that the yield of 1,3-PDO in hydrogenolysis of glycerol was positively related to the length of the W-Al perimeter interface on the Pt/WO3/Al2O3 catalyst, while a W–(OH)–Al site on the W–Al interface functioned as a main active center (Aihara et al., 2019). Since then, they have conducted in-depth research on the hydrogenation of various polyols and ethers over Pt/WO3/Al2O3 catalysts and found that the hydrogenolysis rate of a C-O bond at a secondary carbon was higher than that at a primary carbon, suggesting that the hydrogenation reaction with secondary carbocation as an intermediate takes precedence over that with primary carbocation (Aihara et al., 2020). During the catalytic reaction, the surface density of the W species, the number and strength of the Brønsted acid sites, as well as the interaction between Pt-W are the key factors to the selectivity of 1,3-PDO (Garcia-Fernandez et al., 2017). Using a Pt/WOx/ZrO2 catalyst, the Zhu group (Zhu et al., 2014) found that when the ZrO2 support was transformed from the monoclinic phase to tetragonal nanoparticles, it could inhibit the lateral growth of WOx, promote the dispersion of WOx on the carrier, and significantly improve the acidity of the catalyst and the selectivity to 1,3-PDO. The results have also been confirmed by Fan et al. (Fan et al., 2017). The aforementioned changes in the crystalline phase of materials result in a great difference in catalytic performance.
In addition, most researchers added metal oxides to the Pt/W catalyst (Wang et al., 2015). Metal oxides can not only be used as catalytic support to promote the dispersion of active components, but can also adjust the acidity of the catalyst and increase the yield of 1,3-PDO (García-Fernández et al., 2015; Wang et al., 2016; Shi et al., 2018; Liu et al., 2020).
Molecular sieves combined with Pt-W active components were also attempted (Shi et al., 2018). Priya and his group (Priya et al., 2015) first used the hydrothermally stable molecular sieve SBA-15 as a carrier to support Pt-W to prepare a Pt-W-SBA15 catalyst. It is believed that in Pt-W-based catalysts, the selectivity of 1,3-PDO is positively correlated with the concentration of the Brønsted acid site (Sun et al., 2016; da Silva Ruy et al., 2020; Nakagawa et al., 2014; Lee et al., 2015; Kandasamy et al., 2019; Mizugaki and Kaneda, 2019; Nakagawa and Tomishige, 2011). However, there is an exception, Fan et al. (Fan et al., 2017) prepared a Pt NPS-W-SBA15 catalyst with the tetrahedral phase WO4, which only showed Lewis acidity, but the selectivity of 1,3-PDO was up by 70.8%. The discrepancy of the result indicates that the reaction mechanism still needs further improvement.
In addition, doping with specific metals also has positive effects on the selectivity of 1,3-PDO, which can strengthen the interaction between Pt and W metals, increase electron density, and improve acidity (Wang et al., 2016; Zhao et al., 2017). The Yang group (Yang et al., 2018) doped the Nb element onto Pt/WOx material, improving the H2 tolerance of the catalyst and inhibiting the over-reduction of active WOx under high hydrogen pressure. Subsequently, they tried to replace Nb with Au. The addition of Au led to the partial substitution of W6+ by Au3+, which changed the electronic structure and properties of WO3 and increased the electron density of the active metal (Yang et al., 2018). Feng et al. (Feng et al., 2019) introduced the Al element into the Pt-W-SBA-15 catalyst, which changed the ratio of Lewis acid and Brønsted acid, thereby affecting the product distribution. We have summarized the catalytic performance of typical Pt-W-based catalysts for the hydrogenolysis of glycerol in batch reactors, as shown in Table 1.
Unlike the Pt-W-based catalytic system, the active sites in the Ir-Re-based catalytic system are on the Ir-Re interface. The hydride produced by hydrogen dissociation can attack the C-O bond in the substrate, and the OH groups at both ends of the substrate are anchored by Re sites, exposing the adjacent C-O bond to potential attack and cracking (Nakagawa et al., 2018). The Ir-Re-based catalysts usually show moderate conversion of glycerol but higher selectivity to 1,3-PDO than the Pt-W-based catalysts, as shown in Table 1. A very distinct feature of the Ir-Re-based catalytic system is that the reaction temperature is lower (120°C) owing to the high activity of the Ir-Re-based catalyst. This high activity for C-O bond cleavage can be attributed to the synergistic effect between the Ir and ReOx species. However, the high activity of the Ir-Re catalyst may reduce the stability to a certain extent, which makes it easier for the catalyst to be poisoned.
The Ir-Re-based catalyst (Ir-ReOx/SiO2) for glycerol hydrogenolysis was firstly reported by the Tomishige team (Nakagawa et al., 2010), who further improved the catalytic performance by adding a variety of acid promoters such as H2SO4, Amberlyst 70, and H-ZSM-5. The role of the co-catalyst was to accelerate the protonation of the ReOx cluster on the surface of the catalyst, thereby increasing the sites for adsorbing terminal hydroxyl groups to enhance the stability and activity of the catalyst (Nakagawa et al., 2012). In order to further increase the yield of 1,3-PDO, they prepared an Ir-ReOx/rutile catalyst using rutile titanium dioxide as a support and achieved high productivity of 1,3-PDO (52g gIr−1 h−1) (Liu et al., 2019).
For Ir-Re-based catalysts, there is a significant synergistic effect between Ir- and Re-species (Deng et al., 2015; Varghese et al., 2018). Deng et al. (Deng et al., 2015) proposed that on the surface of Ir-Re alloy particles, Re can activate water molecules to form the (Ir)-Re-OH species, which are adsorption sites of glycerol, resulting in the high activity of the Ir-Re alloy for glycerol hydrogenolysis. The Luo group (Luo et al., 2016) prepared a shell Ir-ReOx catalyst. The special morphology shortened the diffusion distance and accelerated the thermal diffusion on the surface, which inhibited the excessive hydrogenolysis of 1,3-PDO and thus improved the selectivity. In addition, the molar ratio of the Ir and Re metals (Liu et al., 2019) and the adjustment of the impregnation order (Luo et al., 2016) on the support make the catalytic performance remarkably different. The catalytic performance of typical Ir-Re-based catalysts for the hydrogenolysis of glycerol in batch reactors is summarized in Table 1.
Compared with Pt-W-based catalysts, Ir-Re-based catalysts are also employed in the cleavage of C-O bonds in other biomass platform molecules, for example 1,2-hexanediol, tetrahydrofurfuryl alcohol, and cyclohexanediol (Tomishige et al., 2014). The active sites at the Ir-ReOx interface can anchor the terminal hydroxyl group of substrates, causing the neighboring C-O bond to be attacked and cleaved. Therefore, Ir-Re-based catalysts show unique advantages in the selective cleavage of C-O bonds of various platform molecules.
In addition to the two main series of catalysts above, many other catalysts were also reported, such as metal-supported molecular sieves, modified heteropoly acids, oxyphilic metal oxide, and so on.
A molecular sieve is a material with a large surface area, high porosity, and strong adsorption capacity. Priya et al. (Priya et al., 2016) prepared a highly efficient catalyst of H-Mordenite to support Pt, with a porous system and a large number of acid sites, that obtained a high glycerol conversion (94.9%) and a selectivity of 1,3-PDO (48.6%). Subsequently, they added Cu to the catalyst to synthesize a bimetallic catalyst (Priya et al., 2016). Although the selectivity of 1,3-PDO was improved, the stability and reusability of the catalyst needed to be improved. They also tried natural clay montmorillonite activated by sulfuric acid for glycerol hydrogenolysis (Samudrala et al., 2018). Wan et al. (Wan et al., 2019) prepared an Ir-ZSM5 catalyst by combining a HZSM-5 molecular sieve with Ir nanoclusters and achieved a good result. In addition to Pt and Ir, cheap transition metal-supported H-beta zeolite (Zr-Ni/H-beta) was also employed and achieved an acceptable result (Kant et al., 2017).
Heteropoly acid has both an acid base and oxidizing properties, but its specific surface area is relatively low. Therefore, alkaline cation modification is used to adjust the pH or modify the specific surface area. Mai et al. (Mai & Ng, 2017) exchanged the basic cation Cs+ to catalyst 10Ni-30H4SiW /Al2O3. With the increase of the Cs+ content, the selectivity of 1,3-PDO and 1-PO increased first and then decreased. In recent studies, heteropoly acids have also been added to materials as acid adjuvants. Cai et al. (Cai et al., 2017) added heteropoly acid (HSiW, HPW, HPMo) to a Co-Al catalyst to investigate the influence of reaction variables such as solvent, reaction temperature, and feeding speed in the hydrogenolysis of glycerol.
Due to their great affinity with non-metallic elements, oxyphilic metals such as Al, Si, Zr, and Ti easily form stable oxides and easily undergo electron transfer in the reaction. In addition to the combination of the above Pt-W series catalysts, oxyphilic metal oxides were also employed in the hydrogenolysis of glycerol, usually combined with other transition metals. Soares et al. (Soares et al., 2016) synthesized a Pt-Fe/Al2O3 bimetallic catalyst by supporting the metals Pt and Fe on Al2O3 and applied it to glycerol hydrogenolysis and the reforming reaction. In the hydrogenolysis process, it promoted the selectivity of 1,2-PDO and n-PO, and showed high activity. Ahmed and his collaborators (Ahmed et al., 2016) used Al2O3 as a carrier to support Ru, and fluorinated it with AlF3. The fluorination reaction had a significant effect on the physicochemical properties of the catalyst such as specific surface area, Ru dispersion, total pore volume, and average pore size.
In the abovementioned reaction systems, most of the reactions are carried out in batch reactors. In addition, there were many attempts in flow systems carried out in fixed-bed reactors. The flow system can shorten the residence time of the substrate and product over the catalyst, and prevent excessive hydrogenolysis of the target product, thereby increasing the selectivity of 1,3-PDO. Here we summarized the catalytic performances of glycerol hydrogenolysis in fixed-bed reactions, as shown in Table 2.
In this mini review, the research progress of selective hydrogenolysis of glycerol to 1,3-PDO was overviewed, the mechanism of the reaction and typical types of catalysts were comprehensively summarized. The hydrogenolysis of glycerol is a consecutive reaction, during which a number of products can still undergo hydrogenolysis. Increasing the reaction temperature will accelerate the rate of C-O bond cleavage to improve the conversion of glycerol, but this comes at the expense of a slight decrease in 1,3-PDO selectivity. Raising the hydrogen pressure can slightly increase the selectivity of 1,3-PDO, which also carries the risk of excessive hydrogenolysis. To improve the conversion and selectivity, controlling both reaction steps is crucial: the selective hydrogenolysis of the secondary hydroxyl group of glycerol and the inhibition of excessive hydrogenolysis of 1,3-PDO. Therefore, the design of the current catalysts must be combined with the control of these two steps, to improve the selectivity of 1,3-PDO. Pt-W-based and Ir-Re-based catalysts have shown great potential for 1,3-PDO production from hydrogenolysis of glycerol but still need improvements for practical application, the combination of these two types of catalyst might be a good solution. Moreover, from the viewpoint of industrial application, the efficiency, stability, and cost of the catalyst must all be considered and anticipated. The catalytic reaction is ideally conducted in a fixed-bed continuous reactor with high concentration and high time-space velocity.
JC, QX, YW, and YH discussed the topic and wrote the manuscript together.
This work was supported financially by the NSFC of China (No. 21972056 and 21603072).
The authors declare that the research was conducted in the absence of any commercial or financial relationships that could be construed as a potential conflict of interest.
Ahmed, T. S., Abdelaziz, O. Y., and Roberts, G. W. (2016). Preparation of Al2O3/AlF3-supported ruthenium catalysts for the hydrogenolysis of biodiesel-derived crude glycerol. Ind. Eng. Chem. Res. 55, 5536–5544. doi:10.1021/acs.iecr.6b00500
Aihara, T., Miura, H., and Shishido, T. (2019). Effect of perimeter interface length between 2D WO3 monolayer domain and γ-Al2O3 on selective hydrogenolysis of glycerol to 1,3-propanediol. Catal. Sci. Technol. 9, 5359–5367. doi:10.1039/C9CY01385G
Aihara, T., Miura, H., and Shishido, T. (2020). Investigation of the mechanism of the selective hydrogenolysis of C O bonds over a Pt/WO3/Al2O3 catalyst. Catal. Today 352, 73–79. 10.1016/j.cattod.2019.10.008.
Pan, C., Tan, G. A., Ge, L., Chen, C. L., and Wang, J. Y. (2019). Two-stage microbial conversion of crude glycerol to 1,3-propanediol and polyhydroxyalkanoates after pretreatment. J. environ. manage. 232 615–624. doi:10.1016/j.jenvman.2018.11.118
Cai, F., Song, X., Wu, Y., Zhang, J., and Xiao, G. (2017). Selective hydrogenolysis of glycerol over acid-modified Co-Al catalysts in a fixed-bed flow reactor. ACS Sustain. Chem. Eng. 6, 110–118. doi:10.1021/acssuschemeng.7b01233
da Silva Ruy, A. D., de Brito Alves, R. M., Reis Hewer, T. L., de Aguiar Pontes, D., Gomes Teixeira, L. S., and Magalhães Pontes, L. A. (Forthcoming 2020). Catalysts for glycerol hydrogenolysis to 1,3-propanediol: a review of chemical routes and market. Catal. Today. doi:10.1016/j.cattod.2020.06.035
Deng, C., Duan, X., Zhou, J., Zhou, X., Yuan, W., and Scott, S. L. (2015). Ir-Re alloy as a highly active catalyst for the hydrogenolysis of glycerol to 1,3-propanediol. Catal. Sci. Technol. 5, 1540–1547. doi:10.1039/C4CY01285B
Deng, C., Leng, L., Duan, X., Zhou, J., Zhou, X., and Yuan, W. (2015). Support effect on the bimetallic structure of Ir-Re catalysts and their performances in glycerol hydrogenolysis. J. Mol. Catal. Chem. 410, 81–88. doi:10.1016/j.molcata.2015.09.009
Edake, M., Dalil, M., Darabi Mahboub, M. J., Dubois, J.-L., and Patience, G. S. (2017). Catalytic glycerol hydrogenolysis to 1,3-propanediol in a gas-solid fluidized bed. RSC Adv. 7, 3853–3860. doi:10.1039/C6RA27248G
Fan, Y., Cheng, S., Wang, H., Tian, J., Xie, S., Pei, Y., et al. (2017). Pt-WOx on monoclinic or tetrahedral ZrO2: crystal phase effect of zirconia on glycerol hydrogenolysis to 1,3-propanediol. Appl. Catal. B-Environ. 217, 331–341. doi:10.1016/j.apcatb.2017.06.011
Fan, Y., Cheng, S., Wang, H., Ye, D., Xie, S., Pei, Y., et al. (2017). Nanoparticulate Pt on mesoporous SBA-15 doped with extremely low amount of W as a highly selective catalyst for glycerol hydrogenolysis to 1,3-propanediol. Green Chem. 19, 2174–2183. doi:10.1039/C7GC00317J
Feng, S., Zhao, B., Liang, Y., Liu, L., and Dong, J. (2019). Improving selectivity to 1,3-propanediol for glycerol hydrogenolysis using W- and Al-incorporated SBA-15 as support for Pt nanoparticles. Ind. Eng. Chem. Res. 58, 2661–2671. doi:10.1021/acs.iecr.8b03982
Feng, S., Zhao, B., Liu, L., and Dong, J. (2017). Platinum supported on WO3-doped aluminosilicate: a highly efficient catalyst for selective hydrogenolysis of glycerol to 1,3-propanediol. Ind. Eng. Chem. Res. 56, 11065–11074. doi:10.1021/acs.iecr.7b02951
García-Fernández, S., Gandarias, I., Requies, J., Güemez, M. B., Bennici, S., Auroux, A., et al. (2015). New approaches to the Pt/WOx/Al2O3 catalytic system behavior for the selective glycerol hydrogenolysis to 1,3-propanediol. J. Catal. 323, 65–75. doi:10.1016/j.jcat.2014.12.028
García-Fernández, S., Gandarias, I., Requies, J., Soulimani, F., Arias, P. L., and Weckhuysen, B. M. (2017). The role of tungsten oxide in the selective hydrogenolysis of glycerol to 1,3-propanediol over Pt/WOx/Al2O3. Appl. Catal. B-Environ. 204, 260–272. doi:10.1016/j.apcatb.2016.11.016
Garcia-Fernandez, S., Gandarias, I., Tejido-Nunez, Y., Requies, J., and Arias, P. L. (2017). Influence of the support of bimetallic platinum tungstate catalysts on 1,3-propanediol formation from glycerol. Chemcatchem 9, 4508–4519. doi:10.1002/cctc.201701067
Gerardy, R., Morodo, R., Estager, J., Luis, P., Debecker, D. P., and Monbaliu, J. M. (2018). Sustaining the transition from a petrobased to a biobased chemical industry with flow chemistry. Top. Curr. Chem. 377, 1. doi:10.1007/s41061-018-0222-3
Wang, J., Zhao, X., Lei, N., Li, L., Zhang, L., Xu, S., et al. (2016). Hydrogenolysis of glycerol to 1,3-propanediol under low hydrogen pressure over WOx-supported single/pseudo-single atom Pt catalyst. ChemSusChem 9 784–790. doi:10.1002/cssc.201501506
Tomishige, K., Tamura, M., and Nakagawa, Y. (2014). Role of Re species and acid cocatalyst on Ir-ReOx /SiO2 in the C-O hydrogenolysis of biomass-derived substrates, Chem. Rec. 14, 1041–1054. doi:10.1002/tcr.201402026
Kandasamy, S., Samudrala, S. P., and Bhattacharya, S. (2019). The route towards sustainable production of ethylene glycol from a renewable resource, biodiesel waste: a review. Catal. Sci. Technol. 9, 567–577. doi:10.1039/C8CY02035C
Kant, A., He, Y., Jawad, A., Li, X., Rezaei, F., Smith, J. D., et al. (2017). Hydrogenolysis of glycerol over Ni, Cu, Zn, and Zr supported on H-beta. Chem. Eng. J. 317, 1–8. doi:10.1016/j.cej.2017.02.064
Lee, C. S., Aroua, M. K., Daud, W. M. A. W., Cognet, P., Pérès-Lucchese, Y., Fabre, P. L., et al. (2015). A review: conversion of bioglycerol into 1,3-propanediol via biological and chemical method. Renew. Sustain. Energy Rev. 42, 963–972. doi:10.1016/j.rser.2014.10.033
Liu, L., Asano, T., Nakagawa, Y., Tamura, M., Okumura, K., and Tomishige, K. (2019). Selective hydrogenolysis of glycerol to 1,3-propanediol over rhenium-oxide-modified iridium nanoparticles coating rutile titania support. ACS Catal. 9, 10913–10930. doi:10.1021/acscatal.9b03824
Liu, L., Asano, T., Nakagawa, Y., Tamura, M., and Tomishige, K. (2020). One-pot synthesis of 1,3-butanediol by 1,4-anhydroerythritol hydrogenolysis over a tungsten-modified platinum on silica catalyst. Green Chem. 22, 2375–2380. doi:10.1039/D0GC00244E
Liu, L., Kawakami, S., Nakagawa, Y., Tamura, M., and Tomishige, K. (2019). Highly active iridium-rhenium catalyst condensed on silica support for hydrogenolysis of glycerol to 1,3-propanediol. Appl. Catal. B-Environ. 256, 117775. doi:10.1016/j.apcatb.2019.117775
Luo, W., Lyu, Y., Gong, L., Du, H., Jiang, M., and Ding, Y. (2016). The influence of impregnation sequence on glycerol hydrogenolysis over iridium-rhenium catalyst. React. Kinet. Mech. Catal. 118, 481–496. doi:10.1007/s11144-016-0975-z
Luo, W., Lyu, Y., Gong, L., Du, H., Wang, T., and Ding, Y. (2016). Selective hydrogenolysis of glycerol to 1,3-propanediol over egg-shell type Ir-ReOx catalysts. RSC Adv. 6, 13600–13608. doi:10.1039/C5RA24808F
Mai, C. T. Q., and Ng, F. T. T. (2017). Effect of Cs+ on the hydrogenolysis of glycerol to higher value sustainable and green chemicals using a supported Ni-HSiW catalyst. Catal. Today 291, 195–203. doi:10.1016/j.cattod.2017.02.043
Mizugaki, T., and Kaneda, K. (2019). Development of high performance heterogeneous catalysts for selective cleavage of C-O and C-C bonds of biomass-derived oxygenates. Chem. Rec. 19, 1179–1198. doi:10.1002/tcr.201800075
Nakagawa, Y., Ning, X., Amada, Y., and Tomishige, K. (2012). Solid acid co-catalyst for the hydrogenolysis of glycerol to 1,3-propanediol over Ir-ReOx/SiO2. Appl. Catal. Gen. 433-434, 128–134. doi:10.1016/j.apcata.2012.05.009
Nakagawa, Y., Shinmi, Y., Koso, S., and Tomishige, K. (2010). Direct hydrogenolysis of glycerol into 1,3-propanediol over rhenium-modified iridium catalyst. J. Catal. 272, 191–194. doi:10.1016/j.jcat.2010.04.009
Nakagawa, Y., Tamura, M., and Tomishige, K. (2014). Catalytic materials for the hydrogenolysis of glycerol to 1,3-propanediol. J. Mater. Chem. A 2, 6688–6702. doi:10.1039/C3TA15384C
Nakagawa, Y., Tamura, M., and Tomishige, K. (2018). Perspective on catalyst development for glycerol reduction to C3 chemicals with molecular hydrogen. Res. Chem. Intermed. 44, 3879–3903. doi:10.1007/s11164-018-3481-2
Nakagawa, Y., and Tomishige, K. (2011). Heterogeneous catalysis of the glycerol hydrogenolysis. Catal. Sci. Technol. 1, 179–190. doi:10.1039/C0CY00054J
Niu, Y., Zhao, B., Liang, Y., Liu, L., and Dong, J. (2020). Promoting role of oxygen deficiency on a WO3-supported Pt catalyst for glycerol hydrogenolysis to 1,3-propanediol. Ind. Eng. Chem. Res. 59, 7389–7397. doi:10.1021/acs.iecr.9b07067
Priya, S. S., Bhanuchander, P., Kumar, V. P., Bhargava, S. K., and Chary, K. V. R. (2016). Activity and selectivity of platinum-copper bimetallic catalysts supported on mordenite for glycerol hydrogenolysis to 1,3-propanediol. Ind. Eng. Chem. Res. 55, 4461–4472. doi:10.1021/acs.iecr.6b00161
Priya, S. S., Bhanuchander, P., Kumar, V. P., Dumbre, D. K., Periasamy, S. R., Bhargava, S. K., et al. (2016). Platinum supported on H-mordenite: a highly efficient catalyst for selective hydrogenolysis of glycerol to 1,3-propanediol. ACS Sustain. Chem. Eng. 4, 1212–1222. doi:10.1021/acssuschemeng.5b01272
Priya, S. S., Kumar, V. P., Kantam, M. L., Bhargava, S. K., Srikanth, A., and Chary, K. V. R. (2015). High efficiency conversion of glycerol to 1,3-propanediol using a novel platinum-tungsten catalyst supported on SBA-15. Ind. Eng. Chem. Res. 54, 9104–9115. doi:10.1021/acs.iecr.5b01814
Arundhathi, R., Mizugaki, T., Mitsudome, T., Jitsukawa, K., and Kaneda, K. (2013). Highly selective hydrogenolysis of glycerol to 1,3-propanediol over a boehmite-supported platinum/tungsten catalyst, ChemSusChem 6, 1345–1347. doi:10.1002/cssc.201300196
Rao, P., and Rathod, V. (2019). Valorization of food and agricultural waste: a step towards greener future. Chem. Rec. 19, 1858–1871. doi:10.1002/tcr.201800094
Shi, G., Cao, Z., Xu, J., Jin, K., Bao, Y., and Xu, S. (2018). Effect of WOx doping into Pt/SiO2 catalysts for glycerol hydrogenolysis to 1,3-propanediol in liquid phase. Catal. Lett. 148, 2304–2314. doi:10.1007/s10562-018-2464-7
Shi, G., Xu, J., Song, Z., Cao, Z., Jin, K., Xu, S., et al. (2018). Selective hydrogenolysis of glycerol to 1,3-propanediol over Pt-WOx/SAPO-34 catalysts. Mol. Catal. 456, 22–30. doi:10.1016/j.mcat.2018.06.018
Soares, A. V.-H., Perez, G., and Passos, F. B. (2016). Alumina supported bimetallic Pt-Fe catalysts applied to glycerol hydrogenolysis and aqueous phase reforming. Appl. Catal. B-Environ. 185, 77–87. doi:10.1016/j.apcatb.2015.11.003
Samudrala, S. P., Kandasamy, S., and Bhattacharya, S. (2018). Turning biodiesel waste glycerol into 1,3-propanediol: catalytic performance of sulphuric acid-activated montmorillonite supported platinum catalysts in glycerol hydrogenolysis, Sci. Rep. 8 , 7484. doi:10.1038/s41598-018-25787-w
Sun, D., Yamada, Y., Sato, S., and Ueda, W. (2016). Glycerol hydrogenolysis into useful C3 chemicals. Appl. Catal. B-Environ. 193, 75–92. doi:10.1016/j.apcatb.2016.04.013
Tong, Q., Gao, Q., Xu, B., Yu, L., and Fan, Y. (2017). Pt/WO3/ZrO2-Catalyzed selective hydrogenolysis of glycerol to produce 1,3-propanediol. Chin. J. Org. Chem. 37, 753–758. doi:10.1002/cctc.201600981
Varghese, J. J., Cao, L., Robertson, C., Yang, Y., Gladden, L. F., Lapkin, A. A., et al. (2018). Synergistic contribution of the acidic metal oxide-metal couple and solvent environment in the selective hydrogenolysis of glycerol: a combined experimental and computational study using ReOx-Ir as the catalyst. ACS Catal. 9, 485–503. doi:10.1021/acscatal.8b03079
Wan, X., Zhang, Q., Zhu, M., Zhao, Y., Liu, Y., Zhou, C., et al. (2019). Interface synergy between IrOx and H-ZSM-5 in selective C-O hydrogenolysis of glycerol toward 1,3-propanediol. J. Catal. 375, 339–350. doi:10.1016/j.jcat.2019.06.025
Wang, J., Lei, N., Yang, C., Su, Y., Zhao, X., and Wang, A. (2016). Effect of promoters on the selective hydrogenolysis of glycerol over Pt/W-containing catalysts. Chin. J. Catal. 37, 1513–1519. doi:10.1016/S1872-2067(16)62479-8
Wang, J., Yang, M., and Wang, A. (2020). Selective hydrogenolysis of glycerol to 1,3-propanediol over Pt-W based catalysts. Chin. J. Catal. 41, 1311–1319. doi:10.1016/S1872-2067(20)63586-0
Wang, X. L., Zhou, J. J., Sun, Y. Q., and Xiu, Z. L. (2019). Bioconversion of raw glycerol from waste cooking-oil-based biodiesel production to 1,3-propanediol and lactate by a microbial consortium. Front. bioeng. biotech. 7, 14. doi:10.3389/fbioe.2019.00014
Wang, Y., Zhou, J., and Guo, X. (2015). Catalytic hydrogenolysis of glycerol to propanediols: a review. RSC Adv. 5, 74611–74628. doi:10.1039/C5RA11957J
Yang, C., Zhang, F., Lei, N., Yang, M., Liu, F., Miao, Z., et al. (2018). Understanding the promotional effect of Au on Pt/WO3 in hydrogenolysis of glycerol to 1,3-propanediol. Chin. J. Catal. 39, 1366–1372. doi:10.1016/S1872-2067(18)63103-1
Yang, M., Zhao, X., Ren, Y., Wang, J., Lei, N., Wang, A., et al. (2018). Pt/Nb-WOx for the chemoselective hydrogenolysis of glycerol to 1,3-propanediol: Nb dopant pacifying the over-reduction of WOx supports. Chin. J. Catal. 39, 1027–1037. doi:10.1016/S1872-2067(18)63074-8
Zhao, W. C., Ren, H. R., Zhang, X., Wang, Z., Zhao, Y. M., Liu, L., et al. (2019). Rapid determination of 1,3-propanediol in fermentation process based on a novel surface-enhanced Raman scattering biosensor. Spectrochim. Acta 211, 227–233. doi:10.1016/j.saa.2018.11.042
Zhao, X., Wang, J., Yang, M., Lei, N., Li, L., Hou, B., et al. (2017). Selective hydrogenolysis of glycerol to 1,3-propanediol: manipulating the frustrated Lewis pairs by introducing gold to Pt/WOx. ChemSusChem 10, 819–824. doi:10.1002/cssc.201601503
Zhou, W., Li, Y., Wang, X., Yao, D., Wang, Y., Huang, S., et al. (2020). Insight into the nature of Brönsted acidity of Pt-(WOx)n-H model catalysts in glycerol hydrogenolysis. J. Catal. 388, 154–163. doi:10.1016/j.jcat.2020.05.019
Zhou, W., Luo, J., Wang, Y., Liu, J., Zhao, Y., Wang, S., et al. (2019). WOx domain size, acid properties and mechanistic aspects of glycerol hydrogenolysis over Pt/WOx/ZrO2. Appl. Catal. B-Environ. 242, 410–421. doi:10.1016/j.apcatb.2018.10.006
Zhou, W., Zhao, Y., Wang, Y., Wang, S., and Ma, X. (2016). Glycerol hydrogenolysis to 1,3-propanediol on tungstate/zirconia-supported platinum: hydrogen spillover facilitated by Pt(1 1 1) formation. ChemCatChem 8, 3663–3671. doi:10.1002/cctc.201600981
Keywords: glycerol, hydrogenolysis, propanediol, minireview, mechanism
Citation: Chen J, Xia Q, Wang Y and Huang Y (2020) Progress in Production of 1, 3-propanediol From Selective Hydrogenolysis of Glycerol. Front. Chem. Eng. 2:604624. doi: 10.3389/fceng.2020.604624
Received: 10 September 2020; Accepted: 16 November 2020;
Published: 22 December 2020.
Edited by:
Florent Allais, AgroParisTech Institut des Sciences et Industries du Vivant et de L’environnement, FranceReviewed by:
Francesco Mauriello, Mediterranea University of Reggio Calabria, ItalyCopyright © 2020 Xia, Chen, Wang and Huang. This is an open-access article distributed under the terms of the Creative Commons Attribution License (CC BY). The use, distribution or reproduction in other forums is permitted, provided the original author(s) and the copyright owner(s) are credited and that the original publication in this journal is cited, in accordance with accepted academic practice. No use, distribution or reproduction is permitted which does not comply with these terms.
*Correspondence: Qineng Xia, eGlhcWluZW5nQG1haWwuemp4dS5lZHUuY24=; Yuandong Huang, aHVhbmd5ZEB1c3N0LmVkdS5jbg==
Disclaimer: All claims expressed in this article are solely those of the authors and do not necessarily represent those of their affiliated organizations, or those of the publisher, the editors and the reviewers. Any product that may be evaluated in this article or claim that may be made by its manufacturer is not guaranteed or endorsed by the publisher.
Research integrity at Frontiers
Learn more about the work of our research integrity team to safeguard the quality of each article we publish.